Abstract
Electroporation, an attractive process for delivering DNA and other molecules into target cells in vivo and in vitro is limited by the necessity of electrodes that need to be in contact with the subject or object to be electroporated. We have used magnetic fields, which do not require material contact with the subject, to temporarily permeabilize cells in guinea pig skin in vivo to enhance uptake and expression of GFP plasmid DNA. The results show for the first time that magnetic fields can trigger a process likely similar to electroporation. In designing the magnetic pulses, our most important criterion was a high rate of change of the magnetic field, based on the principle described by Michael Faraday which is expressed by the formula: E = -dB/dt, (E, electric field, B, magnetic field, t, time). Magnetic fields were generated by a flat electromagnet in a hand-held applicator positioned above the target tissue. The magnetic pulses had a peak magnetic flux density of 4 tesla; 50 pulses were applied in 5 sec. Biphasic magnetic pulses were twice as effective as monophasic pulses and about equally effective as traditional electroporation pulses . Advantages of magnetopermeabilization over electoporation include: No contact between applicator and subject (“contact-less”); no need for invasive, disposable, sterile electrodes (“needle-less”); no pain from needles and reduced overall pain; no known side effects; easier and faster to administer than electroporation; less expensive due to absence of disposables; and, importantly, greater tissue penetration of the magnetic field allowing treatment of anatomical areas inaccessible by electroporation.
Introduction
A critical step in DNA-based vaccination is the mode of DNA delivery into the target tissue and ultimately into appropriate target cells. A sufficient number of target cells must be transfected with an adequate amount of DNA, while remaining capable of producing and exporting a critical amount of proteins coded for by the vaccine DNA, in order to elicit the desired immune response. Among the various methods for in vivo DNA delivery, electroporation, also referred to as electropermeabilization (in this paper we will use both terms interchangeably), has been used extensively over many years in research laboratories as well as in medical and other applications,Citation1-Citation3 including the delivery of DNA vaccines.Citation4,Citation5 Electroporative delivery of DNA usually involves the injection of DNA solution into the target tissue by mechanical means, e.g., needle and syringe, followed by the insertion of needle array electrodes encompassing the injection site, and subsequent delivery of electrical pulses via these electrodes. Electrode configurations other than needle electrodes have also been used,Citation6 but their effectiveness tends to be lower, especially in larger mammals including humans, with the possible exception of microneedle arrays designed for electroporation of the skin.Citation7 The imparted electric field causes transient changes in the cell membrane that temporarily allows the DNA to pass through this barrier, which otherwise would exclude the DNA from the cell interior. In order to generate electric fields of sufficient strength for electroporation to occur, electrodes generally must be in physical contact with the tissue to be electroporated. Methods which do not use contact electrodes, including helium plasma and spark discharge,Citation8,Citation9 have only been shown to permeabilize skin cells, but not cells in underlying tissue, and their effectiveness in larger animals and humans has not been demonstrated. The use of invasive electrodes such as needle electrodes which are most effective for electroporating cells located in human skin as well as in a variety of underlying tissues has a number of disadvantages: the electrodes must be sterile and must be placed into the tissue uniformly and with sterile technique; insertion of the electrodes and subsequent delivery of electric pulses causes pain;Citation10 the size of the area and the depth and location of tissue that can be electroporated is quite limited; and side effects such as burns at spots of high current density may occur.
We wanted to overcome the inherent disadvantages of electrodes in producing electric fields by circumventing the use of electrodes altogether and instead achieve the induction of electric fields by employing changing magnetic fields. Since Faraday’s famous experiments on electromagnetism, it has been known that magnetic fields that change in their magnitude and/or direction induce electric fields according to the equation introduced by Maxwell:
(1)
E = -dB/dt
This vector equation states that an electric field E is generated as the magnetic field B changes with time t. Furthermore, an electric field generates current that is proportional to the magnitude of the electric field and inversely proportional to the electric resistance R of the substrate in which the current flows, in our case the resistance of the tissue in which the electric field is induced. Thereby, the changing magnetic fields generate currents in the tissue. Electropermeabilization and magnetopermeabilization are similar, with one of the differences being that the geometry of the changing magnetic field produces circular electric fields and eddy currents, as explained in the Kardos/Rabussay patent application 12/732,195 in 2010.Citation11
While the concept of using magnetic fields for electrode-less, and thus contact-less, electropermeabilization has been considered for some timeCitation6,Citation12 the practical realization of this concept has not been pursued until recently. Early calculations had shown that high magnetic field strengths in the order of several tesla (changed at a high rate) would be required in order to induce electric fields of sufficient strength to achieve effective electropermeabilization. Additional considerations as to how rapidly changing magnetic fields of the desired intensity, shape, focus and homogeneity can be produced at reasonable expense and without ponderous equipment were also discouraging.
