Abstract
A veritable explosion of primary research papers within the past 10 years focuses on nucleolar and ribosomal stress, and for good reason: with ribosome biosynthesis consuming ~80% of a cell’s energy, nearly all metabolic and signaling pathways lead ultimately to or from the nucleolus. We begin by describing p53 activation upon nucleolar stress resulting in cell cycle arrest or apoptosis. The significance of this mechanism cannot be understated, as oncologists are now inducing nucleolar stress strategically in cancer cells as a potential anti-cancer therapy. We also summarize the human ribosomopathies, syndromes in which ribosome biogenesis or function are impaired leading to birth defects or bone narrow failures; the perplexing problem in the ribosomopathies is why only certain cells are affected despite the fact that the causative mutation is systemic. We then describe p53-independent nucleolar stress, first in yeast which lacks p53, and then in other model metazoans that lack MDM2, the critical E3 ubiquitin ligase that normally inactivates p53. Do these presumably ancient p53-independent nucleolar stress pathways remain latent in human cells? If they still exist, can we use them to target >50% of known human cancers that lack functional p53?
Introduction
Advances over the past two decades have revealed multiple functions for the eukaryotic nucleolus.Citation1,Citation2 Beyond its primary function in ribosome biosynthesis, the nucleolus initiates assembly of the signal recognition particle and processes several other small RNAs such as tRNAs, the telomerase RNA, the U6 snRNA, and the RNase P RNA. Regulatory proteins that participate in genome maintenance, telomere replication, and cell cycle progression associate dynamically with nucleoli. The nucleolus also acts as a principal stress sensor within mammalian cells by regulating murine double minute 2 (MDM2), the E3 ubiquitin ligase that negatively regulates p53, a critical tumor suppressor protein in mammalian cells.Citation3 In doing so, the nucleolus regulates mammalian cell cycle progression, senescence, and apoptosis. Obviously, these cell states have profound impacts on cell growth and organism development.
“Nucleolar stress” is the term now used to describe failures in ribosome biogenesis or function that ultimately leads to disruptions in cell homeostasis. Over the past 10 years we have seen a veritable explosion of papers describing nucleolar stress. Several recent reviews collectively cover nucleolar stress, and we are indebted to their authors.Citation4-Citation12 While nucleolar stress leading to p53-dependent cell cycle arrest has captured our full attention, several recent reports describe nucleolar stress leading to p53-independent response pathways that also lead to cell cycle arrest or apoptosis. Since >50% of human cancers lack functional p53, understanding these p53-independent pathways could potentially reveal additional cancer therapies that utilize induced nucleolar stress. Our aim here is to compare and contrast p53-dependent and p53-independent nucleolar stress pathways primarily in metazoan systems but with glimpses of ribosome and nucleolar stress in yeast for perspective.
Overview of Nucleolar Biology
Comprehensive reviews on nucleolar biology have been provided by Busch and Smetana,Citation13 Jordan and Cullis,Citation14 Hadjiolov,Citation15 and Olson.Citation3,Citation16 We know from the pioneering work of Perry,Citation17,Citation18 Edström et al.,Citation19 and Warner and SoeiroCitation20 that nucleoli are responsible for ribosome biogenesis (), and from the work of Brown and Gurdon,Citation21 Ritossa et al.,Citation22,Citation23 and Birnstiel et al.Citation24 that chromosomal nucleolar organizers first described by HeitzCitation25 and McClintockCitation26 contain tandemly repeated genes that encode pre-rRNA. Indeed, our best direct visualization of gene transcription comes from the electron microscopic spreads of rRNA genes.Citation27 Detailed mechanistic models now describe the tandem rDNA transcription units, recruitment of RNA polymerase I (Pol I) to rDNA promoters by various transcription factors, and cell cycle-dependent regulation of Pol I transcription which indirectly regulates nucleolar disassembly during prophase and re-assembly (nucleologenesis) beginning in telophase.Citation28,Citation29
Figure 1. Ribosome biogenesis in eukaryotic cells occurs in the nucleolus, a nuclear compartment that displays the fibrillar center (FC), the dense fibrillar component (DFC), and the granular component (GC) at the ultra-structural level. Transcription of pre-rRNA (47S in mammals) by RNA polymerase I (Pol I) is generally thought to occur at the boarders of the FC and DFC on tandem gene repeats emanating as radial loops from more condensed rDNA within the FCs. The 5S rRNA is transcribed by RNA Pol III in all eukaryotes; in yeast the 5S genes reside within the nucleolus within intergenic sequences that lie between the larger tandem rDNA gene repeats, but in metazoans, the 5S genes reside as tandem repeats outside the nucleolus. Processing and cleavage of the 47S pre-rRNA occurs in the DFC as ribosomal proteins synthesized in the cytoplasm enter the nucleolus and assemble with the 18S rRNA to form the small ribosomal subunit (SSU) and with the 5.8S, 28S, and 5S rRNAs to form the large ribosomal subunit (LSU). Large and small ribosomal subunits continue to assemble and mature as they enter the GC which contains GTPases (e.g., GNL3) that likely function in subunit maturation or nucleolar release. The GC is drawn here as a heterogeneous nucleolar compartment consisting of RNA-containing and RNA-deficient zones. Another GTPase, Nucleostemin (NS), resides in the RNA-deficient zones of the GC.Citation50 Rather than participating in ribosome biogenesis directly, NS functions in maintaining stem cell homeostasis and in responding to nucleolar stress.Citation82 Export of the ribosomal subunits is finally mediated by nuclear export factors, NMD3 and CRM1. See text for more details.
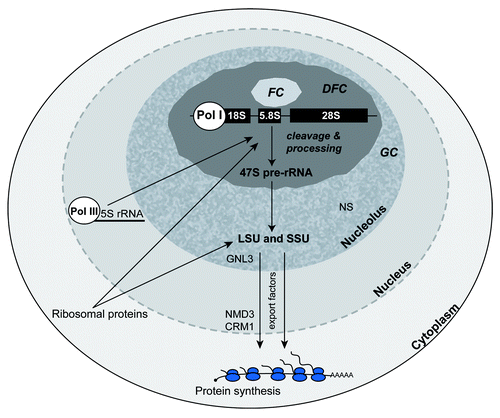
By transmission electron microscopy, typical nucleoli contain three morphologically distinct sub-compartments (): the fibrillar center (FC), the dense fibrillar component (DFC), and the peripheral granular component (GC). Transcription of pre-rRNA occurs on the boarders between the FC and DFC, while pre-rRNA processing and initial ribosome subunit assembly occur largely within the DFC. In mammals, Pol I transcription produces a 47S pre-rRNA transcript (35S in yeast) which is cleaved (processed) to generate 18S, 5.8S, and 28S rRNAs. Genes encoding the 5S rRNA are transcribed by Pol III; in metazoans the 5S genes are tandem repeats lying outside the nucleolus. In yeast, the 5S genes reside within intergenic regions that separate individual nucleolar rDNA transcription units.Citation30
Nucleolar Stress Effectors
Several ribosome assembly factors maintain their greatest steady-state concentrations within nucleoli under normal growth conditions, but assume altered functions to act as effectors under nucleolar stress conditions. In this section we introduce these factors by describing their normal nucleolar functions. In the next section we describe their altered roles during nucleolar stress. Besides these nucleolar assembly factors, the ribosomal proteins themselves act as stress effectors when they fail to assemble into small and large ribosomal subunits (in this case referred to as ribosomal stress).
Pol I transcription of pre-rRNA
The Pol I machinery has been well described in yeast and vertebrate systems.Citation31-Citation33 For instance, the productive Pol I transcription initiation complex in mouse includes the upstream binding factor UBF, the multi-protein complex TIF-IB (called selectivity factor SL-1 in humans), TIF-IA, the Pol I complex itself, and several other factors that regulate Pol I transcription, in particular SIRT7 that deacetylates the Pol I subunit PAF53 under normal non-stress conditions to ensure occupancy of Pol I with the rDNA.Citation34 UBF dimers bind the core promoter element that spans the transcription start site; they also bind the upstream control element (UCE) to perform several roles.Citation35 Once bound, UBF participates in opening the promoter region and in recruiting TIF-IB and Pol I complexes to the promoter core. UBF may also participate in the release of Pol I from the promoter at transcription initiation and in transcription elongation as UBF also decorates the length of the rDNA transcription units.Citation36
Of the Pol I transcription factors, TIF-IA is likely the central homeostatic factor in activating Pol I transcription. Under normal growth conditions, TIF-IA is critical in establishing productive Pol I transcription initiation complexes, due in part to its growth factor-dependent phosphorylation by ERK, or by its nutrient-dependent mTOR signaling and activation.Citation37,Citation38 However, upon oxidative stress (H2O2) or ribotoxic stress with anisomycin, activated c-Jun N-terminal kinase 2 (JNK2) phosphorylates Thr200 in mammalian TIF-IA, and this not only abrogates TIF-IA’s interactions with both Pol I and TIF-IB, but redistributes TIF-IA to the nucleoplasm. Thus, pre-initiation complex assembly fails under these particular conditions leading to nucleolar stress.Citation39,Citation40
Pre-rRNA processing
Pre-rRNA processing and ribosome assembly occur within complex yet highly coordinated reactions and interactions within the 90S processome of the nucleolar DFC.Citation41 In general, we know the major pre-rRNA processing events: box C/D and box H/ACA small nucleolar ribonucleoprotein particles (snoRNPs) catalyze nucleotide-specific 2’-O-ribose methylation and pseudouridylation, respectively.Citation42 Concomitantly, pre-rRNA undergoes endo-nucleolytic cleavage reactions to generate mature 18S, 5.8S, and 28S rRNAs as nascent ribosomal proteins arrive from the cytoplasm to assemble with these rRNA ().
