Abstract
Analytical methods based on light microscopy, 90° light-scattering and surface plasmon resonance (SPR) allowed the characterization of aggregation that can occur when antibodies are mixed with human plasma. Light microscopy showed that aggregates formed when human plasma was mixed with 5% dextrose solutions of Herceptin® (trastuzumab) or Avastin® (bevacizumab) but not Remicade® (infliximab). The aggregates in the plasma-Herceptin®-5% dextrose solution were globular, size range 0.5–9 μm, with a mean diameter of 4 μm. The aggregates in the plasma-Avastin®-5% dextrose samples had a mean size of 2 μm. No aggregation was observed when 0.9% NaCl solutions of Herceptin®, Avastin® and Remicade® were mixed with human plasma. 90° light-scattering measurements showed that aggregates were still present 2.5 h after mixing Herceptin® or Avastin® with 5% dextrose-plasma solution. A SPR method was utilized to qualitatively describe the extent of interactions of surface-bound antibodies with undiluted human serum. Increased binding was observed in the case of Erbitux® (cetuximab), whereas no binding was measured for Humira® (adalimumab). The binding of sera components to 13 monoclonal antibodies was measured and correlated with known serum binding properties of the antibodies. The data presented in this paper provide analytical methods to study the intrinsic and buffer-dependent aggregation tendencies of therapeutic proteins when mixed with human plasma and serum.
Introduction
With numerous monoclonal antibodies (mAbs) already on the market and many more in the development, the progress in understanding of the factors that affect their safety and efficacy becomes increasingly important. In particular, the response of the immune system to these molecules gains increasing attention. The monitoring of the formation of anti-drug antibodies is an important aspect of clinical development due to the potential loss of efficacy,Citation1,Citation2 as well as potential cross-reactivity with endogenous proteins.Citation3,Citation4
Protein aggregation, one possible origin for the immunogenicity of biopharmaceuticals, can be potentially minimized at the design stage of the protein primary sequenceCitation5,Citation6 and by process and formulation design aimed at removal of protein aggregates.Citation7-Citation10 While protein behavior in formulation buffers has been extensively studied in terms of self-association,Citation9-Citation14 viscosityCitation15-Citation17 and chemical stability,Citation18,Citation19 the direct observation of the interactions with human plasma or sera has seldom been attempted.Citation20,Citation21
Incompatibility of dilution solutions with biopharmaceuticals is often highlighted in documents. For example, the prescribing information for Herceptin® (trastuzumab), Avastin® (bevacizumab) and Remicade® (infliximab) states that 0.9% NaCl should be used, and the use of 5% dextrose is prohibited; no justification is provided.Citation22 In contrast, for Neupogen® (recombinant methionyl human granulocyte colony-stimulating factor, r-metHuG-CSF), the use of 0.9% NaCl is prohibited because the “product may precipitate” and 5% dextrose solution should be used.Citation23
The instruction not to use a particular solution with antibodies (e.g., 5% dextrose for Herceptin®) is not explained in prescribing documents, and to our knowledge, the reasons for such interdictions have not been described in the literature. For example, the possibility that micron-sized aggregates might form in the patient’s bloodstream after application of the biopharmaceutical drug in the presence of incompatible diluents and that these aggregates may be responsible for side effects such as possible blocking of blood capillaries has not been ruled out or even addressed, to our knowledge.
Here, we present analytical methods to study the interaction of biopharmaceuticals with human plasma and human serum. Light microscopy and 90° light-scatter methods were used to investigate the plasma aggregation properties of Herceptin®, Avastin® and Remicade® diluted in 5% dextrose or in 0.9% NaCl. A surface plasmon resonance method is presented that allowed the qualitative characterization of the binding of human plasma components to different therapeutic antibodies.
Results
Analytical methods were adapted to study the aggregation phenomena that may occur when mAbs are mixed with human plasma. The formation of aggregates at the interface when human plasma was mixed with Herceptin® and Avastin® solutions in 5% dextrose is shown in , respectively. No aggregation occurred in the mixing region between plasma and Remicade® in 5% dextrose, . Plasma from three human volunteers showed the same aggregation properties when mixed with Herceptin® and Avastin® in 5% dextrose solutions (data not shown). No aggregation was observed when 0.9% NaCl solutions of Herceptin®, Avastin® and Remicade® were mixed with human plasma (); these results are in agreement with the recommendation by the manufacturer to use 0.9% NaCl for the infusion or dilution of the antibody. For the 5% dextrose solution of Herceptin®, the mixing region with plasma was compact (; ) and contained many globular aggregates in the size range of 0.5–9 μm, with a mean diameter of 4 μm (). In the case of mixing human plasma with 5% dextrose-Avastin®, the aggregation zone was more diffuse () and the aggregates were smaller (), in the range of 0.5–6 μm with a mean size of about 2 μm (). The shape of the aggregates at the Avastin®-plasma mixing zone was more heterogeneous, containing punctuate and elongated structures (). In , the numbers of particles per 6.25 nanoliters were 577 and 581 for Herceptin® and Avastin®, respectively. This indicates that the number of particles having a diameter between 0.5–9 μm is about 108 particles/ml.