In this paper we report evidence that magnetopermeabilization is indeed feasible, and that it can be achieved with relatively inexpensive and portable equipment. Our results demonstrate that gene expression levels reached with magnetopermeabilization are comparable with those achieved by traditional electroporation with needle electrode arrays. The immediate advantages of magnetopermeabilization, however, are clear in that no invasive, disposable, sterile needle electrodes are required and patient discomfort from embedded sharps and electric pulses is eliminated. Other advantages and potential advantages of this new method will be discussed in the following sections.
Results
As described in Materials and Methods, hairless guinea pigs received intradermal injections of GFP (Green Fluorescent Protein) plasmid DNA in a pattern of pre-marked sites along their backs on the left and right side of the spine (). Thirty to 60 sec after injection, magnetic pulses were delivered to the injection site. The number, length and frequency of the pulses had been established in a series of preliminary experiments (results not shown).
Figure 1. Animal No. MG-08 with 24 marked sites for DNA injection and subsequent pulse delivery, if indicated.
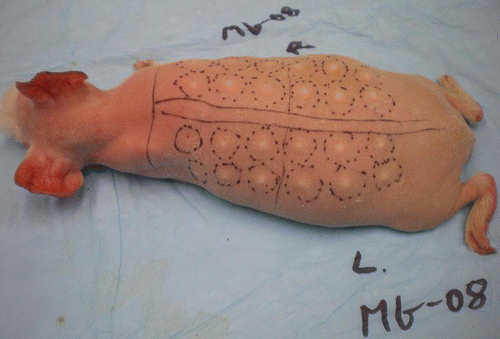
For the experiments reported in this paper, the magnetic pulses used were either monophasic or biphasic. show oscilloscope recordings representing the magnetic field strength (y- axis) over time (x- axis) during the magnetic pulses. There is a rapid rise of the magnetic field at the leading edge of the pulses, which appears as a nearly vertical line in . shows the leading edge of a pulse on an expanded time scale where a rise time of about 700 ns can be observed. It is during the rapid rise of the pulse that eddy-currents and rotating electric fields are induced to the greatest extent in the tissue. The greater the rate of change of the magnetic field, the greater the induction (see EquationEqn. 1).
Figure 2. Oscilloscope tracings of magnetic pulses applied in this study. X-axis, time; y-axis volts, representative of magnetic field strength and polarity. (A) Monophasic pulse, total pulse width about 150 µs. (B) Biphasic pulse, total pulse width about 300 µs.
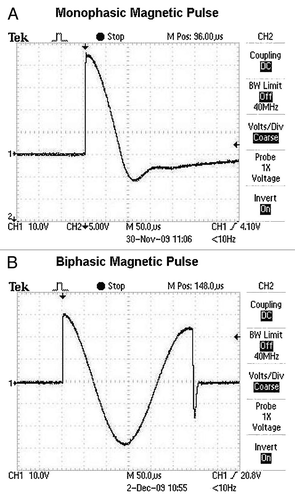
Figure 3. Oscilloscope tracing of the leading edge of a magnetic pulse applied in this study. The rise time is approximately 700 ns. x-axis, time; y-axis, volts, representative of magnetic field strength.