Approximately 250 non-ribosomal nucleolar proteins act as ribosome assembly factors; specifically, they function as nucleases, helicases or chaperones to mediate the many processing and assembly events. Many of these nucleolar proteins are conserved throughout the eukaryotes. For instance, fibrillarin is the well conserved methyl-transferase associated with box C/D snoRNPs, while dyskerin (Cbf5 in yeast) is the pseudouridylase within box H/ACA snoRNPs. While fibrillarin and dyskerin appear to be limited to their sole tasks, nucleophosmin and nucleolin are multi-functional DFC proteins. Nucleophosmin 1 (NPM1, also called B23, numatrin in mammals, or NO38 in amphibians) binds nucleic acids, displays RNase activity, and participates in cleaving of the second internal transcribed spacer within pre-rRNA, clearly making it a ribosome assembly factor. When overexpressed, NPM1 binds p53, suggesting a role in modulating p53’s abundance and function (see detailed discussion below).
Nucleolin contains four RNA recognition motifs (RRMs) followed by a carboxyl tail rich in Arg-Gly-Gly tri-peptide motifs. These RNA-binding motifs allow nucleolin to bind defined stem-loop structures within the pre-rRNA, perhaps acting as a chaperone for proper RNA folding in ribosome assembly.Citation43 Nucleolin, however, also contains an N-terminal domain consisting of alternating acidic and basic motifs. The acidic motifs are rich in phospho-serine, while the basic domains resemble the tails of histone H1, especially with its multiple CDK1/Cyclin B phosphorylation sites. Studies indicate that nucleolin associates with nucleolar chromatin and participates in Pol I transcription, perhaps linking Pol I transcription with pre-rRNA processing in a regulatory feedback mechanism.Citation44-Citation46 Similar to NPM1, nucleolin interacts with several proteins,Citation47 including p53.Citation48 Takagi et al.Citation49 showed that when overexpressed, nucleolin binds the 5′ UTR of the p53 transcript to suppress its translation; conversely, downregulation of nucleolin promotes p53 expression.
Ribosome assembly
During the course of ribosome assembly, equimolar amounts of ribosomal proteins are translated in the cytoplasm and imported into the nucleus. The 18S rRNA assembles with 33 proteins to form the small 40S ribosomal subunit (SSU in ), while the 5.8S, 28S, and Pol III-transcribed 5S rRNAs assemble with 50 proteins to form the large 60S ribosomal subunit (LSU in ). Ribosomal proteins comprising the small subunit are designated RpS1, RpS2, etc., while large subunit proteins are designated RpL1, RpL2, etc. Important for discussions on nucleolar stress are RpS3, RpS7, RpL5, RpL11, RpL23, and RpL26.
Immature 40S and 60S subunits emerge from the DFC to occupy specified sub-compartments within the GCsCitation50 (see and below). Subunit export to the cytoplasm is mediated by the adaptor protein NMD3 and the export factor CRM1.Citation51 We continue to discern how the ribosomal subunits achieve functional maturation within the cytoplasm.Citation52-Citation55 Cryo-EM and crystallographic structures of eukaryotic ribosomes provide opportunities to fully comprehend not only ribosome function during translation initiation, elongation, and termination, but emerging inter-relationships between ribosome biogenesis and/or function and cell homeostasis; that is, how cell homeostasis is lost when individual ribosomal proteins are mutated or deleted (the ribosomopathies).Citation56-Citation62 As discussed below, these investigations should allow us to select strategically nucleolar or ribosomal targets for novel anti-cancer therapeutics.
p53-Dependent Nucleolar Stress
In their landmark paper, Rubbi and MilnerCitation63 employed UV irradiation to induce DNA damage to disrupt nucleoli, which in turn resulted in p53 activation and cell cycle arrest. Links between double strand chromosomal breaks, activation of ATM, and the transient block of Pol I initiation complex assembly and in transcription elongation were subsequently established.Citation64,Citation65 Rubbi and MilnerCitation63 could bypass the UV-induced stimulation of nucleolar stress by injecting an antibody against Upstream Binding Factor (UBF), the Pol I transcription and/or nucleolar chromatin factor. Thus, by blocking Pol I transcription selectively, they were again able to induce nucleolar disruption leading to p53 activation, but now without DNA damage. They concluded that the nucleolus is a major stress sensor which when disrupted, initiates p53-dependent cell cycle arrest. The principal mechanism that links nucleolar disruption with p53 activation and mammalian cell cycle arrest utilizes MDM2 (murine and/or human double minute 2), the ubiquitin E3 ligase that negatively regulates p53 by marking it for ubiquitin-mediated proteasomal degradation (see ).Citation66
Figure 2. Regulation of p53 during normal and nucleolar stress conditions. (A) During normal, non-stressed conditions, the E3 ubiquitin ligase MDM2 associates with p53, promoting p53’s degradation.Citation66 Nucleophosmin (NPM) and ARF are located in the nucleolus.Citation78 (B) During nucleolar stress, normal ribosome biogenesis and function are perturbed. The association between MDM2 and p53 is disrupted; additional proteins such as ribosomal proteins (RpL5, RpL11) with the 5S rRNACitation115,Citation116 and Arf can associate with MDM2.Citation69 p53 is stabilized and activates the cell cycle inhibitor p21 and other p53-responsive genes. These events lead to cell cycle arrest and apoptosis.
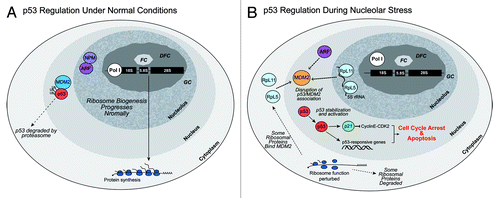
Nucleolar factors that block MDM2
Upon nucleolar stress, several ribosome assembly factors that normally enrich within nucleoli redistribute to the nucleoplasm, while ribosomal proteins entering the nucleus (nucleoplasm) are incapable of assembling into ribosomes. Several of these assembly factors and ribosomal proteins bind to and block MDM2 activity resulting in p53 stabilization. Even the 5S rRNA is now known to help trigger the activation of p53 by inactivating MDM2 (). shows where various factors bind MDM2 to inhibit its activity; the N-terminal domain of MDM2 binds p53, preventing p53 from inducing transcription of downstream effector genes (e.g., p21). The carboxy RING finger domain of MDM2 is the E3 ligase responsible for ubiquitinylation of p53, marking it for proteasomal destruction. The central acidic domain of MDM2 contains a C4 zinc finger, and it likely folds such that the N-terminal domain of MDM2 with its bound p53 now lies in close juxtaposition with the carboxy E3 ligase. Preventing the central domain of MDM2 from folding would likely interfere with MDM2-mediated p53 degradation.