Figure 1. Light microscopy pictures of human plasma mixed with 5% dextrose solutions of Herceptin® (A), Avastin® (B) and Remicade® (C). (E–G) correspond to human plasma mixed with 0.9% NaCl solutions of Herceptin®, Avastin® and Remicade®, respectively. The protein concentrations in the antibody solutions prior to the mixing with human plasma were: 1.06 mg/ml Herceptin®, 1.33 mg/ml Avastin® and 1.23 mg/ml Remicade®. No aggregation occurred when human plasma was mixed with 5% dextrose and 0.9% NaCl, (D and H) respectively. The distance between two thick lines is 250 μm (the smallest square is 250 μm × 250 μm).
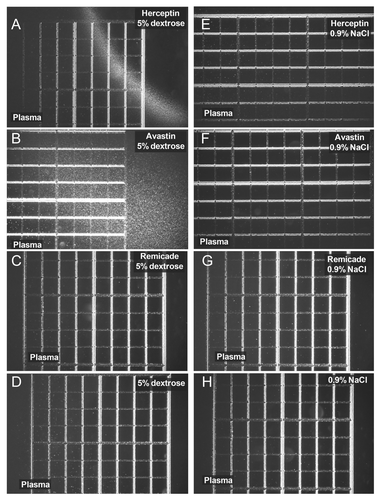
Figure 2. Light microscopy pictures and particle distributions of the aggregates observed at the contact region between Herceptin® diluted in 5% dextrose and human plasma (A–C) and between Avastin® diluted in 5% dextrose and human plasma (D–F). Herceptin® and Avastin® stock solutions (see Material and Methods) were diluted with 5% dextrose to a final protein concentration of 1.1 mg/ml and 1.3 mg/ml, respectively. The particle distributions were analyzed as described in Materials and Methods: 577 and 581 particles were counted in for the histograms in (C and F), respectively. The distance between two lines in (A and D) is 250 μm. The sizes of (B and E) correspond to 250 μm × 250 μm.
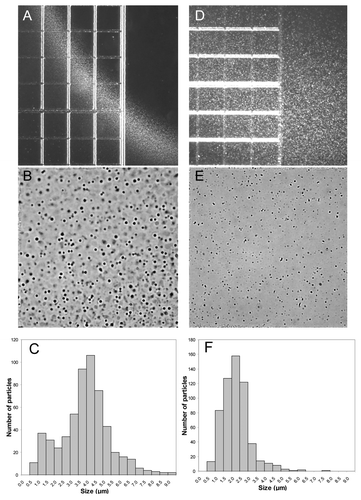
The extent of aggregate formation in the case of mixing human plasma with 5% dextrose solutions of Herceptin® or Avastin® was investigated by 90° light-scatter spectroscopy (). In these experiments, 3 ml of 5% dextrose were mixed with 10 μl of human plasma, and the 90° light-scatter was measured. To the 3 ml of dextrose with 10 μl plasma were added 20 μl of Herceptin® or Avastin® or 40 μl of Remicade® stock solutions. The scatter for the Herceptin® sample (after subtraction of the plasma scatter) is stronger than the scatter of the Avastin®-plasma sample (). A small scatter was observed for the Remicade® sample (). These results are in agreement with the microscopy data ( and ). For all three antibodies, no strong aggregation was measured in 0.9% NaCl (). Kinetic experiments at 25°C showed that the 90° light-scatter of the Herceptin® and Avastin® samples mixed with plasma in 5% dextrose decreased in time over 2.5 h (). The reduction in scatter had a fast phase (within the first 10 min) followed by a slow phase. This reduction in 90° light-scatter within the first 10 min is likely to be due to the reduction in size of the aggregates formed in plasma after mixing with Herceptin® in 5% dextrose, as observed by light microscopy ().
Figure 3. 90° light-scattering spectra of Herceptin® (red), Avastin® (blue) and Remicade® (green) mixed with human plasma in 5% dextrose (A) or 0.9% NaCl in water (B). Ten microliters of human plasma were mixed with 3 ml of 5% dextrose (A) or 0.9% NaCl solutions (B). After recording the 90° light-scattering spectra of the human plasma dextrose or human plasma NaCl mixtures, 20 μl of Herceptin® or Avastin® or 40 μl of Remicade® antibody stock solutions were added in the cuvettes. The final antibody concentrations in the cuvettes were 0.14 mg/ml, 0.17 mg/ml and 0.13 mg/ml for Herceptin®, Avastin® and Remicade®, respectively. The 90° light-scattering spectra were recorded within 2 min after mixing the antibodies with 5% dextrose-plasma or 0.9% NaCl-plasma. The scatter spectra of the human plasma were subtracted from the scatter spectra of plasma with antibodies.
