Abstract
Alu repeats within human genes may potentially alter gene expression. Here, we show that 3′-UTR-located inverted Alu repeats significantly reduce expression of an AcGFP reporter gene. Mutational analysis demonstrates that the secondary structure, but not the primary nucleotide sequence, of the inverted Alu repeats is critical for repression. The expression levels and nucleocytoplasmic distribution of reporter mRNAs with or without 3′-UTR inverted Alu repeats are similar; suggesting that reporter gene repression is not due to changes in mRNA levels or mRNA nuclear sequestration. Instead, reporter gene mRNAs harboring 3′-UTR inverted Alu repeats accumulate in cytoplasmic stress granules. These findings may suggest a novel mechanism whereby 3′-UTR-located inverted Alu repeats regulate human gene expression through sequestration of mRNAs within stress granules.
Introduction
Alu repeat elements are the most abundant repetitive elements in the human genome. The roughly 1.4 million Alu repeats in human DNA, account for at least 12% of the mass of the human genome.Citation1 The vast majority of Alu repeats are found in introns; however, approximately 5% of fully spliced cDNAs harbor Alu repeats, with 82% of these Alus located in 3′ untranslated regions (3′-UTRs), 14% in 5′ untranslated regions (5′-UTRs) and 4% within coding regions.Citation2
UTRs play important roles in the post-transcriptional regulation of gene expression, including regulation of mRNA localization, stability, and translation.Citation3-Citation6 Alu elements inserted in 3′-UTRs may be potential sources of adenine and uracil-rich elements or AREs. In silico analysis suggests that antisense Alu repeats inserted in 3′-UTRs could generate AREs.Citation7 AREs are known to affect the stability and subsequent expression of mRNAs.Citation8-Citation10 Additionally, Alu repeats within 3′-UTRs are potential target sites for microRNAs (miRNAs), small noncoding RNAs that influence gene expression post-transcriptionally by repressing translation or causing mRNA degradation. Computer analysis for sequence complementarity between Alu repeats located in mRNAs and miRNAs showed that sense Alu sequences are enriched for potential miRNA target sites.Citation11
Recent findingsCitation12 suggest a role for 3′-UTR Alus in Staufen 1 (STAU1)-mediated mRNA decay (SMD). It was shown experimentally that base-pairing between an Alu repeat in the 3′-UTR of an mRNA and another Alu repeat located within a cytoplasmic polyadenylated long non-coding RNA (lncRNA) forms RNA-RNA duplexes that are targets for SMD.Citation12
Inverted repeats in the 3′-UTRs of mRNAs have recently been shown to regulate gene expression by mRNA nuclear retention. Localization and translation of the 8 kb mouse Cat2 transcribed nuclear-RNA (CTN-RNA) is influenced by inverted Alu-like repeats in the 3′-UTR of the transcript.Citation13 The inverted repeats are proposed to form regions of double-stranded RNA (dsRNA) that are targets for adenosine deaminases acting on RNA (ADARs), RNA-editing enzymes which convert adenosine residues in dsRNA to inosine.Citation14 Adenosine-to-inosine (A-to-I) edited CTN-RNA is then bound by p54nrb, a multifunctional nuclear RNA binding protein, and retained in nuclear paraspeckles.Citation13 In another study, a pair of inverted Alu repeats in the 3′-UTR of EGFP reporter mRNA caused nuclear retention of the EGFP mRNA and reduced EGFP protein expression.Citation15 Nuclear retention also correlated with A-to-I hyperediting and binding of p54nrb to the RNA.
Although recent findings have provided evidence for the repression of gene expression by 3′-UTR-located inverted Alu repeats, the precise mechanism(s) of this repressive action remain controversial. In the present study, we utilized a reporter gene expression system to examine the molecular mechanisms underlying gene repression by 3′-UTR inverted Alu repeats. Consistent with previous findings,Citation15 we show that expression of an AcGFP reporter gene is significantly reduced by inverted Alu repeats in the 3′-UTR. Mutational analysis of the 3′-UTR inverted Alu repeats indicates that the secondary structure of the inverted Alu repeats, but not the primary nucleotide sequence, is critical for repression of reporter gene expression. The mRNAs with 3′-UTR-located inverted Alu repeats are not extensively edited and are not retained in the nucleus, but instead, accumulate in cytoplasmic stress granules. These results, together with previous studies, suggest that Alu repeats within the 3′-UTRs of human genes can modulate gene expression through multiple mechanisms.
Results
AcGFP reporter gene expression is strongly repressed by inverted repeat Alu elements in the 3′-UTR region of the gene
To study the effects of 3′-UTR inverted Alu repeats on gene expression, a reporter gene construct was generated by inserting inverted Alu repeats (a sense-oriented AluJb repeat and an antisense AluYa5 repeat separated by 162 base pairs) into the 3′-UTR region of the AcGFP reporter gene in the expression vector pAcGFP1-C1. The resulting plasmid was designated pAluIR. To evaluate the impact of a single sense or antisense Alu on gene expression, derivative constructs containing either the single antisense AluYa5 or the sense AluJb repeat were generated from pAluIR and designated pAluYa5 and pAluJb, respectively. All constructs carried the AcGFP coding sequence driven by the human cytomegalovirus (CMV) immediate early promoter. Plasmid constructs are represented schematically in . The details of plasmid construction are outlined in the Materials and Methods.
Figure 1. Expression of an AcGFP reporter gene is inhibited by 3′-UTR-located inverted Alu repeats . (A) Schematic representation of AcGFP expression constructs. The AcGFP gene of all constructs is expressed from the CMV promoter. (B) western blot analysis of total AcGFP protein shows significantly reduced AcGFP protein levels in 293 cells transfected by an AcGFP expression construct with 3′-UTR inverted Alus (lane 4) relative to cells transfected with an AcGFP control (lane 1). The expression level of AcGFP protein was determined by western blot 48 h post-transfection and normalized to that of β-galactosidase as a transfection control and to α-tubulin as a loading control. Figures are representative of five independent experiments. **p = 0.0031, paired t-test. (C) Bar graph densitometry of western blot bands expressed in arbitrary units. Data are expressed as mean normalized expression, using β-galactosidase and α-tubulin, and are presented as means ± s.e.m. of five independent experiments. The value obtained from the control vector, pAcGFP1-C1, was set to 1. Quantification of images was done by scanning densitometry with NIH Image J 1.54 software (National Institutes of Health, Bethesda, MD, USA).