Figure 3. MDM2 and p53 Regulation. MDM2 has three main functional domains: a N-terminal p53-binding domain, a central acidic region, and a carboxy RING finger, which has E3 ubiquitin ligase activity.Citation72 (A) MDM2 folds such that the carboxy RING finger domain interacts with p53 bound at MDM2’s N-terminus. p53 is then ubiquitinylated and targeted for degradation.Citation66,Citation70 (B) Protein folding is prevented when certain proteins such as ARF, Nucleostemin (NS), or other ribosomal proteins bind to the phosphorylated central domain of MDM2. This results in stabilization and accumulation of p53 levels.Citation100,Citation103
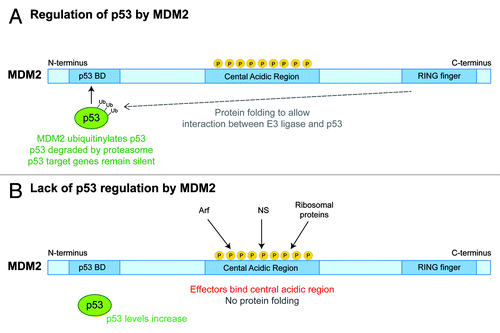
Arf (alternative reading frame protein, p19Arf in mouse, p14Arf in humans) is a tumor suppressor protein expressed from the p16INK4a gene locus that also expresses p16INKa, a cyclin-dependent kinase (CDK) inhibitor. The locus expresses both proteins by alternative utilization of exons and reading frames.Citation67 Arf normally localizes to nucleoli in non-stressed cells, most likely due to its interaction with nucleophosmin (see below). While exogenous Arf can sequester MDM2 to nucleoli (), subsequent studies indicate that nucleolar stress releases Arf to the nucleoplasm where it binds and blocks MDM2,Citation68,Citation69 thus allowing p53 to accumulate and block cell cycle progression (). When released from nucleoli, Arf binds the central acidic region of MDM2 to inhibit its ubiquitinylation of p53.Citation70-Citation74
Nucleophosmin 1 (NPM1) is an abundant nucleolar protein with multiple, complex roles. As mentioned above, it acts as a nucleolar endoribonuclease required for ITS2 cleavage in pre-rRNA processing,Citation75 and it functions as a key regulator in nucleolar control of cell homeostasis. For example, NPM1 shuttles between the nucleolus and the nucleoplasm, and in so doing it may regulate transcription by interacting with factors such as Myc, NFĸB, YY1, p53, and others. NPM1 is a histone chaperone that helps maintain genome stability by participating in DNA double-strand break repair, blocks apoptosis when overexpressed, and participates in centrosome duplication at prophase and mitotic spindle pole formation at metaphase.Citation76,Citation77 NPM1 is also a critical regulator in the nucleolar stress response. Specifically, NPM1 interacts with the N-terminus of Arf; one interpretation suggests that upregulation of Arf sequesters NPM1 to the nucleolus thus blocking NPM1’s shuttling to the nucleoplasm ().Citation78 MDM2 also binds the N-terminus of Arf antagonizing the interaction between Arf and NPM1, and thereby reversing the inhibitory effects of Arf on NPM1. Itahana et al.Citation79 further suggested that exogenously expressed Arf negatively regulates NPM1 by promoting the poly-ubiquitinylation of NPM1, leading to its destruction and a block in ribosome biogenesis.
A more recent interpretation, however, suggests that under normal growth conditions, the abundance of NPM1 exceeds that of Arf, and as a consequence, most Arf protein is bound to NPM1 within the nucleolus.Citation80,Citation81 Sequestering Arf to the nucleolus would prevent its association with MDM2 in the nucleoplasm.Citation80 The interaction with NPM1 in the nucleolus would also stabilize Arf which rapidly degrades in the absence of NPM1.
Nucleostemin (NS) is another nucleolar regulator of p53, but it modulates p53 indirectly by binding MDM2.Citation82 Mammalian NS consists of an N-terminal basic domain followed by a coiled-coil domain, a central GTP-binding domain, an RNA-binding domain, and finally a carboxyl acidic domain. NS belongs to the YawG subfamily of GTPases with its central GTP-binding motifs arranged in permutated fashion (G5-G4-G1-G2-G3) rather than in the conventional G1-G2-G3-G4-G5 as found in Ras-family GTPases. NS is abundant in stem cells and cancer cells, but its abundance drops in stem cells as they enter differentiation pathways where cycle progression (proliferation) slows down.Citation83 NS localizes to nucleoli with GTP bound, but it transits to the nucleoplasm with GDP bound.Citation84,Citation85 Cycling between the nucleolus and the nucleoplasm likely imparts separate functions for NS.Citation86 Interestingly, mammalian NS occupies sub-compartments within the GC that are actually deficient in RNA (the lighter gray areas within the GC of ).Citation50 This suggests that the RNA-containing compartments of the GC function in ribosomal subunit maturation and release, while RNA-deficient compartments within the GC are reserved for maintaining cell homeostasis. As discussed below, mammalian NS plays a critical role in nucleolar stress response by interacting with p53.Citation82
Determining the precise function(s) of mammalian NS, however, has been challenging. Romanova et al.Citation87 showed that NS forms a large complex that co-fractionates with the pre-60S subunit. The complex contains processing factors Pes1, DDX21, EBP2, and several ribosomal proteins. Prolonged depletion of NS inhibited the processing of the pre-rRNA 32S intermediate to the mature 28S rRNA, while overexpression of NS facilitated 32S processing. Because NS localizes to non-rRNA containing regions of the GC,Citation50 it may not be directly involved with ribosome biosynthesis or maturation. Outside the nucleolus, NS has been reported to protect DNA at telomeres by mediating the interaction between PML-IV and SUMOylated telomeric repeat factor 1 (TRF1) which then recruits RAD51 to the telomeres.Citation88 Furthermore, NS may protect the genome from double-strand breaks associated with DNA replication in actively dividing stem cells by interacting with (perhaps recruiting) RAD51 to the sites of damage.Citation89 Recently, Lin et al.Citation90 argued that the NS gene in mammals has evolved from an ancestral gene, GNL3, such that now the primary role of NS is to protect the genome (e.g., from S-phase DNA damage) while maintaining only a minor, perhaps even indirect role in ribosome biogenesis.
Other, paralogous NS-like family members have been implicated directly in ribosome biogenesis. For example, loss of Grn1 in S. pombe blocks 35S pre-rRNA processing while blocking the release of RpL25a.Citation91 Similarly, loss of NST-1 in C. elegans reduces 18S and 26S rRNA abundance.Citation92 Rosby et al.Citation93 depleted Drosophila NS1 (homologous to vertebrate GNL3LCitation82) by RNAi expression, and showed an inhibition in LSU release from nucleoli leading to autophagy in terminally differentiated polyploid cells, and loss of diploid progenitor island cells in the larval intestines.
Regulation of p53 by NS is likewise complex: both over-and under-expression of NS seems to activate p53 leading to cell cycle arrest.Citation55,Citation82 When overexpressed and therefore abundant in the nucleoplasm, NS uses its coiled-coil domain to bind the acidic domain of MDM2. This blocks MDM2’s E3 ubiquitin ligase activity, thus allowing p53 to accumulate ().Citation94 Conversely, siRNA depletion of NS causes p53-dependent cell cycle arrest.Citation94,Citation95 In this case, ribosome production is disrupted by the loss of NS, and unassembled RpL5 and RpL11 are thought to bind MDM2 to block its ubiquitinylation of p53 in the same nucleolar stress mechanism already described.Citation94 Thus, MDM2 activity is blocked either by NS itself when overexpressed, or by ribosomal proteins when ribosome production is disrupted by NS depletion.
Regulation of NS is made more complicated due to its interactions with other nucleolar and nuclear proteins. Ma and PedersonCitation95 showed that NS abundance was inversely related to the abundance of Arf, a negative regulator of MDM2 (described above). They showed that NS levels fell by 50% when Arf was expressed exogenously in U2OS osteosarcoma cells which normally lack Arf. The instability of NS was attributed to the rise in p53 levels, perhaps due to Arf blocking MDM2 activity. Conversely, NS levels rose when endogenous Arf was depleted in HeLa cells. NS function may also be regulated by mechanisms that were not immediately obvious (i.e., ubiquitinylation), while its stability is regulated by GTP levels in mechanisms that remain largely unknown.Citation55 For example, Lo et al.Citation96 showed that NS undergoes proteasomal degradation in the absence of GTP, but in an ubiquitin and MDM2-independent fashion.
Regulation of NS may involve nucleophosmin (NPM1). As described above, NPM1 is a multi-functional nucleolar processing/assembly factor implicated in centrosome duplication, cell proliferation, and both oncogenic and tumor-suppressor activities depending on p53 levels in particular cell types. Ma and PedersonCitation97 showed that in human U2OS osteosarcoma NS and NPM1 co-localize within the granular component of interphase nucleoli and to telophase pre-nucleolar bodies prior to nucleologenesis; they showed a direct interaction between NS and NPM1 by co-IP, yeast two-hybrid, and in vivo bimolecular fluorescence complementation.
Avitabile et al.Citation98 extended these studies to neonatal rat cardiomyocytes and cardiac progenitor cells, and therefore to heart disease. They showed that NS, NPM1, and Arf redistribute to the nucleoplasm upon doxorubicin-induced cardiotoxicity which causes nucleolar stress. Release of Arf correlated with increased p53 activity as measured by increased p21 gene expression and by induced DNA damage as measured by the TUNEL assay. Silencing of NPM1 in these cells caused nucleolar stress resulting in the redistribution of NS and Arf from nucleoli to the nucleoplasm, but depletion of NS did not redistribute NPM1. Interestingly, they showed a re-expression of NS and NPM1 in border zone cardiomyocytes after pathological injury (myocardial infarction) indicating that reintroduction of NS in adult myocardial cells may be an important aspect of healing after myocardial infarction. Hariharan and SussmanCitation99 review the role of the nucleolus and nucleolar proteins in cardiovascular pathophysiology, especially the nucleolus as a stress sensor in cardiac disease.