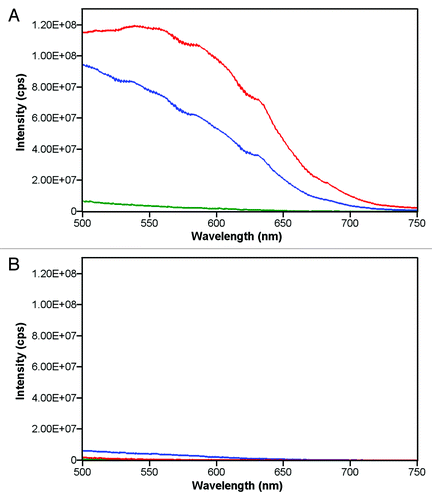
Figure 4. Changes in time in the 90° light-scattering at 500 nm of solutions of 5% dextrose of Herceptin® (red) and Avastin® (blue) mixed with human plasma (A). Ten microliters of human plasma were mixed with 3 ml of 5% dextrose. After recording the 90° light-scattering at 500 nm of the plasma-dextrose mixture, 20 μl of Herceptin® or Avastin® or 40 μl of Remicade® antibody stock solutions were added in the cuvettes. The final antibody concentrations in the cuvettes were 0.14 mg/ml for Herceptin® and 0.17 mg/ml for Avastin®. The 90° light-scatter at 500 nm of human plasma alone was subtracted from the 90° light-scatter of Herceptin® (red) and Avastin® (blue) plasma mixtures. Light microscopy pictures of human plasma mixed with 5% dextrose solutions of Herceptin® (1.06 mg/ml) were taken 1 min (B) and 7 min (C) after mixing.
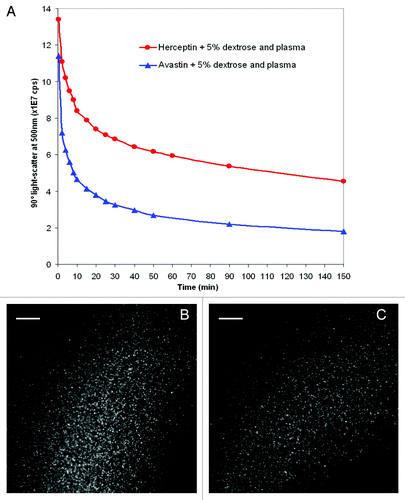
To qualitatively detect binding of human sera to therapeutic antibodies, we developed a high throughput (96-well plate auto-sampling) surface plasmon resonance (SPR) method. In a first step, therapeutic mAbs were chemically conjugated to the activated polysaccharide surface of the chip. To simulate the multivalent nature of the binding by antibodies, the reaction was performed until most of available sites were occupied to assure close proximity between the antibody molecules. No attempt was made to analyze the association and dissociation rates due to the unknown nature and inherent heterogeneity of the binding moieties in serum, as well as possible steric hindrance effects. At such high concentration of surface-attached antibody, addition of undiluted human serum resulted in a fast saturation of the binding of serum components to the chip ().
Figure 5. SPR measurements of mAb A4 (solid line) and mAb A8 (dashed line). 50 sec after the injection of mAbs, 10 μl of human serum were injected. The injected serum flowed over the activated chip surface for 2 min at 0.05 ml/min. After the running buffer replaced the injected volume, the residual amount of serum component that bound to the surface was observed between 160 and 250 sec. Two regeneration steps were then performed by injecting 50 mM sodium hydroxide (2 μl each). The relative amount of serum components bound to the chip is marked with vertical bars at ~200 sec.
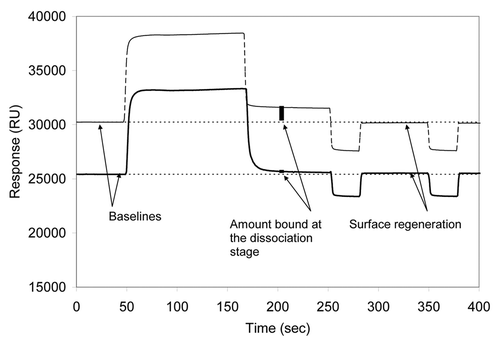
After the injected sample bolus left the flow cell, the remaining amount of bound protein was measured (at ~200 sec; ). Two consecutive regeneration injections were performed to strip off the bound material and re-equilibrate the surface by restoring the flow of the running buffer (between 250 and 400 sec; ). Examination of the responses from sera taken from different individuals suggested that the variability between the measurements of a sample from the same individual, as well as differences between different individuals, was about 10–20% (data not shown). For an experimental mAb A8 immobilized to the chip at values above 10,000 RU (13,500–19,400 RUs), the interaction with serum components from human, rhesus monkey and mouse wild-type and knockout were distinctly different with a relatively low variability suggesting multimeric nature of the interaction with human serum components ().