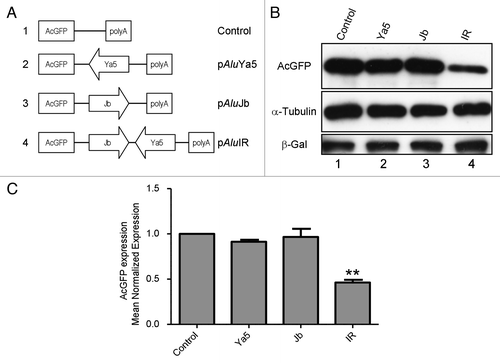
The inverted Alu 3′-UTR construct (pAluIR), its single Alu derivatives (pAluJb and pAluYa5), and the parental AcGFP control vector (pAcGFP1-C1) were each transfected into HEK293 cells using the calcium phosphate DNA precipitation method.Citation16 At 48 h post-transfection, the AcGFP expression level from each plasmid was measured by Western Blot. AcGFP expression was normalized to β-galactosidase expression, as a measure of transfection efficiency and to α-tubulin, as a loading control. All experiments were performed at least five times.
AcGFP reporter gene expression was not significantly altered by a 3′-UTR-located single sense or antisense Alu repeat (, lanes 2 and 3). In contrast, AcGFP expression was significantly reduced by the 3′-UTR-located inverted Alu repeats (, lane 4). These results are consistent with a previous report that 3′-UTR inverted Alu repeats reduced reporter gene expression, while single antisense or sense Alu repeats had little impact on gene expression.Citation15
Inverted Alu repeat secondary structure contributes to AcGFP repression
A previous studyCitation15 suggested that stem-loop secondary structure contributed by 3′-UTR-located inverted Alu repeats may function to repress gene expression. Inverted Alu secondary structure formation was indirectly inferred from observations of A-to-I editing of the Alu repeats. However, the formation of inverted Alu secondary structure and its direct contribution to repression of gene expression remains unclear.
Repression of gene expression by 3′-UTR-located inverted Alu repeats could be mediated by specific nucleotide sequences within the inverted Alu repeats, such as adenine and uracil-rich elements (AREs) that have been shown to regulate mRNA stability.Citation17-Citation19 Alternatively, the inverted Alu sequences could provide structural motifs, such as the stem-loop required for ASH1 mRNA localization in budding yeast.Citation20
To test whether the secondary structure or the primary nucleotide sequence of the 3′-UTR inverted Alus contributes to repression of reporter gene expression, a systematic substitution mutagenesis approach was used. Primary sequence changes designed to disrupt a potential stem-loop arising from Alu-Alu base pairing were introduced into the 3′-UTR inverted Alu pair. In one mutant, pIRsubJb, each nucleotide within the sense AluJb sequence of the inverted Alu pair was altered by purine-to-purine and pyrimidine-to-pyrimidine substitutions. A second mutant, pIRsubYa5, was generated by purine-to-purine and pyrimidine-to-pyrimidine substitutions of each nucleotide within the antisense AluYa5 sequence of the inverted Alu pair. In addition, a compensatory mutant (pIRcompensatory) which alters both the AluJb and the AluYa5 sequences of the inverted Alu pair, but restores base pairing with altered non-Alu sequences was generated. Plasmid constructs are represented schematically in . Details of mutant plasmid construction are described in the Materials and Methods. The complete nucleotide sequence of the wild-type Alu repeats and the synthetic substitution fragments are shown in Fig. S1.
Figure 2. Inverted Alu repeat secondary structure contributes to AcGFP repression. (A) Schematic representation of mutant plasmid constructs. The AcGFP gene for all constructs is expressed from the CMV promoter. AluYa5 substitution mutation (Arrow with diamonds); AluJb substitution mutation (Arrow with diagonal lines). (B) western blot analysis of HEK293 cells transfected with constructs expressing an AcGFP gene (Control), an AcGFP gene with 3′-UTR-located inverted Alus (IR) or an AcGFP gene with 3′-UTR mutant derivatives of the inverted Alu repeats (IRsubYa5, IRsubJb, and IRcompensatory). Substitution of either the sense AluJb (IRsubJb) or the antisense AluYa5 (IRsubYa5) of the inverted Alu pair relieved the inverted Alu repeat-mediated repression of AcGFP expression (lanes 3 and 4). Repression of AcGFP expression is maintained by a compensatory mutant (lane 5). The expression level of AcGFP protein was detected by western blot at 48hrs post-transfection and normalized to that of β-galactosidase as a transfection control and to α-tubulin as a loading control. Figures are representative of five independent experiments. ***p = 0.0005, **p = 0.0048; paired t-test. (C) Bar graph densitometry of western blot bands expressed in arbitrary units. Data are expressed as mean normalized expression, using β-galactosidase and α-tubulin, and are presented as means ± s.e.m. of five independent experiments. The value obtained from the control vector, pAcGFP1-C1, was set to 1. Quantification of images was done by scanning densitometry with NIH Image J 1.54 software (National Institutes of Health, Bethesda, MD, USA).
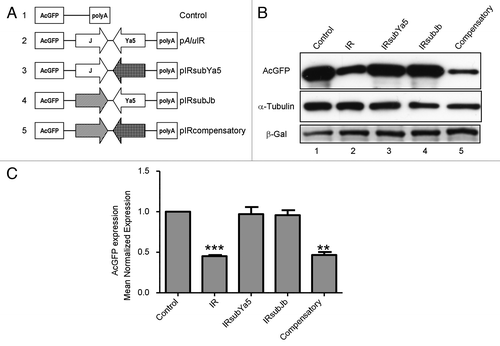
The 3′-UTR inverted Alu substitution mutants were tested by transient transfection for their ability to repress AcGFP reporter gene expression. AcGFP reporter gene expression from each plasmid was measured by western blot 48 h after transfection. Western blot analysis of cotransfected β-galactosidase was used to normalize transfection efficiency (). AcGFP expression was normalized to α-tubulin, as a loading control. All experiments were performed at least five times.
Substitution mutations that alter the sequence of one Alu of the inverted pair relieved the inverted Alu repeat-mediated repression of AcGFP expression. Transfection of pIRsubJb (sense Alu substitution) or pIRsubYa5 (antisense Alu substitution) into HEK293 cells resulted in similar levels of reporter gene expression compared with AcGFP control gene expression (, lanes 3 and 4). In contrast, AcGFP protein levels are reduced in compensatory mutant (pIRcompensatory) transfected HEK293 cells (, lane 5). The reduction of AcGFP expression in compensatory mutant (pIRcompensatory) transfected cells was comparable to that observed for inverted Alu repeat (pAluIR) transfected cells (, lanes 2 and 5). Taken together, these results demonstrate that the secondary structure, but not the primary nucleotide sequence of the 3′-UTR inverted Alu repeats sequence is important for reduced reporter gene expression.