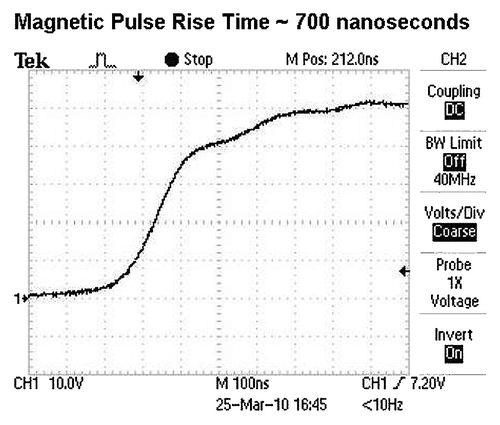
Both mono- and biphasic pulses were delivered to DNA injection sites at a rate of 10 pulses per second for five seconds, a total of 50 pulses. The rise time of the magnetic pulses was about 700 ns and the peak magnetic field strength was about 4 tesla, or 40,000 gauss. As listed in the manufacturer’s information, the MagPro R30 stimulator with the C-100 coil produces a maximal field strength of approximately 4 tesla during the steep rise of the pulse near the coil surface and within about one cm of the central axis. For comparison, 4 tesla is in the range of field strength associated with the strongest permanent magnets presently known, as well as the field strength generated in today’s large Magnetic Resonance Imaging (MRI) machines; however, both of those fields are static magnetic fields. In monophasic pulses only the magnetic field strength changes: it increases rapidly, reaches a sharp peak and then declines (). The decline of the monophasic pulse is much slower than its rise, but still occurs within the short time span of about 110 µs. In the case of biphasic pulses both the magnetic field strength and the direction of the field change, creating two instances per pulse where the rate of change of the magnetic field is very high and thus strong electric fields are induced ().
The effectiveness of magnetopermeabilization as a result of applying the described pulses is demonstrated by the data summarized in and . shows fluorescent images created by the expression of GFP after intradermal DNA injection and exposure of the DNA injection sites to the different types of pulses (monophasic or biphasic magnetic pulses, electropermeabilization pulses), or no pulses. Twelve injection sites are shown for each type of treatment. From visual inspection of the levels of GFP expression it can be seen that biphasic magnetic pulses and electropermeabilization pulses yield, on average, similar results. Monophasic magnetic pulses appear, on average, less effective than biphasic pulses and GFP expression in the absence of any applied electromagnetic pulses is clearly weakest. It is also apparent that exposure to biphasic and electropermeabilization pulses correlate with more consistent levels of GFP expression than levels obtained with monophasic or no pulses.
Figure 4. Fluorescent images of GFP expression in guinea pig skin. The four panels show fluorescent images of GFP expression in skin flaps (two flaps per panel) from guinea pigs exposed to different types of electromagnetic pulses (including Magnetic Monophasic, Biphasic and EP, Electroporation), or no pulses. As described more fully in Materials and Methods, guinea pigs were injected intradermally at pre-marked sites with GFP plasmid DNA, followed by delivery of mono- or biphasic magnetic pulses, electric pulses, or no pulses, as indicated at the top of each panel. After about 2.5 d animals were euthanized, skin flaps were removed and images were taken with an OV 100 Imaging System (Olympus). The mean fluorescence intensity of treatment sites labeled 1–12 for each of the four treatment groups shown in this figure correspond to four bars in .
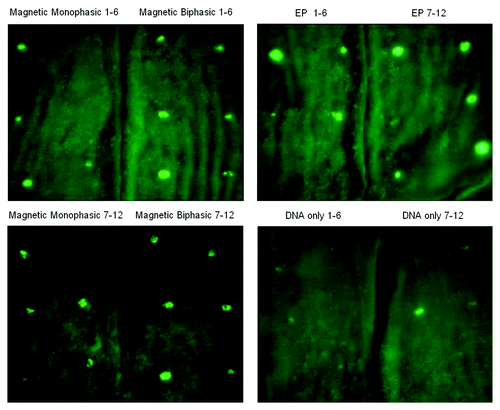
Figure 5. Quantitative Evaluation of the Fluorescent Images.The digitized images of the 12 sites of each group shown in were analyzed using Pixcavator 4.3 software by determining the area of fluorescent expression and the average intensity of fluorescence within that area. The units of the y-axis represent the product of the size of the fluorescent spot (mm2) multiplied by the average intensity (on a scale of 0–255). Each bar represents the mean value for the group indicated. The corresponding numeric values, from left to right, are 563, 943, 1004 and 175, respectively. All images were obtained with the same settings and all perimeters were determined with the same intensity threshold. The four groups were statistically compared with each other using Minitab 15 software and the Two-Sample T-test (see ).