Figure 6. Biacore® responses using mAb A8 at various levels of immobilization on the chip. The sera from four different sources were used: human (white), rhesus monkey (light gray), mouse-wild type (dark gray) and mouse-knockout (black). The gene for the antigen targeted by the mAb detected was deleted in the knockout mice. The relative response increases (RU units) due to the immobilization of the antibody on the chip (before the exposure to serum) were 19400, 15400, 13500 and 7100 for groups 1, 2, 3 and 4, respectively.
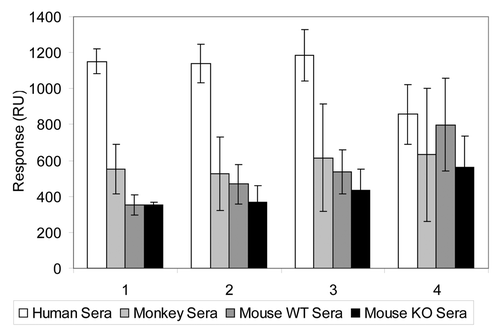
Thirteen mAbs were immobilized and the extent of binding to the sera from about 30 individuals was sequentially recorded (). The first group of white bars () represents a series of Merck experimental mAbs (IgG1 and IgG2) that bind to the same target. Baseline binding for a human IgG in this format is around 200–300 RUs, thus inert mAbs were used as a control surface to identify binding mAbs (> 700 RUs). While mAbs A1 to A5 showed baseline signals, mAbs A7 and A8, and perhaps A6, showed elevated responses, indicative of binding to serum components (). Interestingly, mAbs A6, A7 and A8 bear sequence similarity of their complementarity-determining-regions that differentiates them from the mAbs A1–A5.
Figure 7. Biacore® responses from various monoclonal antibodies. The dashed line denotes an arbitrary level differentiating “binders” from “non-binders.” First group of bars (white): mAbs A1–A8 are targeted against common antigen, and mAbs A6–A8 bear high sequence similarity of their complementarity-determining regions. Second group of bars (gray): monoclonal antibodies B1 and B2 are directed toward common antigen; mAb B2 was shown to interact with human blood components by independent methods. Third group of bars (black): C1 and C3 correspond to adalimumab and cetuximab, respectively; C2 is an experimental monoclonal antibody that was not associated with any adverse reactions in primate toxicity studies.
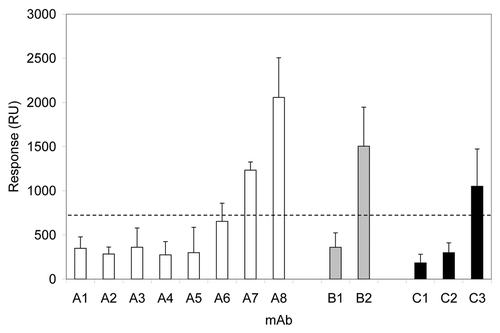
The second group of bars (gray) in shows that mAb B2 interacts with human sera components: antibodies B1 and B2 were designed to bind to the same target. Since mAb B2 was known to bind strongly and specifically to fibrinogen (data not shown), the results in can be explained by fibrinogen binding to the B2 antibody.
The third group of bars (black) in consists of antibodies that bind to other targets: C1 is Humira® (adalimumab), C2 is a Merck in-house antibody and C3 is Erbitux® (cetuximab). Erbitux® gave a strong SPR response () result that is in agreement with the known properties of Erbitux® to bind human sera components and to cause infusion hypersensitivity reactions.Citation25 Humira®, C1, had a negligible SPR response, in agreement with the fact that the antibody is known to have a low incidence of infusion hypersensitivity. C2 antibody showed no interaction with human sera () and had no side effects in animal toxicity studies.
Discussion
In a previous study, it was shown that when Herceptin® was mixed with 5% dextrose, antibody aggregates formed immediately,Citation14 a phenomenon that may be related to the restriction to use only 0.9% NaCl with Herceptin®. The present study showed that augmented protein aggregation occurred when human plasma was mixed with Herceptin® diluted in 5% dextrose. Whereas the aggregates of Herceptin® in 5% dextrose were difficult to detect and the solution was clear by eye,Citation12 the aggregates in the plasma and Herceptin®-5% dextrose solution were very large and the solution was turbid. Avastin® diluted in 5% dextrose also aggregated when mixed with human plasma; no aggregation was observed for Remicade® (). Plasma from three volunteers showed the same aggregation behavior when mixed with Herceptin® in 5% dextrose, indicating that the aggregation under these conditions is a general phenomenon. In agreement with the recommendations of the manufacturers to use saline solutions for infusion,Citation22 no aggregation was observed when 0.9% NaCl solutions of Herceptin®, Avastin® and Remicade® were mixed with human plasma.