Regulation of AcGFP expression occurs post-transcriptionally
3′-UTR sequences can influence mRNA transcript levels, stability, translational efficiency, and localization.Citation13,Citation15,Citation21-Citation24 To determine the mechanisms that underlie gene repression by 3′-UTR inverted Alus, we examined the steady-state levels and subcellular localization of mRNAs with or without 3′-UTR inverted Alu repeats. Northern blot analysis using 32P-labeled probes for AcGFP and GAPDH mRNAs was performed on total RNA, and RNA isolated from subcellular fractions. mRNAs encoding AcGFP and GAPDH were detected in all samples (). The size differences between the chimeric AcGFP mRNAs are due to variations in the relative lengths of their 3′-UTRs. The intensity of each northern blot band was determined by densitometry. AcGFP mRNA levels were normalized to GAPDH as a control for the amount of mRNA. Western blot analysis against α-tubulin, a cytoplasmic protein, and TATA-binding protein TFIID, a nuclear protein, was performed to check the effectiveness of fractionation ().
Figure 3. AcGFP mRNAs localize to the cytoplasm. (A) northern blot analysis of AcGFP mRNA. Equal amounts (20 μg) of total (tot), nuclear (nuc) and cytoplasmic (cyt) RNA were analyzed by northern blot using radiolabeled random-primed probes specific for AcGFP and GAPDH mRNAs. Levels and subcellular localization of AcGFP mRNA were similar for all transfected constructs. Western blot analysis against α-tubulin, a cytoplasmic protein, and TATA-binding protein TFIID, a nuclear protein, was performed to check the effectiveness of fractionation. Representative northern blots are shown. n = 5 experiments. (B) AcGFP mRNA nuclear to cytoplasmic (n/c) ratios were quantified by densitometry, normalized to GAPDH, and the obtained results from five independent experiments are represented on the graph as means ± s.d. The value obtained from the control vector, pAcGFP1-C1, was set to 1. (C) northern blot analysis of AcGFP mRNA. Equal amounts (20 μg) of total (tot), nuclear (nuc) and cytoplasmic (cyt) RNA were analyzed by northern blot using radiolabeled random-primed probes specific for AcGFP and GAPDH mRNAs. Levels and subcellular localization of AcGFP mRNA were similar for all transfected constructs. Western blot analysis against α-tubulin, a cytoplasmic protein, and TATA-binding protein TFIID, a nuclear protein, was performed to check the effectiveness of fractionation. Representative northern blots are shown. n = 5 experiments. (D) AcGFP mRNA nuclear to cytoplasmic (n/c) ratios were calculated as described above and the obtained results from five independent experiments are represented on the graph as means ± s.d.
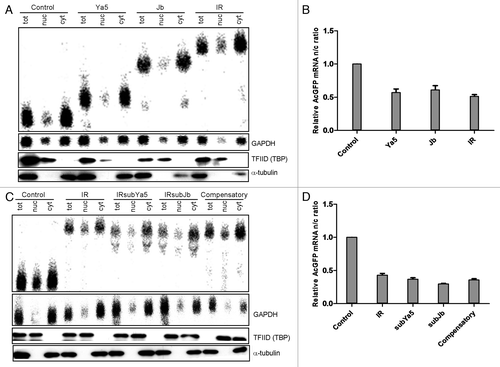
Northern blot analysis of cytoplasmic and nuclear RNA extracts shows localization of AcGFP mRNA primarily to the cytoplasm for all transfected constructs (). The nuclear to cytoplasmic (n/c) RNA ratio of AcGFP mRNA indicates that there is more AcGFP mRNA in the cytoplasmic compartment than in the nucleus (). These findings suggest that repression of AcGFP reporter gene expression by 3′-UTR inverted Alu repeats, is not likely due to nuclear retention of AcGFP mRNA or decreased AcGFP mRNA levels.
Chimeric AcGFP mRNA accumulates in cytoplasmic foci and granule-like structures
As demonstrated by northern blot analysis, chimeric AcGFP reporter mRNAs are largely distributed in the cytoplasm of transfected HEK293 cells and steady-state mRNA levels are not significantly changed. It is, therefore, unlikely that repression of AcGFP reporter gene expression is due to significant transcription inhibition or mRNA degradation. To determine the mechanism of repression, we examined the cytoplasmic fate of the reporter gene mRNA. RNA FISH using biotin-labeled AcGFP DNA as probes was used to localize AcGFP mRNA in transfected cells. The results show that chimeric AcGFP mRNAs in HEK293 cells transfected by 3′-UTR inverted Alu repeat constructs accumulate in discrete cytoplasmic foci (, left panels) and cytoplasmic granule-like structures (, left panels). By comparison, AcGFP mRNAs are diffusely distributed in the cytoplasm of AcGFP control vector transfected cells and in cells transfected by 3′-UTR constructs with a single sense Alu (pAluJb) or a single antisense Alu (pAluYa5) ( and , left panels).
Figure 4. (A) AcGFP reporter mRNA interacts with P-bodies. AcGFP mRNA (red) interacts with GW182-stained P-bodies (Cy5, pseudocolored yellow) in transfected HEK293 cells. Nuclei are stained with DAPI (blue). Right panels represent a merge of the RNA, GW182, GFP, and DAPI signals. White arrowheads indicate AcGFP mRNA foci. White arrows indicate colocalization of AcGFP mRNA and GW182 in P-bodies. Scale bars: 10 μm. (B) Quantification of the percentage of cells with co-localized AcGFP mRNA and P-bodies. Percentages are averages of three independent experiments in which five random microsope fields were selected and at least 100 cells scored for colocalization of AcGFP mRNA with P-bodies.
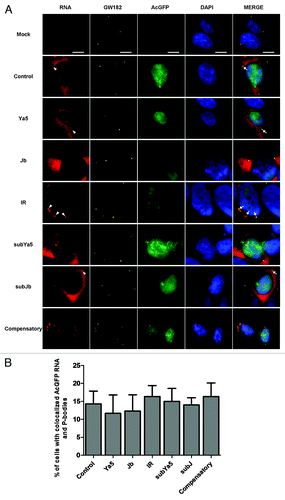
Figure 5. AcGFP reporter mRNAs containing 3′-UTR inverted Alu repeats accumulate into stress granules. AcGFP transcripts carrying 3′-UTR inverted Alu repeats (red) co-localize with eIF3η (Cy5, pseudocolored yellow) into stress granules. The presence of AcGFP mRNA (red) aggregates that co-localize with eIF3η (Cy5, pseudocolored yellow) into stress granules was restricted to 293 cells transfected by pAluIR or pIRcompensatory. Nuclei are stained with DAPI (blue). Right panels represent a merge of the RNA, eIF3η, GFP, and DAPI signals. White arrowheads indicate AcGFP mRNA granules. White arrows indicate colocalization of AcGFP mRNA and eIF3η into stress granules. Scale bars: 10 μm. (B) Quantification of the percentage of cells with co-localized AcGFP mRNA and stress granules. Percentages are averages of three independent experiments in which five random microsope fields were selected and at least 100 cells scored for colocalization of AcGFP mRNA with stress granules.