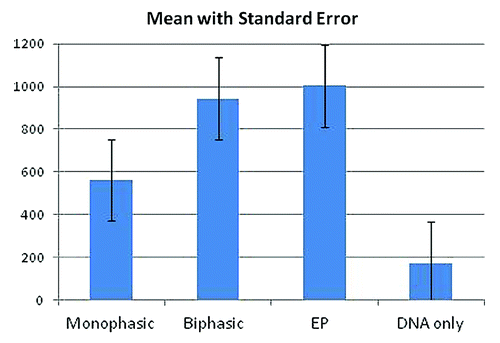
Table 1. Statistical evaluation of the differences between various treatment groups
Data of the quantitative evaluation of GFP expression are summarized in . In terms of the relative effectiveness of the four different types of experimental conditions applied, these data lead to the same conclusion as has been reached from visual inspection. Statistical analysis of the data shown in allow the conclusion that biphasic magnetic pulses and conventional electroporation pulses yield about equally high levels of GFP expression (no significant difference; p = 0.84). Monophasic magnetic pulses are about half as effective as biphasic magnetic or electroporation pulses (p < 0.05). The absence of any application of magnetic or electric pulses results in about 6-fold lower GFP levels compared with biphasic magnetic or electroporation pulses, and about 3-fold lower levels than obtained with monophasic magnetic pulses (p < 0.001 and p = 0.01, respectively).
Improvements in both experimental technique and quantitative analysis over the ones used in this study are possible and desirable. The scatter in the data presented in and is most likely due to two main factors: variability in the intradermal injection technique and difficulty in co-localizing the areas of highest magnetic field emanating from the center of the applicator with the site of DNA injection. We believe both can be improved by optimizing, and becoming increasingly familiar with, applicable experimental technique and by minor equipment modifications. Improved quantitative analysis of transgene expression may be achieved by using marker genes other than GFP, e.g., marker genes whose products can be determined quantitatively by enzymatic or immunological tests. Such tests may be more reliable in yielding linear responses to the quantity of transgene product than fluorescence measurements.
In conclusion, the data presented demonstrate that magnetic pulses of appropriate field strength, rate of change, frequency and number such as used in our experiments can achieve transfection results that equal results obtained by conventional electroporation with invasive needle electrodes. Definite and potential strengths and weaknesses of magneto- and electropermeabilization, respectively, will be discussed in the following section.
Discussion
This study has taken a novel approach to achieve temporary permeabilization of cell membranes. We have demonstrated for the first time that the application of fast-changing magnetic fields enhances the transport of plasmid DNA across cell membranes in vivo to a similar extent as does “classical” electroporation with electric fields. This opens the possibility of building on the knowledge and applications that started with the discovery of this remarkable phenomenonCitation13 and has since grown into an active segment of research and applications, including in the medical field.Citation14-Citation20 Magnetopermeabilization has the potential of becoming a simpler yet more broadly applicable method than electropermeabilization for reasons discussed below.
The potential use of magnetic fields for the induction of electric fields capable of electropermeabilizing cell membranes has been on the scientific horizon for some time, but the realization of that concept has been lacking. After several unsuccessful attempts, we eventually defined the magnetic pulse parameters and suitable equipment that enabled successful membrane permeabilization. The breakthrough occurred when we realized that working with shorter rise times and larger numbers of pulses was the more promising path to achieving membrane permeabilization than attempts by changing other pulse parameters and equipment specifications. At this time we do not know whether the permeabilization mechanism triggered by the magnetic field is strictly due to the induced electric field, i. e., analogous to the mechanism at work in classical electroporation, or whether the magnetic field’s direct interaction with electric charges on membrane molecules, DNA molecules and ions also contributes to the membrane permeabilization effect. As indicated by EquationEqn. 1 and the subsequent discussion in the Introduction, a changing magnetic field provides a magnetomotive force analogous to the electromotive force from a static electric field, both of which move ions and electric charges. The kinetic energy of the accelerated ions may even contribute to the disruption of the cell membrane, which may support the passage of large molecules through the membrane.