Globular, dense aggregates between 0.5 μm and 9 μm in diameter were formed in the mixing region of Herceptin® in 5% dextrose solution and human plasma, with a mean size of 4 μm (). The 5% dextrose Avastin® plasma aggregation zone contained smaller, heterogeneous aggregates, in the size range of 0.5–6 μm with mean size of about 2 μm (). The number of particles in 6.25 nanoliters were 577 and 581 for Herceptin® and Avastin®, respectively; this corresponds to about 108 particles/ml having diameters between 0.5 μm and 9 μm and to about 1,600,000 particles/ml of 9 μm diameter in the case of human plasma mixed with Herceptin® in 5% dextrose. Although this size of 9 μm is just below the 10 μm USP limit of tolerance,Citation24 the number of 9 μm particles is very high and largely exceeds 6000, the number of sub-visible particles in parental formulations of 10 μm and larger allowed by the USP General Chapter < 788 > Particulate Matter in Injections.Citation24 The potential for side effects of these particles is not negligible. 90° light-scattering experiments showed that the aggregates did not solubilize quickly, still being present 2.5 h after mixing Herceptin® or Avastin® with 5% dextrose-plasma solution ().
A SPR high throughput method was developed to study the binding of human serum components to a surface covered with immobilized mAbs ().Citation25-Citation27 After saturating the binding of the antibodies to the chip surface, undiluted human serum was flushed and the amount of bound proteins to the mAb layer was measured. Thirteen antibodies were screened by the SPR assay for their binding to human sera. The SPR method could distinguish between antibodies known to interact or not interact with human serum components. Thus, in the SPR measurements Humira® (adalimumab) showed no significant binding of serum components, in agreement with the low incidence of infusion hypersensitivity associated with administration of the antibody.Citation28 On the other hand, Erbitux® (cetuximab) had a strong SPR response (), which is in agreement with the known properties of Erbitux® to bind to pre-existing antibodies in human sera and to cause infusion hypersensitivity reactions.Citation29
The light microscopy, 90° light-scatter, and the biosensor methods could be used as screening tools to study the interaction of therapeutic proteins with human plasma and sera. The methods are robust and permit a fast test using multiple conditions and numerous samples. Light microscopy does not require dilution of the antibody, the plasma or serum samples, and thus provides information under conditions similar to the in vivo use. The different SPR interactions with human serum observed with the 13 mAbs () show that the SPR screening assay may also be a useful tool in the evaluation and selection of therapeutic protein candidates and of their optimal formulations.
This paper also documents that depending on the formulation a therapeutic antibody may or may not aggregate in the presence of human plasma. This observation is new and important for the field of formulation research in the biopharmaceutical industry. At present, major efforts are being made in the development of chemically and physically stable formulations of biopharmaceuticals, the stability being assessed in vitro, in the application container (e.g., vials or syringes). Our data show that formulation optimization and development studies should also include the compatibility with human plasma, sera and blood.
Use of incompatible diluents carries a definitive risk of adverse events due to micron-size aggregates, such as blocking of blood capillaries and increased immunogenicity. It is long known that foreign particles entering the blood stream during intravenous (i.v.) administration may act as emboli and form granulomas or thrombi.Citation30,Citation31 In the case of Herceptin®, the use of 5% dextrose in i.v. solutions is forbidden in both the full prescribing information and the package inserts. Dextrose is also excluded as an i.v. diluent in the full prescription for Avastin®;Citation22 however, we found package inserts for Avastin® in the German, French and Dutch languages where dextrose incompatibility is not mentioned.Citation32
Combination therapies that result in mixing of drugs and incompatible diluents may also be a source of formation of particulate aggregates. For example, the chemotherapy agents Paraplatin® (carboplatin) or Taxol® (paclitaxel) can be administered either with 5% dextrose or 0.9% NaCl;Citation33 these chemotherapy agents are often co-administered with Avastin®. For Platinol® (cisplatin), pre-administration of large volumes, i.e., 1–2 L, of 5% dextrose containing saline and mannitol to the patient is recommended.Citation34 Administration of Avastin® to these patients, even if administered in 0.9% NaCl, may result in aggregates formation since the patient already has large amounts of dextrose in his or her bloodstream. Aggregates that form when human plasma is mixed with 5% dextrose-Avastin® solutions could be one origin of the reported “arterial thrombolytic events, including cerebral infarction, transient ischemic attacks, myocardial infarction and angina that occurred at a higher incidence in patients receiving Avastin® in combination with chemotherapy treatment as compared to those receiving chemotherapy alone.”Citation35 Other evaluation of individual patient data came to the conclusion that the addition of Avastin® to chemotherapy treatment did not statistically significantly increase the risk of venous thrombolytic events.Citation36 Our finding that Avastin® aggregates in human plasma in the presence of 5% dextrose, together with new studies of aggregation compatibility of Avastin® with different chemotherapeutic agents, may be considered in the re-evaluation of patient data and may contribute to a better understanding of the origin of reported side effects.
In summary, aggregation and interactions studies using the methods reported here open the way to study the behavior of therapeutic proteins in biological fluids. These new investigations could thereby help in the development of safe biopharmaceuticals and aid in the reduction of reported adverse events for marketed proteins caused by the use of non-compatible administration solutions or by interaction with co-administered drugs.