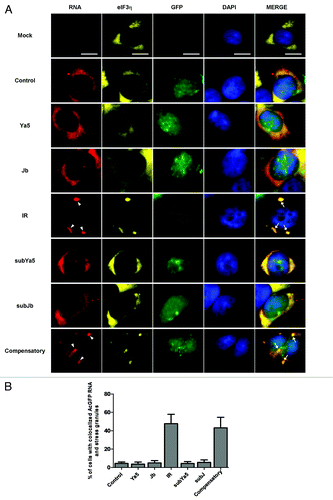
Increasing evidence shows that cytoplasmic RNA granules, such as stress granules and P-bodies, influence the stability, localization, and translation of mRNA.Citation25-Citation28 To determine if the inverted Alu repeat-containing reporter mRNA associates with P-bodies or stress granules, AcGFP RNA FISH was combined with indirect immunofluorescence using antibodies against GW182 (a P-body marker), or eIF3η, (a stress granule marker). The results indicate that in 3′-UTR inverted Alu repeat (pAluIR) transfected cells a significant fraction of chimeric AcGFP mRNA is concentrated in P-bodies and stress granules, as demonstrated by colocalization of the mRNA with GW182 () and eIF3η (). In contrast, while AcGFP transcripts with or without a single sense or single antisense Alu in their 3′-UTRs are readily detected in P-bodies ( and ) these mRNAs were absent from stress granules (). Stress granules were more prominent in cells expressing reporter mRNAs with 3′-UTR-located Alus (pAluIR) than in those expressing AcGFP mRNA without 3′-UTR inverted Alus (). Additionally, very little of the 3′-UTR inverted Alu mRNA was detectable outside of stress granules. These findings suggest that the 3′-UTR inverted Alu repeats may promote the formation of stress granules, where expression of the inverted Alu-containing mRNA is repressed.
It has been reported that RNAs with hyperedited 3′-UTR-located inverted repeats are retained within nuclear paraspeckles.Citation13,Citation15 To determine if the inverted Alu repeat-containing AcGFP reporter mRNA associates with nuclear paraspeckles, AcGFP RNA FISH was combined with indirect immunofluorescence using antibodies against PSPC1 (paraspeckle protein 1), a known protein component of paraspeckles, was performed. Colocalization of AcGFP reporter transcripts with nuclear PSPC1 was not observed, indicating that AcGFP mRNAs with 3′-UTR inverted Alus are not retained within nuclear paraspeckles (unpublished data). These findings are consistent with northern blot analyses which show very little nuclear accumulation of AcGFP mRNA ().
Inverted Alus RNAs are not extensively A-to-I edited
A-to-I RNA editing is the post-transcriptional deamination of adenosine to inosine in dsRNA catalyzed by ADAR (adenosine deaminases acting on RNA) enzymes. The number of deamination events that occur is determined by the secondary structure of the substrate RNA.Citation29,Citation30 RNA that is almost completely double stranded is deaminated at more sites than RNA whose structure is interrupted by mismatches, bulges, and loops.
Several studies have suggested that A-to-I hyperediting of repetitive elements in mRNA transcripts can influence gene expression.Citation13,Citation15,Citation31,Citation32 To determine if the 3′-UTR inverted Alu repeats in AcGFP reporter mRNAs are subject to A-to-I editing we compared multiple cDNA sequences of these RNAs to matching genomic sequences. Because inosine is read as guanosine by reverse transcriptase in the RT reaction, A-to-I editing events are detected as A-to-G transitions in cDNA sequences.
Promiscuous editing (50 – 60% deamination of adenosines) of the inverted Alus was not detected (Fig. S2). RNA editing occurred at only two positions within the AluJb repeat (~1.6% of the total adenosines appeared as guanosines) and at four positions within the antisense AluYa5 repeat (9% of the total adenosines appeared as guanosines).
Discussion
The 3′-UTRs of human mRNAs frequently contain Alu sequences.Citation2 Recent findings have provided evidence for the repression of gene expression by 3′-UTR-located inverted Alu repeats.Citation15 However, the precise mechanism(s) of this repressive action remain unclear. To systematically examine the molecular mechanisms that underlie gene repression by 3′-UTR inverted Alus, we compared the expression, distribution, and subcellular localization of mRNAs with or without 3′-UTR inverted Alu repeats. Consistent with previous findings,Citation15 we found that 3′-UTR-located inverted Alu repeats significantly reduced reporter gene expression while, in contrast, a 3′-UTR-located single sense or antisense Alu did not alter reporter gene expression.
Steady-state abundance and subcellular distribution of AcGFP reporter mRNAs with or without 3′-UTR inverted Alus were similar; indicating that the repression of AcGFP expression by 3′-UTR inverted Alus is not a direct consequence of decreased transcription or reduced mRNA stability. AcGFP reporter mRNA was predominantly localized to the cytoplasm for all transfected constructs; indicating that repression of AcGFP expression by 3′-UTR inverted Alu repeats may occur at the post-transcriptional level in the cytoplasm.
Combined RNA in situ hybridization and immunofluorescence demonstrates that mRNAs with 3′-UTR inverted Alus accumulate in stress granules, as demonstrated by their colocalization with the stress granule marker, eIF3η (). By comparison, mRNAs with 3′-UTR single Alus and those with 3′-UTRs lacking Alu repeats do not localize to stress granules. All of the reporter mRNAs, both those with 3′-UTR inverted or single Alus and those with 3′-UTRs lacking Alus, associated with the P-body marker GW182. Together these findings indicate that mRNAs with 3′-UTR inverted Alu repeats selectively associate with stress granules.
Compensatory substitution mutagenesis that restores the predicted stem-loop structure of the inverted repeats demonstrates that the secondary structure, but not the primary nucleotide sequence, of the 3′-UTR inverted Alu repeats is critical for repression of gene expression and stress granule association. RNA stem-loop structures in 3′-UTRs have been previously shown to repress mRNA expression in several ways. A-to-I hyperediting of 3′-UTR inverted Alu repeats has been linked to mRNA retention in nuclear paraspeckles.Citation15 ADAR editing selectivity (the number of deamination events that occur in a substrate) is determined by the secondary structure of the substrate RNA.Citation29,Citation30 Perfectly base paired, thermodynamically stable dsRNAs are promiscuously deaminated at up to 50 to 60% of their adenines. In contrast, less stable dsRNAs; those with mismatches, bulges, and internal loops, are selectively deaminated at less than 10% of their adenines.Citation29,Citation30 It was shown experimentally that the size of the internal spacer or loop between the inverted repeats significantly impacts editing efficiency.Citation30
Because ADAR editing efficiency is influenced by the length of the dsRNA, large internal loops decrease the number of ADAR binding sites by destabilizing the secondary structure, whereas smaller loops do not.Citation30 In the study by Chen et al.,Citation15 two pairs of highly homologous, closely spaced 3′-UTR inverted Alus were analyzed. The sense and antisense repeats of one inverted Alu pair were separated by a 50 base pair internal spacer or loop. The oppositely-oriented Alus of the other inverted pair were separated by a 70 base pair internal loop. These inverted Alu substrates have the potential to form very stable stem-loop structures and according to the authors both pairs of inverted Alus are extensively edited. In our expression constructs, the oppositely-oriented Alus are separated by a 162 base pair internal loop generating a less stable dsRNA structure that is not extensively edited. This difference could account for the lack of nuclear paraspeckle association observed for inverted Alu-containing mRNAs in the present study.