A comparison of magnetic and electric pulses used in our experiments described above shows that magnetic pulses were of much shorter duration and were delivered at higher frequency and in greater number than electric pulses, but both produced about the same effect on GFP expression levels. In fact, the cumulative effective pulse duration for biphasic magnetic pulses per treatment site was about 70 µs which is about 1,700-fold lower than the accumulated pulse duration of the electric pulses (120 ms). We suspect that the shortness of the magnetic pulses was compensated for by their larger number. This interpretation is in agreement with the observation mentioned earlier that biphasic magnetic pulses, each of which produces two strong electric pulses, are about twice as effective as monophasic pulses (). Moreover, we have observed that the level of GFP expression increases, within the range of 0 to 50 pulses, with the number of magnetic pulses (data not shown). It is also known from many optimization experiments in the electroporation area that pulse length and the number of pulses can compensate for each other within certain limits. We can however provide a quantitative measure for successful magnetically induced permeabilization, which is a measure of the dB/dt in the amount of 4 tesla/700 ns = 5.7 MT/s (megatesla per second), applied with 50 biphasic pulses over 5 sec. Optimization of pulse parameters is frequently performed for electroporation pulses since experience has shown that optimal parameters for different cell and tissue types can vary over a wide range. Optimization of magnetic pulse parameters is more difficult because, at present, our capability of manipulating pulse amplitude, duration and shape is quite limited. However, we believe that with further development of magnetic pulse generators it will become possible in the future to also optimize magnetic pulse parameters for specific applications.
Advantages of magnetopermeabilization over electropermeabilization are several-fold: (1) No invasive, disposable, sterile electrodes are needed that have to be carefully placed and removed under aseptic conditions. This eliminates the potential risk of infection, which may become especially important when large scale vaccinations are to be performed. (2) No pain or anxiety due to electrode needle insertion has to be tolerated. This may be of particular advantage in the case of DNA vaccination of children, both in developed and even more importantly in developing countries. (3) Reduced pain from magnetic pulses compared with electric pulses, since there are no embedded sharps in the tissue during pulse-induced contractions. (4) Fewer potential side effects due to high local current density, e.g., burns at needle insertion sites. (5) Absence of high-voltage sources or other potential spark-generating equipment which may trigger explosions of flammable gases, including certain anesthetic gases in a hospital environment. (6) Larger areas and greater volumes of tissue can be permeabilized simply by “sweeping” the applicator over the target area at a certain rate to deliver the desired number of pulses to every point of the area or volume to be treated. (7) Greater depth penetration of the magnetic field; the magnetic field strength from this coil drops off by about 50% with every 1 cm distance away from the surface of the contactless applicator. Therefore, starting with about 4 tesla and 5.7 MT/s at the applicator surface, which is what we have demonstrated in skin, the present device would provide half of these values at 1 cm depth or a quarter at 2 cm depth. With improved equipment capable of greater dB/dt, it would be possible to achieve similar permeabilization results as shown in this paper at correspondingly greater tissue depths.
We envision that magnetopermeabilization will become an attractive choice in areas where electropermeabilization has already proven to be very useful. Such areas include DNA vaccines, gene therapy, electrochemotherapy of cancer and dermal/cosmetic applications.