Materials and Methods
Materials
Sensor chips CM5, HBS-EP buffer at pH 7.4 (consisting of 10 mM 4-[2-hydroxyethyl]piperazine-1-ethane-sulfonic acid, 0.15 M sodium chloride, 3 mM EDTA, 0.005% (v/v) polysorbate 20), amine coupling kit containing N-hydroxysuccinimide (NHS), N-ethyl-N′-(3-diethylaminopropyl)carbodiimide (EDC), and ethanolamine hydrochloride were obtained from Biacore AB. ELISA wash buffer and substrate, and 4-methylumbelliferyl phosphate were purchased from Virolabs. Fibrinogen, transferrin, albumin from human sources, sodium chloride and dextrose were purchased from Sigma-Aldrich. Humanized mAbs referred to as A1-A8, B1, B2 and C2 were produced and purified in Merck Research Laboratories. The mAbs referred to as C1 (Humira®, adalimumab, Abbott Laboratories) and C3 (Erbitux®, cetuximab, ImClone), bevacizumab (Avastin®, Roche), trastuzumab (Herceptin®, Roche) and infliximab (Remicade®, Janssen Biotech) are commercially available and were purchased. All antibodies were analyzed prior to the expiration date. The stock solutions of Herceptin®, Avastin® and Remicade® consisted of:Citation22 (1) Herceptin® lyophilized formulation after reconstitution with water was 21 mg/ml trastuzumab in 20 mg/ml (52.9 mM) α, α-trehalose dihydrate, 0.5 mg/ml (2.4 mM) l-histidine HCl, 0.32 mg/ml (2.1 mM) l-histidine, 0.09 mg/ml (0.009% w/v) polysorbate 20, pH 6; (2) Avastin® is a liquid formulation of 25 mg/ml bevacizumab in 60 mg/ml (158.6 mM) α, α-trehalose dihydrate, 5.8 mg/ml (42 mM) monobasic sodium phosphate monohydrate, 1.2 mg/ml (8.5 mM) dibasic sodium phosphate anhydrous, 0.4 mg/ml (0.04% w/v) polysorbate 20, pH 6.2; and (3) Remicade® lyophilized formulation after reconstitution with water was 10 mg/ml infliximab in 50 mg/ml (146 mM) sucrose, 0.22 mg/ml (1.6 mM) monobasic sodium phosphate monohydrate, 0.61 mg/ml (3.4 mM) dibasic sodium phosphate dihydrate, 0.05 mg/ml (0.005% w/v) polysorbate 80, pH 7.2.
Human sera were obtained from BioChemed Services. Human plasma bags were purchased from Blutspendezentrum SRK beider Basel.
Light microscopy
2.5 μl of antibodies (Avastin®, Herceptin® and Remicade®) diluted to ~1 mg/ml in 0.9% sodium chloride or 5% dextrose in water, were placed inside a FastRead 102™ slide (Immune Systems). Then, 2.5 μl of human plasma were added inside the slide so that it came in contact with the mAb solution already present; no mixing was performed. A Leica DM RXE microscope (Leica Microsystems GmbH) with a 5×/0.15 na objective (Leica Microsystems GmbH) was used. The images were acquired with a Sony NEX-5 camera and its firmware, and processed using the ImageJ version 1.43u software (National Institutes of Health). The grid of 250 μm × 250 μm on the FastRead 102™ slide was used to calibrate the relation between pixels and lengths. The microscopy pictures in binary form were analyzed for size distribution using the Feret’s diameter mode.
90° light-scattering
90° light-scattering measurements were performed with a FluoroMax spectrofluorometer (Spex) at 25°C in a thermostated cuvette holder. The spectra were recorded between 500 nm and 750 nm with a 0.02 sec integration time per 1 nm increment. The excitation and emission slits were 1 mm. First, 10 μl of human plasma were added to 3 ml of 0.9% sodium chloride or 5% dextrose in water, and the 90° light-scatter spectrum was recorded. Then 20 μl (Avastin®, Herceptin®) or 40 μl (Remicade®) undiluted antibody solution were mixed in the cuvette containing the 10 μl human plasma in the 3 ml solution of either 0.9% sodium chloride or 5% dextrose in water.
Surface Plasmon Resonance
A Biacore® 2000 instrument (Biacore) was used. The carboxymethylated dextran layer on the sensor chip surface was activated by derivatization through injection of 30 μl of 0.2 M EDC containing 50 mM NHS at an operating temperature of 25°C, giving a sensor response between 50 and 200 response units (RU). This activation procedure was repeated for each of the four flow cells numbered 1 through 4 on the same chip. mAb solutions were injected continuously and the immobilization was aimed typically at 10,000–20,000 RU. After covalent coupling of the mAbs, the surface was deactivated with 30 μl of 1.0 M ethanolamine-hydrochloride (pH 8.5). After each injection of a neat serum sample (no dilution), the response was recorded during the dissociation stage (at 200 sec since the start), followed by two injections of 50 mM NaOH to regenerate the surface. The quantitative analysis was performed within Microsoft® Excel™ environment.