In the present study, we show that mRNAs with 3′-UTR inverted Alu repeats co-localize with the stress granule marker eIF3η. The accumulation of transcripts with 3′-UTR inverted Alu repeats into stress granules would imply that translation of these mRNAs is repressed. Stress granules, cytoplasmic ribonucleoprotein (RNP) aggregates, are implicated in the post-transcriptional regulation of gene expression.Citation33 Translationally repressed mRNAs associated with abortive translation initiation complexes, and numerous RNA-binding proteins that affect mRNA expression are found in stress granules.Citation34,Citation35 Several proteins that influence mRNA stability, including HuR (Human antigen R) and TTP (tristetrapolin) accumulate in stress granules.Citation4,Citation36,Citation37 In addition, proteins implicated in translational repression, including TIA-1, TIAR, CPEB1, RAP55, and YB-1, are also found in stress granules.Citation38-Citation41 Inosine-containing dsRNAs have been shown to interact with components of stress granules and decrease reporter gene expression in trans.Citation42
Stress granule accumulation is not entirely dependent on RNA editing, but is dependent upon stem-loop secondary structure formation. While the precise details of the 3′-UTR inverted Alu repeat-mediated repression that we observe will require further study, stress granule association may block mRNA translation as previously described.Citation42 The 3′-UTR inverted Alu repeat-mediated repression may also involve microRNAs. Alu repeats within 3′-UTRs are potential target sites for miRNAs.Citation11 Additionally, effector molecules of RNAi-mediated posttranscriptional gene silencing including Argonaute proteins 1 and 2, and microRNAs, are found in stress granules.Citation43
In summary, our present study identifies a novel regulatory role for 3′-UTR inverted Alu repeats in the regulation of mRNA expression. Together with previous studies, our work demonstrates that Alu repeats within the 3′-UTRs of human genes can modulate gene expression through multiple mechanisms; including the nuclear retentionCitation15 and Staufen 1 (STAU1)-mediated mRNA decayCitation12 of mRNAs containing 3′-UTR Alus, and as we observe sequestration of mRNAs with 3′-UTR inverted Alus into stress granules. Given that the 3′-UTRs of human genes frequently harbor Alu sequences, it is quite likely that these sequences play important roles in regulating mRNA expression.
Materials and Methods
Cell culture
Human embryonic kidney cells (HEK293) were cultured in Dulbecco’s Modified Eagle’s Medium (Invitrogen, cat. no. 11965–118) supplemented with 10% (v/v) fetal bovine serum (Gemini Bio-Products, cat. no. 900–108) and 5% streptomycin/penicillin (Gibco-BRL, Invitrogen, Carlsbad, CA, USA). Cells were maintained at 37°C with 5% CO2.
Plasmid construction
To generate plasmid pAluIR, a genomic DNA fragment containing inverted Alu repeats (a sense-oriented AluJb repeat and an antisense AluYa5 repeat separated by 162 base pairs) was PCR-amplified from intron 16 of the human Angiotensin Converting Enzyme (ACE) gene. Genomic DNA was extracted from HEK293 cells using the USB PrepEase Genomic DNA Isolation Kit (USB, cat. no. 78850) according to the manufacturer’s instructions. Two oligonucleotide primers, GCCCAGCTCCCACATTAGAACAATG (forward) and GACGTGGCCATCACATTCGTCAGATC (reverse) were used to amplify the corresponding DNA fragment by PCR. PCR reactions were performed in 50 μl volumes with Platinum Taq DNA polymerase High Fidelity (Invitrogen, cat. no. 11304–011) according to the manufacturer’s instructions. The PCR conditions were as follows: 1 min of denaturation at 94°C, followed by 35 cycles of 94°C for 30 sec, 57°C for 30 sec, 68°C for 1 min, and a final extension at 68°C for 5 min.
The 1142-bp PCR product was gel purified from a 1% agarose gel using a QIAquick Gel Extraction kit (Qiagen Inc., cat. no. 28706) and cloned into TA cloning vector, pCR2.1 (Invitrogen, cat. no. K2020–20), to generate pCR2.1ACE16. Correct orientation of the subcloned ACE gene fragment, with respect to ACE genomic DNA, was determined by sequencing. An 1184-bp HindIII-BglII fragment containing the inverted Alu repeats was then isolated from pCR2.1ACE16 and subcloned into the 3′-UTR region of the AcGFP1 gene of BamHI-HindIII digested pAcGFP1-C1 (Clontech, cat. no. 632470). Plasmid pAluJb was generated from pAluIR by XbaI digestion to remove the AluYa5 repeat and self-ligation. Plasmid pAluYa5 was generated from pAluIR by SbfI-SpeI digestion to remove the AluJb repeat, blunt ending with T4 DNA polymerase (New England Biolabs, cat. no. M0203S) and self-ligation.
To generate the mutant plasmids pIRsubYa5 and pIRsubJb we utilized vector pAluIR. The wild-type full-length AluYa5 repeat or the wild-type full-length AluJb repeat of vector pAluIR was replaced by synthetic DNA fragments of the same length as the AluYa5 repeat or the AluJb repeat. To design the synthetic DNA fragments, we utilized the wild-type AluYa5 sequence or the wild-type AluJb sequence as templates. Each purine base (A or G) of the full-length AluYa5 and full-length AluJb repeats was substituted for another purine base. Likewise each pyrimidine base (C or T) of the Alu repeats was substituted for another pyrimidine. The synthetic sequences were synthesized and subcloned into pIDTSMART plasmids by Integrated DNA Technologies (IDT).