Ribosomal proteins
Besides nucleolar assembly factors, several ribosomal proteins are known to bind MDM2 to block its ubiquitinylation of p53 ().Citation100,Citation101 These include RpS3,Citation102 RpS7,Citation103,Citation104 RpL5,Citation105-Citation107 RpL11,Citation71,Citation108-Citation110 and RpL23.Citation111 Upon nucleolar stress (failure in ribosome biogenesis), rather than being released from the nucleolus, these ribosomal proteins likely bind MDM2 in the nucleoplasm as they first enter the nucleus from the cytoplasm. Like Arf, these ribosomal proteins bind to the acidic central domain of MDM2. The functional significance of MDM2’s central domain for normal ubiquitinylation of p53 is well established.Citation72 One hypothesis suggests that binding of ribosomal proteins and other nucleolar factors reduces the flexibility of MDM2’s central domain, preventing the N-terminal domain of MDM2 with its bound p53 from folding to form a close juxtaposition with its carboxy domain that displays E3 ubiquitin ligase activity.Citation100
Disruption of nucleoli may not be the only trigger that liberates ribosomal proteins that then block MDM2. Fumagalli et al.Citation110 conditionally depleted RpS6 and thus the 40S subunit in mouse hepatocytes, but under these conditions nucleoli remained intact and biogenesis of the 60S subunit continued. Interestingly, when the 40S subunit is depleted, extra amounts of RpL11 were produced by a selective recruitment of rpL11 mRNA to actively translating polysomes. The rpL11 mRNA contains a translation-repressive 5′ poly-pyrimidine tract (5′-TOP). This transcript and others with 5′-TOP sequences (those encoding RpS8, RpS16, RpL26) actually maintain translational activity upon loss of the 40S subunit, suggesting a de-repression of the 5′-TOP upon loss of the 40S subunit. Thus, when the 40S subunit is depleted by loss of RpS6 (or RpS7, RpS23), translation of 5′-TOP transcripts (e.g., rpL11) produces excess RpL11 which then blocks MDM2 to ultimately activate p53 and arrest the cell cycle. How de-repression of 5′-TOP transcripts is mediated by loss of the 40S remains an intriguing question.
While most ribosomal proteins degrade rapidly within proteasomes during nucleolar stress,Citation112-Citation114 RpL5 and RpL11 tend to accumulate selectively.Citation107 This is in contrast to other ribosomal proteins known to bind MDM2. L5 and L11 accumulate by continued de novo protein synthesis, and by mutual protection from proteasomal degradation. During nucleolar stress (actinomycin D-mediated inhibition of Pol I transcription), these two proteins co-localize with each other, p53, MDM2, and the promyelocytic leukemia (PML) protein within remnant nucleoli. Based upon their co-localizations, Bursać et al.Citation107 suggest the nucleoli serve as the staging site for RpL5 and RpL11 binding and inhibition of MDM2, resulting in p53 activation (). Two subsequent reports further showed that RpL5 and RpL11 interact with the 5S rRNA to form a trimeric RNP complex that then blocks MDM2 (HDM2).Citation115,Citation116 Sloan et al.Citation115 noted the accumulation of the trimeric L5/L11/5S rRNA complex within the nucleoplasm rather than in nucleoli as observed by Bursać et al.,Citation107 but this may be due to the different cell types used in the respective studies.
Drugs and Environmental Insults that Induce Nucleolar Stress
Blocking Pol I transcription
Nucleolar stress (often referred to as ribosomal stress) is any disruption in ribosome biogenesis. The term ribosomopathy describes phenotypes associated with non-functional ribosomes within the cytoplasm. Nucleolar stress can be induced at multiple steps within the nucleolus, from Pol I transcription initiation and elongation to early and late pre-rRNA processing (ribosome assembly), and eventually to ribosome maturation and eventual release from the GC. Perturbations in ribosome biogenesis can cause disruptions in nucleolar integrity which releases nucleolar and ribosomal proteins to the nucleoplasm where they take on secondary functions as stress response effectors.Citation63 Here we briefly look at the various methods that have been used to block ribosome biosynthesis to induce nucleolar stress, beginning with Pol I transcription.
RNA Pol I transcription can be blocked by several drugs leading to nucleolar disruption.Citation4,Citation117 Early on we knew that actinomycin D at low concentrations (<5 nM) intercalates preferentially within GC rich rDNA to block Pol I transcription elongation. Inhibition of Pol I by actinomycin D causes nucleolar segregation where the FC and DFC form a crescent shaped “cap” over a somewhat centralized GC.Citation29 Other intercalating agents known to disrupt nucleolar transcription include mitomycin C, mitoxantrone, and doxorubicin; the latter two block topoisomerase function. The alkylating and DNA-crosslinking agents, cisplatin and oxaliplatin also block Pol I transcription effectively, as does the anti-metabolic drug, methotrexate. All these drugs have been used as chemotherapeutic agents in various cancer treatments.Citation4,Citation117 Depending upon the drug, nucleoli could also fragment into smaller “spots” or into “necklaces” as observed by the redistribution of nucleolar markers such as fibrillarin.Citation117 Newer drugs currently in Phase I and II clinical trials include ellipticine which blocks Pol I transcription initiation,Citation118 CX-3543 which blocks Pol I elongation by disrupting nucleolin interactions with rDNA,Citation119 and CX-5461 which blocks Selectivity Factor-1 (SL-1) from binding rDNA promoters to thus prevent pre-initiation complex assembly.Citation120 Expectations in cancer therapy may be high regarding these latter two drugs as they have exceptional specificity for targeting transcription by Pol I vs. Pol II and Pol III.Citation4,Citation5
Drugs that block pre-rRNA processing and ribosome assembly
While the drugs described above block Pol I transcription, other drugs target pre-rRNA processing. Camptothecin, flavopiridol, and roscovitin block early pre-rRNA processing as measured by inhibitions in 47S pre-rRNA cleavage. MG-132, homoharringtonine, and 5-fluorouracil block later processing events as measured by loss of mature 18S and 28S despite the presence of the 32S pre-rRNA intermediate.Citation117 While 5-fluorouracil blocks thymidylate synthetase, it also incorporates into nascent RNA which blocks subsequent pseudo-uridylation and marks these RNAs for destruction by the exosome.Citation117
Environmental insults
Besides drugs, environmental insults such as heat shock, oxidative stress, hypoxia, and UV irradiation can induce nucleolar re-organization or disruption. Several reports show that heat shock disrupts nucleoli and redistributes nucleolar proteins.Citation121,Citation122 Interestingly, mRNAs can transit through the yeast nucleoli during heat shock.Citation122 Trypanosoma cruzi at the epimastigote stage (the life cycle stage at which the tail is anterior to the nucleus) also accumulates mRNAs within its nucleoli during severe heat shock.Citation123 Hypoxia and acidosis can also downregulate rDNA transcription via the hypoxia-inducible factor (HIF) and the von Hippel-Lindau tumor suppressor protein (VHL).Citation124 UV irradiation damages genomic DNA thus blocking RNA Pol II transcription, and this ultimately leads to nucleolar disruption. Hypotonic shock is also known to quickly and reversibly disrupt nucleoli,Citation125 while hypertonic shock disrupts related Cajal bodies.Citation126 As far as we know, however, the effects of osmotic shock on nucleolar stress pathways have not been described.
Blocking mTOR signaling
Pre-rRNA transcription by RNA polymerase I is the rate-limiting step in ribosome biogenesis.Citation28 As such, it is tightly regulated by growth factor signaling and nutrient availability (e.g., TOR signaling). The mammalian target of rapamycin (mTOR) kinase regulates Pol I transcription by positively controlling transcription factors TIF-1A, SL-1, and UBF.Citation32,Citation40 mTOR also regulates protein translation by phosphorylating 4E-BP and S6 kinase that then respectively regulate eIF4E for cap-dependent translation initiation and RpS6 which influences elongation factor 2 kinase.Citation127 Starvation or treatment with rapamycin downregulates mTOR in yeast and mammalian cells, and this leads to a significant reduction in nucleolar size and a block in rDNA transcription. Tsang et al.Citation128 showed that upon inhibiting mTOR in yeast, the histone deacetylase, Rpd3-Sin3, rapidly associated with rDNA to deacetylate K5 and K12 of histone H4 in a rDNA-specific manner. Consequently, Pol I redistributed from the nucleolus and rRNA transcription was blocked. Deleting the rpd3 gene or H4 hyper-acetylation mutants blocked these adverse effects on nucleolar structure and function caused by inhibiting mTOR.