Abbreviations: | ||
surface plasmon resonance | = | SPR |
monoclonal antibody | = | mAb |
N-hydroxysuccinimide | = | NHS |
N-ethyl-N′-(3-diethylaminopropyl)carbodiimide | = | (EDC) |
Acknowledgments
The authors are grateful to Jon Condra, Donna Montgomery and James Drummond for providing monkey and mouse versions of the antibodies and useful discussions. We also thank Qinjian Zhao for providing fibrinogen-related data and Bei Wang for the assistance with setting up the instrument.
Disclosure of Potential Conflicts of Interest
No potential conflicts of interest were disclosed.
References
- Schellekens H. Immunogenicity of therapeutic proteins: clinical implications and future prospects. Clin Ther 2002; 24:1720 - 40, discussion 1719; http://dx.doi.org/10.1016/S0149-2918(02)80075-3; PMID: 12501870
- Schellekens H. Bioequivalence and the immunogenicity of biopharmaceuticals. Nat Rev Drug Discov 2002; 1:457 - 62; http://dx.doi.org/10.1038/nrd818; PMID: 12119747
- Macdougall IC. Epoetin-induced pure red cell aplasia: diagnosis and treatment. Curr Opin Nephrol Hypertens 2007; 16:585 - 8; http://dx.doi.org/10.1097/MNH.0b013e3282f0c4bf; PMID: 18089975
- Schellekens H, Jiskoot W. Erythropoietin-associated PRCA: still an unsolved mystery. J Immunotoxicol 2006; 3:123 - 30; http://dx.doi.org/10.1080/15476910600845567; PMID: 18958692
- Chennamsetty N, Voynov V, Kayser V, Helk B, Trout BL. Prediction of aggregation prone regions of therapeutic proteins. J Phys Chem B 2010; 114:6614 - 24; http://dx.doi.org/10.1021/jp911706q; PMID: 20411962
- Koren E, De Groot AS, Jawa V, Beck KD, Boone T, Rivera D, et al. Clinical validation of the “in silico” prediction of immunogenicity of a human recombinant therapeutic protein. Clin Immunol 2007; 124:26 - 32; http://dx.doi.org/10.1016/j.clim.2007.03.544; PMID: 17490912
- Singh SK, Afonina N, Awwad M, Bechtold-Peters K, Blue JT, Chou D, et al. An industry perspective on the monitoring of subvisible particles as a quality attribute for protein therapeutics. J Pharm Sci 2010; 99:3302 - 21; http://dx.doi.org/10.1002/jps.22097; PMID: 20310025
- Carpenter JF, Randolph TW, Jiskoot W, Crommelin DJA, Middaugh CR, Winter G, et al. Overlooking subvisible particles in therapeutic protein products: gaps that may compromise product quality. J Pharm Sci 2009; 98:1201 - 5; http://dx.doi.org/10.1002/jps.21530; PMID: 18704929
- Saluja A, Kalonia DS. Nature and consequences of protein-protein interactions in high protein concentration solutions. Int J Pharm 2008; 358:1 - 15; http://dx.doi.org/10.1016/j.ijpharm.2008.03.041; PMID: 18485634
- Mahler HC, Friess W, Grauschopf U, Kiese S. Protein aggregation: pathways, induction factors and analysis. J Pharm Sci 2009; 98:2909 - 34; http://dx.doi.org/10.1002/jps.21566; PMID: 18823031
- Kanai S, Liu J, Patapoff TW, Shire SJ. Reversible self-association of a concentrated monoclonal antibody solution mediated by Fab-Fab interaction that impacts solution viscosity. J Pharm Sci 2008; 97:4219 - 27; http://dx.doi.org/10.1002/jps.21322; PMID: 18240303
- Demeule B, Palais C, Machaidze G, Gurny R, Arvinte T. New methods allowing the detection of protein aggregates: a case study on trastuzumab. MAbs 2009; 1:142 - 50; http://dx.doi.org/10.4161/mabs.1.2.7632; PMID: 20061815
- Demeule B, Gurny R, Arvinte T. Detection and characterization of protein aggregates by fluorescence microscopy. Int J Pharm 2007; 329:37 - 45; http://dx.doi.org/10.1016/j.ijpharm.2006.08.024; PMID: 17005340
- Demeule B, Lawrence MJ, Drake AF, Gurny R, Arvinte T. Characterization of protein aggregation: the case of a therapeutic immunoglobulin. Biochim Biophys Acta 2007; 1774:146 - 53; http://dx.doi.org/10.1016/j.bbapap.2006.10.010; PMID: 17142116
- Harn N, Spitznagel T, Perkins M, Allan C, Shire S, Middaugh CR. Biophysical signatures of monoclonal antibodies. Biotechnology Pharmaceutical Aspects 2010; 11:229 - 46
- Liu J, Nguyen MDH, Andya JD, Shire SJ. Reversible self-association increases the viscosity of a concentrated monoclonal antibody in aqueous solution. J Pharm Sci 2005; 94:1928 - 40; http://dx.doi.org/10.1002/jps.20347; PMID: 16052543