To replace the AluJb repeat in plasmid pAluIR with a synthetic DNA fragment, pAluIR was first linearized with SpeI and blunt-ended with T4 DNA polymerase (New England Biolabs, cat. no. M0203S). Linearized pAluIR was then digested with SbfI to remove the AluJb repeat. The pAluIR vector backbone was then ligated to the synthetic mutant DNA fragment isolated from BsrBI/SbfI digested pIDTSmartAluJbsub (Integrated DNA Technologies) to generate plasmid pIRsubJb. To replace the AluYa5 repeat in plasmid pAluIR with a synthetic DNA fragment, pAluIR was digested with XbaI to remove the AluYa5 repeat, blunt-ended with T4 DNA polymerase (New England Biolabs, cat. no. M0203S), and digested with SbfI. Linearized pAluIR was then treated with calf intestinal alkaline phosphatase (New England Biolabs, cat. no. M0290S) to prevent self-ligation and ligated to the synthetic mutant DNA fragment isolated from SbfI/SnaBI digested pIDTSmartAluYa5sub (Integrated DNA Technologies) to generate plasmid pIRsubYa5. A third mutant plasmid (pIRcompensatory) containing substitutions of both the AluYa5 and AluJb repeats for synthetic DNA fragments was also generated. To construct pIRcompensatory, plasmid pIRsubYa5 was digested with EcoRI, blunt-ended with T4 DNA polymerase (New England Biolabs, cat. no. M0203S), and digested with SbfI to remove the AluJb repeat. The isolated vector bone was then ligated to the substitution DNA fragment from BsrBI/SbfI digested pIDTSmartAluJbsub. Spacing between the inverted Alu repeats was recapitulated in the mutant constructs.
Western blot
HEK293 cells were cotransfected in 10 cm cell culture dishes by calcium phosphate precipitation with 10 μg of an AcGFP expression plasmid, together with 1 μg of pCMVβ (Clontech, cat. no. PT2004–5), a β-galactosidase control plasmid. Forty-eight hours post-transfection, total proteins were isolated from transfected cells using the PARIS kit (Ambion, cat. no. AM1921) according to the manufacturer’s instructions. Cell lysates were separated by electrophoresis on 12% (w/v) SDS-polyacrylamide gels and transferred to Immobilon-P polyvinylidene fluoride (PVDF) membrane (Millipore, cat. no. IPVH00010). Membranes were then blocked in 5% BSA in TBST (50 mM Tris, pH 7.6, 150 mM NaCl, 0.1% Tween-20) for one hour at room temperature. After blocking, the membranes were incubated overnight with primary antibodies against AcGFP (1:1000; Santa Cruz Biotechnology Inc.), α-tubulin (1:1000; Santa Cruz Biotechnology Inc.), and β-galactosidase (1:500; Santa Cruz Biotechnology Inc.). Blots were washed with TBST and then incubated with secondary antibodies conjugated to horseradish peroxidase (1:1000; Santa Cruz Biotechnology Inc.). After a final wash, protein bands were detected by using an ECL detection kit (Thermo Fisher Scientific Inc., cat. no. 34087), and band densities were evaluated with ImageJ software (US. National Institutes of Health, Bethesda, MD, USA).
Northern blot analysis
HEK293 cells were transiently transfected in triplicate using the calcium phosphate DNA precipitation methodCitation16 with AcGFP expression plasmids. At 48 h post-transfection, total RNA and protein was harvested from one dish. Cytoplasmic and nuclear RNA fractions were isolated from the remaining dishes. The PARIS kit (Ambion, cat. no. AM1921) was used to isolate RNA and protein from total cell lysates or from nuclear or cytoplasmic fractions according to the manufacturer’s instructions. Isolated protein was saved for western blot analysis to confirm the purity of subcellular fractions. Total, nuclear, or cytoplasmic RNA (20 μg) was mixed with an equal volume of Glyoxal Load Dye (Ambion, cat. no. AM8551) and incubated at 65°C for 15 min. After electrophoresis at 5V/cm on a 1% agarose gel, RNA was transferred onto BrightStar-Plus positively charged nylon membranes (Ambion, cat. no. AM10102) and crosslinked with UV light. Membranes were prehybridized in ULTRAhyb buffer (Ambion, cat. no. AM8670) at 42°C for 30 min. Membrane bound RNA was then hybridized with 1X10Citation6 cpm/mL of 32P-radiolabeled random-primed probes specific for AcGFP and GAPDH at 42°C overnight. Following hybridization, membranes were washed with a low stringency wash buffer (Ambion, cat. no. AM8673) at room temperature for 10 min, followed by two washes in high stringency buffer (Ambion, cat. no. AM8674) at 42°C for 15 min. Radioactivity on the membranes was visualized by phosphor imaging on a Cyclone Storage Phosphor System (PerkinElmer Inc., cat. no. C431200). ImageJ software (US. National Institutes of Health) was used to quantify bands on images generated with the Cyclone Storage Phosphor System.
Immunofluorescence, RNA FISH
Combined RNA FISH and immunofluorescence was performed as previously described.Citation44 Immunofluorescent detection of GW182, eIF3η, or PSPC1 was performed prior to RNA FISH. Briefly, transiently transfected HEK293 cells were grown on coverslips in 6-well plates and fixed with 3% paraformaldehyde in PBS at room temperature for 10 min. After being washed with PBS three times, cells were permeabilized with 0.5% Triton X-100 with 1 U/μl RNasin (Promega Biotech, cat. no. N2115) in PBS on ice for 5 min. Samples were washed three times in PBS and blocked in 1% w/v BSA with 1 U/μl RNasin (Promega Biotech, cat. no. N2115) in PBS for 15 min. at room temperature. Primary and secondary antibodies were diluted in blocking buffer with 1 U/μl RNasin (Promega Biotech, cat. no. N2115). Goat anti-eIF3η antibodies (Santa Cruz Biotechnology Inc., cat. no. sc-16377) were used at a dilution of 1:200. Human anti-GW182 autoimmune antibody 18033 (a generous gift of Dr. Marvin Fritzler) was used at a dilution of 1:200. All primary antibodies were detected with Cy5-conjugated secondary antibodies (Jackson ImmunoResearch Laboratories Inc.). Following immunofluorescent staining cells were washed three times in PBS and post-fixed with 3% paraformaldehyde in PBS at room temperature for 10 min. Samples were washed twice in 2X SSC. Twenty-five ng of biotin-labeled probes specific for AcGFP mRNA were denatured and hybridized overnight at 37°C in a humidified chamber. For detection, cells were incubated for 1 h with 7 μl of 2 µg/ml of Texas Red-conjugated streptavidin (Invitrogen, cat. no. S-872). Coverslips were mounted in DAPI-containing Vectorshield mounting medium (Vector Laboratories, cat. no. H-1200) and staining visualized on a Nikon Eclipse Ti-E inverted fluorescence microscope using NIS-Elements AR 3.2 software (Nikon).