Endogenous Mutations: The Ribosomopathies
In their comprehensive review, Narla and EbertCitation129 defined the ribosomopathies as a collection of human disorders with defined clinical phenotypes caused by impaired ribosome biogenesis and function. These disorders result from natural mutations in genes that encode ribosome assembly factors within nucleoli or the ribosomal proteins themselves. The mutations can lead to craniofacial malformations, skin lesions, or an absence of erythroid progenitor cells in the bone marrow leading to anemia. Why only certain tissues are affected remains an intriguing question.Citation130 Here we briefly describe the ribosomopathies and the nucleolar or ribosome stress associated with each, but keep in mind that these diseases may not result simply from a defect in protein synthesis. The possibility that unassembled mutant ribosomal proteins may exert toxic effects on non-translation events has not been completely ruled out.Citation12
Treacher Collins syndrome (TCS) is most frequently associated with mutations in Tcof1/treacle, an assembly factor within the dense fibrillar component of nucleoli. TCS presents as a collection of craniofacial malformations that arise during early development.Citation131,Citation132 Treacle is similar to Nopp140 in motif composition and likely function;Citation133,Citation134 both proteins are considered chaperones for nucleolar snoRNPs, however, treacle (and perhaps Nopp140) may also play a vital role in recruiting UBF and Pol I to the rDNA promoter.Citation135 Haplo-insufficiencies in treacle (Tcof+/−) cause a block in 2’-O-ribose methylation,Citation136 an overall loss of cytoplasmic ribosomes,Citation137 and most telling, a loss of specialized embryonic neural crest cells that ostensibly have a high demand for protein synthesis and thus functional ribosomes.Citation131 These cells die by apoptosis prior to their normal migration into branchial arches I and II that give rise to the craniofacial structures that are adversely affected in TCS. Interestingly, most other stem and progenitor cells in Tcof+/− embryos survive treacle insufficiencies, again indicating diverse ribosome requirements in different progenitor cell populations at defined points in embryonic development. Using a Trp53 gene deletion in mouse or pifithrin-α, a drug that specifically blocks p53, Jones et al.Citation137 blocked apoptosis in these Tcof+/− neural crest cells, thus preventing the cranial abnormalities and confirming p53-dependent nucleolar stress. While mutations in Tcof1 have been most often associated with TCS, haplo-insufficiencies in POLR1D or mutations in both alleles of POLR1C (POLR1D and POLR1C encode subunits for both RNA Pol I and III) have also been linked to TCS.Citation138 Therefore, in terms of nucleolar stress, haplo-insufficiencies in treacle or Pol I and Pol III result in the loss of ribosome production, and this leads to p53-dependent cell cycle arrest and apoptosis principally in the neural epithelium and neural crest. EllisCitation139 discusses the dissimilar phenotypes associated with the TCS vs. the hematopoietic failures observed in other ribosomopathies as described below. These phenotypic differences may result from errors in pre-rRNA transcription and early processing (i.e., mutations in Pol I and treacle for TCS) vs. mutations in individual genes encoding the ribosomal proteins themselves.
Dyskeratosis congenita (DC) is a human skin and hematopoietic disorder associated with mutations in the X-linked DKC1 gene which encodes dyskerin, the box H/ACA snoRNP enzyme responsible for pseudouridylation of targeted residues within non-coding RNAs (e.g., pre-rRNA, spliceosomal). Dyskerin also interacts with the human telomerase RNA component (TER) due to the H/ACA-like domain at the RNA’s 3′ end of the RNACitation140,Citation141 and is required for telomerase biogenesis.Citation142 Mutations in DKC1 are associated with premature aging, a propensity for certain cancers,Citation129 abnormalities in the skin and mucous membranes, and with bone marrow defects.Citation142 Hoyeraal-Hreidarrson syndrome is a severe form of DC with earlier onset, additional neurological disorders, and higher morbidity.Citation142 At the molecular level defects have been observed in rRNA processing and reduced telomere length,Citation143,Citation144 both of which could lead to losses of bone marrow stem cells.Citation145
Besides mutations in the X-linked DKC1 gene, less severe forms of DC are associated with autosomal mutations in the TERT gene that encodes telomerase reverse transcriptaseCitation146 or in the TERC gene that encodes the RNA component of telomerase.Citation147,Citation148 These mutations cause a haplo-insufficiency in functional telomerase leading to shortened telomeres. Using zebra fish, Pereboom et al.Citation149 showed that loss of Nop10, one of the other three proteins within box H/ACA snoRNPs besides dyskerin (the other being Nhp2), caused a failure in 18S rRNA processing, loss of 40S ribosomal subunits, and a loss of hematopoietic stem cells. Similar to the loss of treacle in embryonic neural crest cells, these hematopoietic cells in zebra fish could survive if they carried a loss-of-function p53 allele indicating a p53-dependent nucleolar stress. Interestingly, however, telomere length in these cells was not restored, suggesting that nucleolar stress rather than shortened telomeres was the principal defect in the zebra fish model of DKC when nucleolar pseudouridylase activity is impaired.
Thumati et al.,Citation142 however, expressed TERT and TER in fibroblasts from X-linked DC patients to restore stable telomere maintenance but still in the presence of the mutant dyskerin protein. These cells continued to show slight but reproducible depletions of pseudouridine in the rRNA, but compared with wild type cells that also expressed exogenous TERT and TER, there were no detectable differences in protein synthesis, internal ribosome entry site translation initiation, in tolerances to ionizing radiation, or in endoplasmic reticulum stress in these modified DC cells. Thumati et al.Citation142 proposed that DC etiology is based primarily on the loss of telomere maintenance rather than on the loss of ribosome function.
Diamond-Blackfan anemia (DBA) in humans is a diverse collection of clinical disorders resulting from mutations consistently observed in genes encoding 40S subunit ribosomal proteins RPS7, RPS10, RPS17, RPS19, RPS24, or RPS26, or in genes encoding 60S subunit ribosomal proteins RPL5, RPL11, RPL26, or RPL35A.Citation139,Citation150,Citation151 Most are missense or nonsense mutations, but deletions have also been observed.Citation152,Citation153 All appear to be haplo-insufficiency-type mutations meaning that the wild type allele cannot provide sufficient product to prevent the disorder. DBA phenotypes vary depending on the particular mutation. While DBA patients could display short stature, an increased risk of malignancies, craniofacial, thumb, urogenital, and heart defects,Citation129,Citation139 the principle defect is congenital bone marrow failure (erythroid precursor failure) leading to anemia, macrocytosis, and reticulocytopenia.Citation154 Loss of erythroid precursor cells occurs by apoptosisCitation155 due to p53 accumulation and activation.Citation156,Citation157 The zebra fish model for DBA similarly shows developmental defects and failures in erythropoiesis upon loss of RpL11 or RpS19.Citation158-Citation160 The developmental defects can be rescued by depleting p53, but the erythropoietic defects appear to be p53-independent.Citation161,Citation162 Interestingly, both the developmental defects and the erythroid defects in RpL35A-deficient zebra fish embryos could be improved by stimulating the mTOR pathway with either leucine or arginine treatments. This suggests that the efficiency in protein translation by a limited number of functional ribosomes may have been bolstered by the now hyper-activated mTOR pathway.
Related to these findings in zebra fish, Narla and EbertCitation129 suggested that loss of erythroid progenitors by apoptosis could occur by one of two mechanisms. In the first pathway, loss of the ribosomal proteins could induce nucleolar stress leading to p53-dependent apoptosis as described above. If this mechanism is correct, we have to assume that erythroid progenitors have a higher demand for functional ribosomes than most other stem and/or progenitor cells within the body. In the second pathway, insufficient ribosomes would cause an under-production of hemoglobin in these erythroid progenitor cells, leading to an accumulation of excess heme that then induces oxidative stress leading to apoptosis and loss of the erythroid progenitors.Citation139 This argues for both p53-dependent and independent nucleolar stress responses not only in the same organism, but perhaps within the same cell. We describe various other p53-independent nucleolar stress responses below.