For DNA vaccines magnetopermeabilization has the potential to become the preferable method of vaccine delivery. In addition to the advantages over electropermeabilization enumerated above, magnetopermeabilization is easier to administer and faster to perform since no preparatory or clean-up steps are necessary. The vaccination procedure would only involve the injection of the vaccine (which in the future may be done in a contactless manner by jet injectorsCitation21), followed by placing the magnetic applicator over the injection site for 5 sec. The jet injector may be inserted through the central hole of the coil.Citation11 The vaccine could be administered with the same magnetopermeabilization instrument either intra- or subcutaneously, intramuscularly, or into other tissues at various depths. Magnetopermeabilization is also less expensive since no disposables are necessary. In this context we would like to mention that recently another contactless delivery device has been reported that may be used to deliver DNA vaccines.Citation9 That device consists of a high-voltage spark generator producing a spark that travels from an electrode positioned several mm above the targeted area into the skin. The application of multiple sparks likely causes an identical or similar effect as classical electropermeabilization and has been shown to enhance GFP expression in guinea pig skin. Potential disadvantages of this method include substantial variability in the intensity of the sparks, only shallow penetration of the electric field into the skin and coverage of only a small area of skin. There is also the risk of triggering an explosion with flammable gasses. In light of these considerations and the fact that skin structure varies substantially depending on age, race, climate and other conditions, it remains to be seen whether consistent vaccination results can be obtained with the spark method.
The same advantages as discussed for DNA vaccine delivery by magnetopermeabilization apply to the area of gene therapy, with the additional advantage to treat anatomical locations not amenable to electropermeabilization, but accessible by magnetopermeabilization due to the superior depth of penetration of magnetic fields into and through tissues. One example where magnetopermeabilization could be useful for gene therapy is for the treatment of diabetesCitation22 by the delivery of a plasmid encoding insulin using a contactless magnetic applicator, to create a “biological insulin pump” in skin or muscle, replacing the need for a mechanical insulin pump. Another example is transcranial telepermeabilization of malignant brain cells. Transcranial magnetic stimulation has been already approved for applications such as treating depression and used for stimulating cortical motor centers.Citation23 The implication of our results is that brain tissue permeabilization may be achieved in a completely noninvasive contactless manner.
Electro-chemotherapy (ECT) is yet another area where the tissue-penetrating ability of magnetic fields could improve treatment capabilities. This type of treatment is now used routinely for the treatment of a limited number of tumor types.Citation14,Citation15,Citation17 It consists of intratumoral injection of an anti-cancer drug followed by electropermeabilization with invasive electrode arrays to permeabilize cancer cell membranes and thus enhance uptake and efficacy of the anti-cancer drug. This method is presently limited by the disadvantages inherent in electrode-dependent electropermeabilization but could become more widely applicable with magnetopermeabilization.
Now that magnetopermeabilization has become feasible in laboratory and potentially in clinical settings, a wide field for future studies has opened up. In addition to studies in the areas mentioned above, further studies are needed to expand our knowledge of the strengths and limitations of this method and the underlying mechanism of magnetopermeabilization. One area that will be essential before clinical applications can be initiated is to determine the effect of the magnetic fields to be applied on various healthy tissues. Especially for applications where deeper-seated tissues are targeted the effect of magnetopermeabilization on overlying tissues that are exposed to the highest magnetic field strength needs to be studied.
Materials and Methods
The study protocol was developed at MagneGene, Inc. and approved by the Institutional Animal Care and Use Committee of BioQuant, San Diego (Protocol number 09–079), a provider of preclinical research facilities. The study animals were also housed, and the in vivo experiments performed, at BioQuant.