RNA editing analysis
HEK293 cells were transfected in 10 cm cell culture dishes by calcium phosphate precipitation with pAluIR. After 48 h, the transfected cells were harvested and split into two equal parts, one part for genomic DNA extraction and the other for RNA extraction. Genomic DNA was prepared using the USB PrepEase Genomic DNA Isolation Kit (USB, cat. no. 78850) according to the manufacturer’s instructions. RNA was isolated using the PARIS kit (Ambion, cat. no. AM1921). RNAs were treated with TURBO DNA-free DNase (Ambion, cat. no. AM1907) to remove genomic DNA contamination. First-strand cDNA was reverse-transcribed from RNA with the SuperScript III First-Strand Synthesis RT-PCR kit (Invitrogen, cat. no. 18080–051) according to the manufacturer’s instructions using a gene-specific primer located downstream of the inverted Alus on the plasmid pAluIR. The resulting cDNA and isolated genomic DNA were PCR-amplified using Platinum Taq DNA polymerase High Fidelity (Invitrogen, cat. no. 11304–011) with forward and reverse oligonucleotides corresponding to the inverted Alu repeats. The cDNA-derived and genomic DNA-derived PCR products were subcloned into TA cloning vector, pCR2.1 (Invitrogen, Carlsbad, cat. no. K2020–20), transformed into E. coli, and multiple independent clones were submitted for automated DNA sequencing.
Additional material
Download Zip (307.1 KB)Disclosure of Potential Conflicts of Interest
No potential conflicts of interest were disclosed.
Acknowledgments
The authors thank Dr. Marvin Fritzler for the human anti-GW182 autoimmune antibody 18033 and Dr. David L. Spector for mouse monoclonal antibody PSPC1 9–99.
Funding
This work was supported by NIH GM grant R01GM078555 to SH.
References
- Lander ES, Linton LM, Birren B, Nusbaum C, Zody MC, Baldwin J, et al, International Human Genome Sequencing Consortium. Initial sequencing and analysis of the human genome. Nature 2001; 409:860 - 921; http://dx.doi.org/10.1038/35057062; PMID: 11237011
- Yulug IG, Yulug A, Fisher EM. The frequency and position of Alu repeats in cDNAs, as determined by database searching. Genomics 1995; 27:544 - 8; http://dx.doi.org/10.1006/geno.1995.1090; PMID: 7558040
- Mignone F, Gissi C, Liuni S, Pesole G.. Untranslated regions of mRNAs. Genome Biol 2002; 3:REVIEWS0004
- Mazumder B, Seshadri V, Fox PL. Translational control by the 3′-UTR: the ends specify the means. Trends Biochem Sci 2003; 28:91 - 8; http://dx.doi.org/10.1016/S0968-0004(03)00002-1; PMID: 12575997
- Andreassi C, Riccio A. To localize or not to localize: mRNA fate is in 3’UTR ends. Trends Cell Biol 2009; 19:465 - 74; http://dx.doi.org/10.1016/j.tcb.2009.06.001; PMID: 19716303
- Martin KC, Ephrussi A. mRNA localization: gene expression in the spatial dimension. Cell 2009; 136:719 - 30; http://dx.doi.org/10.1016/j.cell.2009.01.044; PMID: 19239891
- An HJ, Lee D, Lee KH, Bhak J. The association of Alu repeats with the generation of potential AU-rich elements (ARE) at 3′ untranslated regions. BMC Genomics 2004; 5:97; http://dx.doi.org/10.1186/1471-2164-5-97; PMID: 15610565
- Chakkalakal JV, Miura P, Bélanger G, Michel RN, Jasmin BJ. Modulation of utrophin A mRNA stability in fast versus slow muscles via an AU-rich element and calcineurin signaling. Nucleic Acids Res 2008; 36:826 - 38; http://dx.doi.org/10.1093/nar/gkm1107; PMID: 18084024
- Xin H, Brown JA, Gong C, Fan H, Brewer G, Gnarra JR. Association of the von Hippel-Lindau protein with AUF1 and post-transcriptional regulation of Vascular Endothelial Growth Factor A mRNA. Mol Cancer Res 2011; PMID: 22086907
- McGray AJ, Gingerich T, Petrik JJ, Lamarre J. Regulation of thrombospondin-1 expression through AU-rich elements in the 3’UTR of the mRNA. Cell Mol Biol Lett 2011; 16:55 - 68; http://dx.doi.org/10.2478/s11658-010-0037-x; PMID: 21161418
- Smalheiser NR, Torvik VI. Alu elements within human mRNAs are probable microRNA targets. Trends Genet 2006; 22:532 - 6; http://dx.doi.org/10.1016/j.tig.2006.08.007; PMID: 16914224
- Gong C, Maquat LE. lncRNAs transactivate STAU1-mediated mRNA decay by duplexing with 3′ UTRs via Alu elements. Nature 2011; 470:284 - 8; http://dx.doi.org/10.1038/nature09701; PMID: 21307942
- Prasanth KV, Prasanth SG, Xuan Z, Hearn S, Freier SM, Bennett CF, et al. Regulating gene expression through RNA nuclear retention. Cell 2005; 123:249 - 63; http://dx.doi.org/10.1016/j.cell.2005.08.033; PMID: 16239143
- Hogg M, Paro S, Keegan LP, O’Connell MA. RNA editing by mammalian ADARs. Adv Genet 2011; 73:87 - 120; http://dx.doi.org/10.1016/B978-0-12-380860-8.00003-3; PMID: 21310295
- Chen LL, DeCerbo JN, Carmichael GG. Alu element-mediated gene silencing. EMBO J 2008; 27:1694 - 705; http://dx.doi.org/10.1038/emboj.2008.94; PMID: 18497743
- Kingston RE, Chen CA, Okayama H. Calcium phosphate transfection. Curr Protoc Immunol 2001; Chapter 10:Unit 10 3.