The 5q- syndrome in humans is another disease associated with erythroid failure. A deletion on the long arm of human chromosome 5 eliminates several genes, but a systematic RNAi screen identified RPS14 as the critical gene associated with erythroid failure when haplo-insufficient.Citation163,Citation164 The loss of RPS14 increases the 32S/18S processing ratio in cells derived from patients, and ectopic expression of RPS14 can rescue these cells. Cells expressing RPS14 shRNAs recapitulated the syndrome as these cells were deficient in 40S subunits.Citation163
Schwachman-Diamond syndrome (SDS) is another failure in hematopoiesis, but it also presents exocrine pancreatic failures and increased risks to myelodysplasia and leukemia. The syndrome is associated with hypomorphic mutations in the SBDS (Schwachman-Bodian-Diamond Syndrome) gene.Citation165 The SBDS protein is well conserved from archaea to vertebrates, and is likely multi-functional.Citation166 SBDS co-enriches with the 60S subunit presumably in the cytoplasm; Finch et al.Citation167 report that mouse SBDS acts in the cytoplasm to release eIF6 from the large 60S subunit by stimulating the GTPase activity of elongation factor-like 1 (EFL1). Mechanistic similarities between SBDS and the bacterial ribosome recycling factor (RRF) support the role of SBDS in activating ribosomes for protein translation. Finch et al.Citation167 used a liver-specific deletion of exon 2 in mouse SBDS which uncoupled GTP hydrolysis by EFL1 from the release of eIF6. It is unknown if other SBDS mutations associated with SDS affect the same coupling mechanism.Citation166 SBDS also forms a protein complex with nucleophosmin presumably within nucleoli as determined by reciprocal co-immuno-precipitations.Citation169 While mutations in SBDS do not seem to affect nucleophosmin’s abundance or nucleolar localization, interaction between SBDS and nucleophosmin suggests that SBDS could play a role in ribosome biosynthesis. Indeed, bone marrow cells from SDS patients showed dysregulation in genes involved with pre-rRNA transcription and processing, as well as dysregulation in many of the genes encoding ribosomal proteins.Citation168
Cartilage hair hypoplasia (CHH) is an autosomal recessive, highly pleiotropic disease that displays short stature, hair and hematological defects, and abnormal cellular immunity.Citation129 CHH patients also have an increased propensity for non-Hodgkin lymphoma and basal cell carcinoma. The primary lesion in CHH lies in the RMRP geneCitation170 which encodes the RNA component within the mitochondrial RNA processing (MRP) complex. MRP is an endonuclease with several functions in eukaryotic cells; one function is to cleave the RNA primers at the origins of mitochondrial DNA replication, another is the 5′ maturation of the 5.8S rRNA in the nucleolus.Citation171,Citation172 The RNA component in MRP is transcribed by RNA Pol III; several mutations in the RNA have been linked with CHH.Citation170
Werner and Bloom’s syndromes
The Werner syndrome (WS) is a rare autosomal recessive mutation that causes premature aging in young adults (often called the adult progeria) and an increased propensity for cancer. The WS protein, WRN, is a member of the RecQ DNA helicase family. As a group, the RecQ helicases function in both the stress generated during DNA replication and in DNA repair that uses homologous recombination. WRN functions in genome stability with roles in DNA replication and repair, transcription, and in telomere maintenance. Several studies located WRN in the nucleolus and in other nuclear foci including telomeres.Citation173,Citation174 WRN redistributes from nucleoli to the nucleoplasm during nutrient starvation, actinomycin D-mediated inhibition of Pol I transcription, its post-translational acetylation, and upon downregulation of the deacetylase, SIRT1, thus indicating a tight regulation of WRN by an acetylation and/or deacetylation cycle. While its precise role in ribosome biogenesis remains unknown, WRN co-precipitates with one of the Pol I subunits, augments promoter clearance of Pol I, and associates with the few active rDNA genes in quiescent cells.Citation174,Citation175 Finally, Werner patient fibroblasts display enhanced rDNA methylation,Citation176 again suggesting transcription is impaired. Thus, the possibility that nucleolar stress could result from the loss of WRN function remains quite viable.
Bloom’s syndrome (BS) is another autosomal recessive disorder linked to defects in the DNA RecQ helicase referred to as BLM. Loss of BLM causes increased intra- and inter-chromosomal recombination events, and increased recombination between rDNA genes. Like WS, BS displays predispositions to cancer, but it is characterized specifically by growth inhibition (dwarfism), sensitivities to sun light, immunodeficiency, and infertility.Citation177 BLM localizes to promyelocytic leukemia nuclear bodies (PML-NBs) under normal conditions.Citation178 Grierson et al.Citation179 described the localization of BLM to nucleoli as well as PML-NBs; they showed that BLM could co-precipitate with the largest subunit of Pol I, RPA194, and that BLM facilitated RNA Pol I transcription of pre-rRNA perhaps by unwinding GC-rich rDNA. Within nucleoli, BLM interacts with topoisomerase I to modulate RNA:DNA hybrids and to relax excess supercoils in the rDNA upon Pol I transcription.Citation180
Tikoo et al.,Citation181 however, placed less emphasis on BLM function within nucleoli. In their functional model for BLM, mono-ubiquitinylated BLM located with RAP80 to nuclear PLM bodies under normal conditions. Upon replication stress or chromatin damage, however, BLM was poly-ubiquitinylated by the E3 ligase, RNF8. This allowed BLM to interact with the ubiquitin-interacting motifs of RAP80 now positioned at sites of replication stress or chromatin damage. Depletion of RNF8 caused disruption of the PML-NBs and the redistribution of BLM to nucleoli.
Diseases associated with the small ribosomal subunit (SSU) processome
Sondalle and BasergaCitation182 reviewed several human diseases associated with mutations in proteins associated with the SSU processome, and we briefly mention three of them here. The SSU processome is responsible for 18S rRNA maturation and assembly of the small ribosomal subunit (SSU). The processome contains the U3 snoRNA and about 70 proteins, many of which are referred to as U3 proteins (UTPs). A R565W mutation in hUTP4/Cirhin is implicated in North American Indian childhood cirrhosis (NAIC) which afflicts the Ojibway-Cree population. The R565W mutation in hUTP4/Cirhin likely interferes with its normal associations with NOL11, another SSU processome component, presumably impairing normal SSU biogenesis. Like the other ribosomopathies, NAIC afflicts primarily one tissue type, in this case the liver.
Mutations in the SSU processome protein, hUTP14c, are implicated in male infertility and ovarian cancer. hUTP14c is an intronless retrogene copy of hUTP14a. While hUTP14a is expressed ubiquitously, hUTP14c is expressed only in human testes and in ovaries of some, but not all women. The Y738X mutation in hUTP14c is implicated in male sterility, while expression of wild type hUTP14c in the ovary may destabilize p53, leading to increased incidence of tumor formation.Citation182
Mutations in the SSU processome protein, EMG1 (essential for mitotic growth 1), are implicated in Bowen-Conradi syndrome, which afflicts the Hutterite community living on the Great Plains of the US and Canada. EMG1 is a highly conserved pseudouridine (N1) methyl-transferase, but its role as a ribosome assembly factor likely takes precedence over its methylase activity.Citation182 Interestingly, EMG1 in yeast interacts with SSU processome protein, Utp30, to help recruit RpS19 to the small subunit; overexpression of RpS19 can rescue the growth defect associated with a mutant EMG1. As described above, RpS19 is mutated in DBA, thus Bowen-Conradi and DBA may be linked.Citation182
Neurodegenerative diseases
Linking neurodegenerative diseases to nucleolar stress is an emerging field with tremendous potential in revealing molecular pathologies and eventual therapeutic strategies.Citation9,Citation183,Citation184 Neurodegenarative diseases associated with nucleolar stress include Parkinson disease,Citation184 trinucleotide repeat (polyglutamine) disorders such as spinocerebellar ataxias and Huntington’s disease,Citation185,Citation186 Alzheimer disease,Citation187 and amyotrophic lateral sclerosis (ALS).Citation188 Each of these diseases has direct and/or associated links to altered nucleolar function.Citation184 Here we mention only a few examples. For instance, a conditional ablation of TIF-IA in Nestin-Cre transgenic mice offers an excellent model for the loss of nucleolar function in post-mitotic dopaminergic neurons leading to the slow loss of these neurons and pathologies reminiscent of Parkinson disease.Citation189 In addition, an L166P mutation in PARK7/DJ-1 is associated with early onset Parkinson disease. The mutation causes DJ-1 to mis-fold resulting in either proteasomal degradation of DJ-1 or its accumulation in cytoplasmic Lewy Bodies that are typical within brain neurons of Parkinson patients. Formation of the cytoplasmic aggregates correlates with the redistribution of the tumor necrosis factor (TNF) receptor associated protein (TTRAP) from the nucleolus to these cytoplasmic granules.Citation190 Huntington disease also presents problems for nucleoli. For example, the expanded CAG repeat region of the Huntingtin transcript physically interacts with nucleolin, the prominent ribosome assembly factor that also regulates rRNA transcription within nucleoli. Nucleolin is thus titrated away from UBF and the Pol I transcription machinery, finally resulting in a loss of pre-rRNA transcription.Citation185 We refer the reader to the recent review by Parlato and LissCitation184 for a comprehensive discussion of these various associations between these and other neurodegenerative diseases and nucleolar stress. The one common feature between these different neurodegenerative diseases seems to be nucleolar stress, with defined pathogenic phenotypes that could someday be targets for therapeutic treatments.