Eight hairless female guinea pigs approximately four months old and weighing between 428 g and 574 g were purchased from Charles River Laboratories (Lot No. 24788) and participated in the study. We chose guinea pigs because their skin is an accepted animal model for human skin. Five animals were used to develop the magnetopermeabilization technique and magnetic pulse parameters. The remaining animals were employed to compare the efficacy of monophasic and biphasic magnetic pulses, and magnetopermeabilization against electropermeabilization, respectively. The back of each animal was segregated into 12 locations on the left and 12 locations on the right side of the spine (). Forty-eight locations on three animals were allocated to four groups: after anesthetizing animals by inhalation of isoflurane, all groups were injected intradermally at each location with 20 µl (1 mg/ml) pgWiz-GFP plasmid DNA in distilled water (Aldevron) using a 0.3 mL 29GA × 0.5 in U-100 insulin syringe (Becton-Dickinson, BD#309301). Group A received DNA only; group B locations were also subjected to electroporation; Group C was subjected to monophasic magnetic pulses; and Group D received biphasic magnetic pulses. Magnetic or electric pulses were delivered 30–60 sec after DNA injection to injection sites as indicated. Magnetic pulses were applied using a MagPro R30 Magnetic Stimulator (Alpine Biomed), 230 V, with either a C-100 (flat) or MMC-140–2 (concave) magnetic coil. The MagPro R30 generates the pulses of electric current that power the electromagnets (“coils”) located in the paddle-shaped, hand-held applicators (). The applicators have a central hole surrounded by the conductive coils that generate the magnetic field when energized by electric current pulses originating from the MagPro power supply. The main magnetic field emanates from the hole in a direction perpendicular to the plane of the “paddle.” Therefore, when delivering magnetic pulses, the hole of the applicator is placed in alignment with the targeted DNA injection site. The system generates a magnetic field strength of approximately four tesla with a field rise time of approximately 700 ns. Mono- or biphasic magnetic pulses () were applied for 5 sec at each designated site at a rate of 10 pulses/s (50 pulses per site). The magnetic pulses were recorded on a TDS 210 digital storage oscilloscope (Tektronics). Electric pulses (two pulses, 30V, 60 ms each, 1 Hz) for electroporation were delivered by a BTX T820 ElectroSquare Porator (Genetronics) via a 4-needdle electrode array inserted into the skin (electrode needles of 26G, 10 mm length, arranged at the corners of a rectangle of 2.5 × 4.3 mm). Muscle twitches were observed both with magnetic and electric pulses. Approximately 2.5 d after DNA injection and pulsing (where applicable), animals were euthanized by i.v. injection of Phenobarbital and skin flaps containing six injection sites each were harvested and placed on ice. GFP expression was visually examined as well as quantitatively analyzed using an OV100 Imaging System (Olympus) together with Pixcavator 4.3 (Intelligent Perception), taking into account the area and intensity of the fluorescence of each injection site. Minitab 15 statistical software (Minitab, Inc.) was used for statistical analysis of the fluorescence data and the calculation of p values by Two-Sample T-Test.
Acknowledgment
We are grateful for the assistance of Mr. Daniel A. Brown in the animal studies and for analyzing GFP images using Pixcavator software, as well as for providing his expertise in working with large magnetic fields on magnetic resonance imaging (MRI) equipment.
Disclosure
The authors of this paper are also the inventors on US Patent Application 12/732,195 (2010), and were both officers of MagneGene, Inc.
References
- Saade F, Petrovsky N. Technologies for enhanced efficacy of DNA vaccines. Expert Rev Vaccines 2012; 11:189 - 209; http://dx.doi.org/10.1586/erv.11.188; PMID: 22309668
- Kaneda Y. Update on non-viral delivery methods for cancer therapy: possibilities of a drug delivery system with anticancer activities beyond delivery as a new therapeutic tool. Expert Opin Drug Deliv 2010; 7:1079 - 93; http://dx.doi.org/10.1517/17425247.2010.510511; PMID: 20716020
- Li S, ed. Electroporation Protocols: Preclinical and Clinical Gene Medicine. From Methods in Molecular Biology, Vol 423; Humana Press, New York, 2008.
- Widera G, Austin M, Rabussay D, Goldbeck C, Barnett SW, Chen M, et al. Increased DNA vaccine delivery and immunogenicity by electroporation in vivo. J Immunol 2000; 164:4635 - 40; PMID: 10779767
- Weiner DB. DNA vaccines: crossing a line in the sand. Introduction to special issue. Vaccine 2008; 26:5073 - 4; http://dx.doi.org/10.1016/j.vaccine.2008.07.024; PMID: 18652867
- Rabussay D. Applicator and electrode design for in vivo DNA delivery by electroporation. In: Li S, ed. Methods in molecular biology, New York:Humana Press, 2008:35-59.