- Khabar KS. The AU-rich transcriptome: more than interferons and cytokines, and its role in disease. J Interferon Cytokine Res 2005; 25:1 - 10; http://dx.doi.org/10.1089/jir.2005.25.1; PMID: 15684617
- Khabar KS, Bakheet T, Williams BR. AU-rich transient response transcripts in the human genome: expressed sequence tag clustering and gene discovery approach. Genomics 2005; 85:165 - 75; http://dx.doi.org/10.1016/j.ygeno.2004.10.004; PMID: 15676275
- Mazumder B, Seshadri V, Imataka H, Sonenberg N, Fox PL. Translational silencing of ceruloplasmin requires the essential elements of mRNA circularization: poly(A) tail, poly(A)-binding protein, and eukaryotic translation initiation factor 4G. Mol Cell Biol 2001; 21:6440 - 9; http://dx.doi.org/10.1128/MCB.21.19.6440-6449.2001; PMID: 11533233
- Chartrand P, Meng XH, Singer RH, Long RM. Structural elements required for the localization of ASH1 mRNA and of a green fluorescent protein reporter particle in vivo. Curr Biol 1999; 9:333 - 6; http://dx.doi.org/10.1016/S0960-9822(99)80144-4; PMID: 10209102
- Casey JL, Hentze MW, Koeller DM, Caughman SW, Rouault TA, Klausner RD, et al. Iron-responsive elements: regulatory RNA sequences that control mRNA levels and translation. Science 1988; 240:924 - 8; http://dx.doi.org/10.1126/science.2452485; PMID: 2452485
- Tanguay RL, Gallie DR. Translational efficiency is regulated by the length of the 3′ untranslated region. Mol Cell Biol 1996; 16:146 - 56; PMID: 8524291
- Balmer LA, Beveridge DJ, Jazayeri JA, Thomson AM, Walker CE, Leedman PJ. Identification of a novel AU-Rich element in the 3′ untranslated region of epidermal growth factor receptor mRNA that is the target for regulated RNA-binding proteins. Mol Cell Biol 2001; 21:2070 - 84; http://dx.doi.org/10.1128/MCB.21.6.2070-2084.2001; PMID: 11238942
- Yang Q, McDermott PJ, Duzic E, Pleij CW, Sherlock JD, Lanier SM. The 3′-untranslated region of the alpha2C-adrenergic receptor mRNA impedes translation of the receptor message. J Biol Chem 1997; 272:15466 - 73; http://dx.doi.org/10.1074/jbc.272.24.15466; PMID: 9182579
- Mazroui R, Huot ME, Tremblay S, Filion C, Labelle Y, Khandjian EW. Trapping of messenger RNA by Fragile X Mental Retardation protein into cytoplasmic granules induces translation repression. Hum Mol Genet 2002; 11:3007 - 17; http://dx.doi.org/10.1093/hmg/11.24.3007; PMID: 12417522
- Krichevsky AM, Kosik KS. Neuronal RNA granules: a link between RNA localization and stimulation-dependent translation. Neuron 2001; 32:683 - 96; http://dx.doi.org/10.1016/S0896-6273(01)00508-6; PMID: 11719208
- Hu S, Claud EC, Musch MW, Chang EB. Stress granule formation mediates the inhibition of colonic Hsp70 translation by interferon-gamma and tumor necrosis factor-alpha. Am J Physiol Gastrointest Liver Physiol 2010; 298:G481 - 92; http://dx.doi.org/10.1152/ajpgi.00234.2009; PMID: 20110459
- Carbonaro M, O’Brate A, Giannakakou P. Microtubule disruption targets HIF-1alpha mRNA to cytoplasmic P-bodies for translational repression. J Cell Biol 2011; 192:83 - 99; http://dx.doi.org/10.1083/jcb.201004145; PMID: 21220510
- Bass BL. RNA editing and hypermutation by adenosine deamination. Trends Biochem Sci 1997; 22:157 - 62; http://dx.doi.org/10.1016/S0968-0004(97)01035-9; PMID: 9175473
- Lehmann KA, Bass BL. The importance of internal loops within RNA substrates of ADAR1. J Mol Biol 1999; 291:1 - 13; http://dx.doi.org/10.1006/jmbi.1999.2914; PMID: 10438602
- Borchert GM, Gilmore BL, Spengler RM, Xing Y, Lanier W, Bhattacharya D, et al. Adenosine deamination in human transcripts generates novel microRNA binding sites. Hum Mol Genet 2009; 18:4801 - 7; http://dx.doi.org/10.1093/hmg/ddp443; PMID: 19776031
- Lev-Maor G, Sorek R, Levanon EY, Paz N, Eisenberg E, Ast G. RNA-editing-mediated exon evolution. Genome Biol 2007; 8:R29; http://dx.doi.org/10.1186/gb-2007-8-2-r29; PMID: 17326827
- Anderson P, Kedersha N. RNA granules. J Cell Biol 2006; 172:803 - 8; http://dx.doi.org/10.1083/jcb.200512082; PMID: 16520386
- Kimball SR, Horetsky RL, Ron D, Jefferson LS, Harding HP. Mammalian stress granules represent sites of accumulation of stalled translation initiation complexes. Am J Physiol Cell Physiol 2003; 284:C273 - 84; PMID: 12388085
- Mollet S, Cougot N, Wilczynska A, Dautry F, Kress M, Bertrand E, et al. Translationally repressed mRNA transiently cycles through stress granules during stress. Mol Biol Cell 2008; 19:4469 - 79; http://dx.doi.org/10.1091/mbc.E08-05-0499; PMID: 18632980
- Stoecklin G, Stubbs T, Kedersha N, Wax S, Rigby WF, Blackwell TK, et al. MK2-induced tristetraprolin:14-3-3 complexes prevent stress granule association and ARE-mRNA decay. EMBO J 2004; 23:1313 - 24; http://dx.doi.org/10.1038/sj.emboj.7600163; PMID: 15014438
- Kedersha N, Anderson P. Stress granules: sites of mRNA triage that regulate mRNA stability and translatability. Biochem Soc Trans 2002; 30:963 - 9; http://dx.doi.org/10.1042/BST0300963; PMID: 12440955
- Wilczynska A, Aigueperse C, Kress M, Dautry F, Weil D. The translational regulator CPEB1 provides a link between dcp1 bodies and stress granules. J Cell Sci 2005; 118:981 - 92; http://dx.doi.org/10.1242/jcs.01692; PMID: 15731006
- Yang WH, Yu JH, Gulick T, Bloch KD, Bloch DB. RNA-associated protein 55 (RAP55) localizes to mRNA processing bodies and stress granules. RNA 2006; 12:547 - 54; http://dx.doi.org/10.1261/rna.2302706; PMID: 16484376
- Yang WH, Bloch DB. Probing the mRNA processing body using protein macroarrays and “autoantigenomics”. RNA 2007; 13:704 - 12; http://dx.doi.org/10.1261/rna.411907; PMID: 17339575
- Kedersha NL, Gupta M, Li W, Miller I, Anderson P. RNA-binding proteins TIA-1 and TIAR link the phosphorylation of eIF-2 alpha to the assembly of mammalian stress granules. J Cell Biol 1999; 147:1431 - 42; http://dx.doi.org/10.1083/jcb.147.7.1431; PMID: 10613902
- Scadden AD. Inosine-containing dsRNA binds a stress-granule-like complex and downregulates gene expression in trans. Mol Cell 2007; 28:491 - 500; http://dx.doi.org/10.1016/j.molcel.2007.09.005; PMID: 17996712
- Pare JM, Lopez-Orozco J, Hobman TC. Live cell imaging of Argonaute proteins in mammalian cells. Methods Mol Biol 2011; 725:161 - 72; http://dx.doi.org/10.1007/978-1-61779-046-1_11; PMID: 21528453
- Chaumeil J, Augui S, Chow JC, Heard E. Combined immunofluorescence, RNA fluorescent in situ hybridization, and DNA fluorescent in situ hybridization to study chromatin changes, transcriptional activity, nuclear organization, and X-chromosome inactivation. Methods Mol Biol 2008; 463:297 - 308; http://dx.doi.org/10.1007/978-1-59745-406-3_18; PMID: 18951174