Ribosomopathies in non-mammalian metazoans
We already mentioned above several contributions from zebra fish to our understanding of ribosomopathies. The rDNA-deficient 0-nu mutation in Xenopus was instrumental in defining the nucleolus as the site for ribosome biogenesis.Citation21 Tadpoles homozygous for the mutation fail to synthesize 18S and 28S rRNA; they have only maternal rRNA provided by the fertilized oocyte, but without the ability to synthesize new ribosomes, they die in the early swimming stages some three days post fertilization.
As we begin to transition toward p53-independent forms of nucleolar stress (see below), we briefly describe ribosomopathies in C. elegans and Drosophila, both of which have functional p53, but lack MDM2. Lee et al.Citation191 recently showed that dao-5 in C. elegans encodes a Nopp140 homolog. A null mutation in dao-5 disrupts rRNA synthesis leading to delays in gonadogenesis and an increased incidence of apoptosis in the germ line. An intriguing study in C. elegans by Fuhrman et al.Citation192 showed that mutation in the conserved nucleolar protein, NOL-6, actually enhanced resistance to bacterial infection (e.g., the mutation enhanced innate immunity) by activating p53/CEP-1 and thus its target gene, SYM-1. This study linked the nucleolus and p53 to an ancient form of innate immunity, further establishing the nucleolus as a stress response organelle.
The list of ribosomopathies is far from complete if one considers the many Drosophila mutations known to disrupt ribosome biogenesis or function. Some of these mutations have been known for decades. For instance, deletions in rDNA transcription units referred to as bobbed (bb) mutations have been well studied since the discovery of bbCitation1 by Calvin Bridges in 1915.Citation193,Citation194 The vast majority of Minute genes encode ribosomal proteins; one encodes a translation initiation factor.Citation195,Citation196 Mutations in these genes lead to haplo-insufficient phenotypes of delayed development, shortened and thin bristles, and reduced viability and fertility. Other Drosophila genes known to encode nucleolar processing and/or assembly factors have also been characterized in terms of their mutant phenotype; those that have been better characterized include minifly,Citation197 modulo,Citation198-Citation200 viriato,Citation201 dribble,Citation202 jumeaux,Citation203 pitchoune,Citation204 and Nopp140.Citation205,Citation206 The minifly gene (also called Nop60B) encodes a pseudouridylase homologous to human DCK1. Mutations in minifly not only inhibit pseudouridylation of rRNA, but disrupt normal pre-rRNA cleavage patterns. This leads to reduced body size (thus the name) and to several other developmental abnormalities.Citation197,Citation207,Citation208 Modulo is a multi-functional, nucleolin-like protein in Drosophila that locates to nucleoli, but also to non-nucleolar chromatin where it can affect (modulate) gene expression by position effect variegation. Interestingly, modulo locates to nucleoli when phosphorylated, but to non-nucleolar chromatin when un-phosphorylated.Citation199 While its role in ribosome assembly remains unknown, nucleolar modulo also participates in centromere organization by interacting with the Chromosome Alignment 1 protein (CAL1), a centromere assembly factor required for the localization of the H3-variant, CENP-A (CID in Drosophila).Citation209 As in mammalian systems, nucleolar activity in Drosophila is regulated by Myc; Grewal et al.Citation210 demonstrated the necessary requirement of Myc in upregulating RNA Pol I transcription during Drosophila development. The modulo, viriato, pitchoune, and Nopp140 genes are also known targets for Myc in controlling proliferation and growth.Citation200,Citation201,Citation204
Clearly, many nucleolar processing and/or assembly factors are multi-functional. Comparative siRNA screens of 625 nucleolar proteins in HeLa cells identified 286 that are required for pre-rRNA processing. Of these, 27% have either distinct or additional functions in pre-rRNA processing as compared with their yeast orthologs.Citation211 Thorough understandings of intracellular and whole-organism phenotypes caused by null or partial-loss-of function mutations in genes encoding these factors are critical before devising strategies to induce nucleolar stress in potential cancer therapies (see below). Toward this end, Neumüller et al.Citation212 performed comparative genome-wide loss-of-function analyses of genes necessary for the regulation of nucleolar size (Pol I–mediated transcription) in both yeast and Drosophila. Their comparative approach defined an evolutionarily conserved network of genes required for cell growth. This gene network may provide new insights in limiting tumor cell growth.
p53-Independent Nucleolar Stress
Nucleolar stress in yeast
Ribosome biogenesis in yeast shares many commonalities with its metazoan counterpart.Citation213 Specifically the number of rRNA species, biogenesis factors and ribosomal proteins is roughly equivalent.Citation214,Citation215 However, with this high degree of process conservation there are some major differences in the yeast and mammalian response to stalled or aberrant ribosome biogenesis. As described above, the best studied pathway for the nucleolar stress response in mammals is mediated through a p53-dependent mechanism. However, yeast does not express a characterized p53 or MDM2.Citation216,Citation217 Therefore, the several described p53 dependent pathways do not apply to the yeast nucleolar stress response.Citation95,Citation97,Citation100,Citation218,Citation219 This observation can be interpreted in a few ways. First, nucleolar stress responses in yeast could be a mediated through a unique pathway that is not conserved in mammals, or second, yeast might have a conserved ancestral pathway that has been further enhanced in metazoans to address their complex cellular needs, and this enhanced pathway has become epistatic to the ancestral pathway. The current data support the latter scenario. Unfortunately, the stress response factors involved in yeast nucleolar stress are poorly understood.
In both yeast and mammalian models, cell cycle defects associated with aberrant ribosome biogenesis often precede bulk protein synthesis defects.Citation220-Citation227 As ribosome biogenesis is sensed at START,Citation221 we expect mutations that lead to defects in ribosome biosynthesis would also lead to cell cycle defects at the G1/S transition. In fact, this is the case for several ribosome biogenesis factors. However, there are examples where disruption of ribosome biogenesis leads to specific defects at other steps in the cell cycle.Citation228-Citation231 In a comprehensive study, Thapa et al.Citation228 systematically depleted ribosomal proteins in yeast and showed that perturbation of ribosome biogenesis manifests in several cell cycle and morphological defects. Interestingly, depletion of 22 of 26 small subunit R-proteins placed under Gal-regulation (S0–S6, S9–S11, S13–S15, S17, S19–S22, S26, S27, S29, and S30) resulted in a G1 arrest, while depletion of the remaining four (S8, S23, S24, and S31) showing no change in flow cytometry profiles. In contrast to depletion of small subunits proteins where a relatively uniform phenotype was observed, depletion of large subunit R-proteins that had been placed under Gal-regulation yielded varying phenotypes. Depletion of nine different large subunit ribosomal proteins (L1, L3, L9, L16, L19, L21, L25, L30, and L43) resulted in a G1 arrest, while 11 of the depletions (L2, L5, L8, L10, L13, L20, L23, L27, L32–L34) showed no change in their flow cytometry profiles. In addition, the authors noted that depletion of eight large subunit proteins (L3, L4, L7, L18, L28, L35, L37 and L40) resulted in a unique peak in which cells had greater than 2N DNA content. Using microscopy, they determined that the >2N peak was caused by chains of cells that were arrested in the G2/M and/or cytokinesis.Citation228 Importantly, the various phenotypes observed in this study highlight the complexity of ribosome biogenesis, and strongly suggest that in yeast, multiple pathways exist for nucleolar stress responses.
An even greater level of complexity has been observed for the nucleolar stress response to depletion of Nop7. The yeast Nop7 gene is required for processing the 27SA3 pre-rRNA in the formation of 27SBS intermediate and subsequently the mature 5.8S rRNA.Citation232 Nop7 is also required for export of the large ribosomal subunit.Citation232 Interestingly, two temperature sensitive alleles of the Nop7 gene arrest at different points in the cell cycle. When grown at the non-permissive temperature, cells harboring the Yph1–24 allele arrested at G1, while cells harboring the Yph1–45 allele arrested in G2.Citation233 This interesting result supports the hypothesis of multiple mechanisms for “sensing” ribosome biogenesis.
In budding yeast, the best described coordination between ribosome biogenesis and the cell cycle is through the Whi5 protein (the functional equivalent to the Retinoblastoma tumor suppressor protein in metazoans).Citation221,Citation234,Citation235 Under healthy growing conditions, Whi5 normally binds to SBF/MBF preventing pre-mature transcription of the G1/S regulon that comprises approximately 200 genes. Whi5 is displaced from SBF/MBF upon phosphorylation by Cln3/Cdc28 CDK, at which point SBF/MBF initiates transcription of the G1/S regulon.Citation236,Citation237 Using Gal::Pwp2 (90S pre-ribosomal component), Bernstein et al.Citation220,Citation221 demonstrated that depletion of this SSU processome component resulted in a G1 cell cycle arrest and an increased nuclear retention time for Whi5-GFP, consistent with its role in the G1 regulon repression. In a separate experiment, cells were depleted of Pwp2 within a wild type or a whi5 null background. In the wild type background, depletion of Pwp2 caused an accumulation of unbudded cells. In contrast, even after an extended Pwp2 depletion, the whi5 null cultures accumulated far fewer unbudded cells, indicating Whi5 plays a role in regulating the G1/S checkpoint upon depletion of a ribosome biogenesis factor, and that deletion of Whi5 delays the G1 accumulation that is normally observed with Pwp2 depletion.Citation220 Another interesting observation from this study was that upon Pwp2 depletion, the delayed cell cycle was independent of Cln3, a major Whi5 antagonist. Thus, in the absence of a functional Whi5, the critical cell size checkpoint at (START) is bypassed, allowing the cells to continue dividing in the absence of ribosome biogenesis.