- Guo S, Donate A, Basu G, Lundberg C, Heller L, Heller R. Electro-gene transfer to skin using a noninvasive multielectrode array. J Control Release 2011; 151:256 - 62; http://dx.doi.org/10.1016/j.jconrel.2011.01.014; PMID: 21262290
- Connolly RJ, Rey JI, Lambert VM, Wegerif G, Jaroszeski MJ, Ugen KE. Enhancement of antigen specific humoral immune responses after delivery of a DNA plasmid based vaccine through a contact-independent helium plasma. Vaccine 2011; 29:6781 - 4; http://dx.doi.org/10.1016/j.vaccine.2010.12.054; PMID: 21195804
- Broderick KE, Kardos T, McCoy JR, Fons MP, Kemmerrer S, Sardesai NY. Piezoelectric permeabilization of mammalian dermal tissue for in vivo DNA delivery leads to enhanced protein expression and increased immunogenicity. Hum Vaccin 2011; 7:Suppl 22 - 8; http://dx.doi.org/10.4161/hv.7.0.14559; PMID: 21263230
- Wallace M, Evans B, Woods S, Mogg R, Zhang L, Finnefrock AC, et al. Tolerability of two sequential electroporation treatments using MedPulser DNA delivery system (DDS) in healthy adults. Mol Ther 2009; 17:922 - 8; http://dx.doi.org/10.1038/mt.2009.27; PMID: 19277016
- Kardos TJ, Rabussay DP. Method of contactless magnetic electroporation. U.S. patent application No. 12/732,195. 2010, pending.
- Hofmann GA. Electroporetic gene and drug therapy. US patent No. 6,132,419. (2000).
- Neumann E, Schaefer-Ridder M, Wang Y, Hofschneider PH. Gene transfer into mouse lyoma cells by electroporation in high electric fields. EMBO J 1982; 1:841 - 5; PMID: 6329708
- Breton M, Mir LM. Microsecond and nanosecond electric pulses in cancer treatments. Bioelectromagnetics 2011; In press PMID: 21812011
- Miklavcic D, Mir LM, Vernier PT. Electroporation-based technologies and treatments. J Membr Biol 2010; 236:1 - 2; http://dx.doi.org/10.1007/s00232-010-9287-9; PMID: 20658230
- Li S. Delivery of DNA into tumors. Methods Mol Biol 2008; 423:311 - 8; http://dx.doi.org/10.1007/978-1-59745-194-9_23; PMID: 18370209
- Gehl J. Electroporation for drug and gene delivery in the clinic: doctors go electric. Methods Mol Biol 2008; 423:351 - 9; http://dx.doi.org/10.1007/978-1-59745-194-9_27; PMID: 18370213
- Andre FM, Mir LM. Nucleic acids electrotransfer in vivo: mechanisms and practical aspects. Curr Gene Ther 2010; 10:267 - 80; http://dx.doi.org/10.2174/156652310791823380; PMID: 20557285
- Daud AI, DeConti RC, Andrews S, Urbas P, Riker AI, Sondak VK, et al. Phase I trial of interleukin-12 plasmid electroporation in patients with metastatic melanoma. J Clin Oncol 2008; 26:5896 - 903; PMID: 19029422
- Muthumani K, Lambert VM, Kawalekar O, Heller R, Kim JJ, Weiner DB, et al. Anti-cancer activity of the HIV accessory molecule viral protein R (Vpr): Delivery as a DNA expression plasmid or biologically active peptides. Vaccine 2010; 28:2005 - 10; http://dx.doi.org/10.1016/j.vaccine.2009.10.060; PMID: 20188256
- Babiuk S, Baca-Estrada ME, Foldvari M, Baizer L, Stout R, Storms M, et al. Needle-free topical electroporation improves gene expression from plasmids administered in porcine skin. Mol Ther 2003; 8:992 - 8; http://dx.doi.org/10.1016/j.ymthe.2003.09.008; PMID: 14664802
- Prud’homme GJ, Draghia-Akli R, Wang Q. Plasmid-based gene therapy of diabetes mellitus. Gene Ther 2007; 14:553 - 64; http://dx.doi.org/10.1038/sj.gt.3302907; PMID: 17215847
- Ueno S. Studies on magnetism and bioelectromagnetics for 45 years: from magnetic analog memory to human brain stimulation and imaging. Bioelectromagnetics 2012; 33:3 - 22; http://dx.doi.org/10.1002/bem.20714; PMID: 22012916