- Saluja A, Kalonia DS. Measurement of fluid viscosity at microliter volumes using quartz impedance analysis. AAPS PharmSciTech 2004; 5:e47; http://dx.doi.org/10.1208/pt050347; PMID: 15760080
- Volkin DB, Mach H, Middaugh CR. Degradative covalent reactions important to protein stability. Mol Biotechnol 1997; 8:105 - 22; http://dx.doi.org/10.1007/BF02752255; PMID: 9406181
- Capelle MAH, Gurny R, Arvinte T. High throughput screening of protein formulation stability: practical considerations. Eur J Pharm Biopharm 2007; 65:131 - 48; http://dx.doi.org/10.1016/j.ejpb.2006.09.009; PMID: 17107777
- Demeule B, Shire SJ, Liu J. A therapeutic antibody and its antigen form different complexes in serum than in phosphate-buffered saline: a study by analytical ultracentrifugation. Anal Biochem 2009; 388:279 - 87; http://dx.doi.org/10.1016/j.ab.2009.03.012; PMID: 19289095
- Filipe V, Poole R, Oladunjoye O, Braeckmans K, Jiskoot W. Detection and characterization of subvisible aggregates of monoclonal IgG in serum. Pharm Res 2012; 29:2202 - 12; http://dx.doi.org/10.1007/s11095-012-0749-x; PMID: 22467219
- Herceptin®, Avastin® and Remicade® prescribing information. US Food and Drug Administration www.accessdata.fda.gov/scripts/cder/drugsatfda/
- Neupogen® prescribing information. US Food and Drug Administration www.accessdata.fda.gov/scripts/cder/drugsatfda/
- USP home page: http://www.usp.org/; search “<788<Particulate Matter in Injections” or document m99586-gc_788-1.pdf.
- Malmqvist M, Karlsson R. Biomolecular interaction analysis: affinity biosensor technologies for functional analysis of proteins. Curr Opin Chem Biol 1997; 1:378 - 83; http://dx.doi.org/10.1016/S1367-5931(97)80077-4; PMID: 9667873
- Karlsson R, Kullman-Magnusson M, Hämäläinen MD, Remaeus A, Andersson K, Borg P, et al. Biosensor analysis of drug-target interactions: direct and competitive binding assays for investigation of interactions between thrombin and thrombin inhibitors. Anal Biochem 2000; 278:1 - 13; http://dx.doi.org/10.1006/abio.1999.4406; PMID: 10640347
- Roos H, Karlsson R, Nilshans H, Persson A. Thermodynamic analysis of protein interactions with biosensor technology. J Mol Recognit 1998; 11:204 - 10; http://dx.doi.org/10.1002/(SICI)1099-1352(199812)11:1/6<204::AID-JMR424>3.0.CO;2-T; PMID: 10076841
- Humira® prescribing information. AbbVie. www.rxabbott.com/u4_prescribing_info.cfm
- George TJ Jr., Laplant KD, Walden EO, Davis AB, Riggs CE, Close JL, et al. Managing cetuximab hypersensitivity-infusion reactions: incidence, risk factors, prevention, and retreatment. J Support Oncol 2010; 8:72 - 7; PMID: 20464886
- Garvan JM, Gunner BW. The harmful effects of particles in intravenous fluids. Med J Aust 1964; 2:1 - 6; PMID: 14175312
- Franke RP. Quantifizierung partikuläre Baustanteile in der Mikrozirkulation der Lunge. Beitr Elektronenmikroskop Dirrektabb Oberfl 1986; 19:429 - 34
- Avastin® prescribing information: www.roche.fr/fmfiles/re7199006/notices/avastin/Avastin25mg-ml10132500MAJ08-08-11.pdf
- Paraplatin® and Taxol® prescribing information: packageinserts.bms.com/pi/pi_paraplatin.pdf packageinserts.bms.com/pi/pi_taxol.pdf.
- Platinol® prescribing information. US Food and Drug Administration www.accessdata.fda.gov/scripts/cder/drugsatfda/
- FDA. “Important Drug Warning” Letter to Genentech, January 5, 2005 on the “increased risk of arterial thromboembolic events associated with the use of AVASTINTM (Bevacizumab) in combination with chemotherapy.” see: www.fda.gov/downloads/Safety/MedWatch/SafetyInformation/ SafetyAlertsforHumanMedicalProducts/UCM164188.pdf and www.fda.gov/Safety/MedWatch/SafetyInformation/ SafetyAlertsforHumanMedicalProducts/ucm150721.htm.
- Hurwitz HI, Saltz LB, Van Cutsem E, Cassidy J, Wiedemann J, Sirzén F, et al. Venous thromboembolic events with chemotherapy plus bevacizumab: a pooled analysis of patients in randomized phase II and III studies. J Clin Oncol 2011; 29:1757 - 64; http://dx.doi.org/10.1200/JCO.2010.32.3220; PMID: 21422411