In recent studies, Gomez-Herreros et al.Citation238,Citation239 established that upon stalled ribosome biogenesis, budding yeast accumulate free ribosomal proteins L5 and L11 which leads to a G1 cell cycle arrest. To induce the yeast G1 arrest, the authors treated cells with the nucleotide depleting drugs (NTDs) 6-azauracil (6AU) and mycophenolic acid (MPA) which disrupt transcription elongation by inhibiting inosine monophosphate dehydrogenase to thereby limit the synthesis of guanine nucleotide.Citation240,Citation241 In human cells, MPA has been used to stall rRNA synthesis which disrupts nucleoli leading to the release of the ribosomal proteins L11 and L5.Citation242 L5 and L11 bind to and inhibit the E3 ubiquitin ligase MDM2 and activate of p53 which ultimately leads to a p53 dependent G1 arrest.Citation218,Citation242 Interestingly, even in the absence of a p53 homolog, yeast cells treated with NTDs also arrested in G1. To establish the role of RpL5 and RpL11 in the observed G1 arrest, the authors used 6AU to stall pre-rRNA synthesis, and examined polysome profiles. Surprisingly, in 6AU treated cells there was an accumulation of the ribosomal proteins L11 and L5 within the “free” portion of the fractions. This experiment was repeated in an rrs1 null background. Because Rrs1 is required for assembly of L5 and L11 within the large ribosomal subunit, one would expect that upon stalling biogenesis in an rrs1 null background with an NTD there should be an enhanced cell cycle arrest phenotype. As expected, upon treating cells with 6AU in the rrs1 null background, the authors observed an enhanced G1 arrest as compared with when wild type cells were treated with 6AU. This result suggests a role for L5 and L11 in the yeast nucleolar stress response. Gomez-Herreros et al.Citation238 previously showed that the amount of free cellular L5 increases upon depletion of L11. Therefore, in a separate experiment, the authors repeated the treatment with 6AU while depleting L11, and saw an increase in the G1 accumulation compared with cells treated without depleting L11. This experiment suggested that upon depleting L11, the pool of free L5 was increased leading to a more severe growth arrest. In a contrasting experiment, the authors repeated the 6AU treatment in a dst1 null background because in the absence of Dst1, L5 mRNA transcription is hindered leading to a reduction in L5 protein. Surprisingly, in this experiment the G1 cell cycle arrest induced by 6AU was delayed by several hours.Citation239 This result again reiterates the importance for L5 and L11 in the yeast nucleolar stress response.
Considering the various phenotypes observed when yeast ribosome biogenesis is disrupted, it is intriguing that relatively few pathways have been described that lead to these phenotypes. The Whi5 mechanism for repression of the G1 regulon has been very well characterized,Citation220,Citation221,Citation234-Citation236 but the Whi5 mediated mechanism can only account for cell cycle arrest at G1/S; there may be different mechanisms to elicit G1 arrest when different ribosomal proteins and biogenesis factors are depleted. In addition, relatively little is known about G2/M-phase arrest upon depletion of yeast ribosome biogenesis factors. These factors may be more complex in terms of abundance and functional diversity as they may be required for mitosis. While nucleolar stress in yeast may be considered ancestral, it will likely be as complex as that described for metazoan model systems.
p53-Independent Nucleolar Stress in Metazoans
p53-independent cell cycle control
We begin this section by describing metazoan cell cycle arrest induced by nucleolar stress but in p53-independent manners. Key papers include Donati et al.,Citation243 Iadevaia et al.,Citation244 and Li et al.Citation245 Each example includes some disruption in ribosome biogenesis leading to nucleolar stress, involving E2F-1 downregulation or p27 Kip1 expression, all resulting in cell cycle arrest.
POLR1A encodes the catalytic subunit of RNA Pol I.Citation32 Expressing siRNA to deplete POLR1A function specifically and effectively reduces nucleolar rDNA transcription without disturbing ribosome components made by Pol II and Pol III.Citation243 Silencing POLR1A to inhibit rDNA transcription in U2OS osteosarcoma and HCT-116 colon cancer cells lines (both are p53+/+) resulted in p53 stabilization, increased expression of the cell cycle inhibitor p21, and a decrease in phosphorylated retinoblastoma protein (pRb). As expected, these events led to an accumulation of cells in G1 phase and a significant reduction in S-phase cells as measured by BrdU incorporation.Citation243 To investigate the link between p53 and pRb, both POLR1A and TP53 were silenced in U2OS and HCT-116 cells by siRNA expression. Similar to the previous result in p53+/+ cells, depletion of both p53 and POLR1A activity resulted in a significant reduction of cells in S phase. In a second experiment, a dominant negative inactive form of murine p53 (p53DD) was exogenously expressed in HCT-116 cells; POLR1A-silencing in these p53-deficient cells also caused a significant reduction in DNA synthesis.Citation243 With these two results, we can conclude that cell cycle arrest after POLR1A-silencing can occur in a p53-independent manner.
To assess pRb’s control of cell cycle progression in the absence of rRNA synthesis, Donati et al.Citation243 measured expression of E2F-1, the transcription factor whose activity is negatively regulated by pRb. After 48 h of POLR1A silencing, E2F-1 expression was reduced in both p53+/+ (U2OS) and p53-mutant (HCT-116 p53DD) cells. Both cell types arrested in G1. Since E2F-1 is primarily expressed in S phase, cells expressing p53DD and siRNA to target POLR1A were also treated with siRNAs to silence Retinoblastoma 1 (RB1). Silencing of RB1 and POLR1A in these p53-mutant cells actually rescued the cell cycle arrest.Citation243 In other words, after POLR1A-silencing, sufficient E2F-1 was made available to activate genes necessary for entry and progression through S phase, but only in the absence of pRb. After silencing RB1 and POLR1A in a p53DD mutant background, overall E2F-1 protein levels still declined however. These results indicate that downregulation of E2F-1 expression after POLR1A silencing is not just a consequence of changes in cell cycle progression and regulation by pRb; other E2F-1 regulators must be involved.
MDM2 is known to bind E2F-1 in human cancer cells (PC3 and DU145 prostate cancer cells) and to protect it from proteasome-dependent degradation by displacing SCFSKP2, the E2F-1 E3 ligase, in a p53-, p14Arf-, and pRb-independent manner.Citation246 Donati et al.Citation243 focused on discerning the role of RpL11, because it is an important regulator of MDM2.Citation247 Silencing of RPL11 caused an increase in POLR1A expression, likely as a consequence of Myc activation.Citation243,Citation248 In p53-mutant (HCT-116 p53DD) cells treated with siRNAs to deplete both RpL11 and POLR1A mRNA levels, E2F-1 protein levels did not change. These results contrast with those presented above; recall that in cells expressing RpL11, POLR1A depletion caused a significant decrease of E2F-1 protein levels. Therefore, availability of RpL11 is necessary for the downregulation of E2F-1.Citation243 The model presented by Donati et al.Citation243 suggests that ribosomal stress releases RpL11 that then binds to MDM2 (). Without an association with MDM2, E2F-1 becomes unstable and is eventually degraded, resulting in cell cycle arrest at G1.Citation243,Citation246 In summary, Donati et al.Citation243 demonstrated that the inhibition of rRNA synthesis downregulates the expression of E2F-1, which, in cells lacking p53, hinders cell cycle progression in a pRb-dependent manner. Loss of E2F-1 results from its release from MDM2 as RpL11 binds MDM2 during the ribosomal stress response.
Figure 4. p53-Independent Responses to Nucleolar Stress. (A) Donati et al.Citation243 expressed RNAi to silence POLR1A, a subunit of Pol I, in order to mimic nucleolar stress in p53-deficient HCT-110 human cancer cells. Donati et al.Citation243 proposes a model in which, during perturbations in ribosome biogenesis, modeled by silencing of POLR1A, RpL11 is released from ribosomes and subsequently associates with MDM2. The E2F-1-MDM2 interaction is severed, leading to proteasomal degradation of E2F-1 and cell cycle arrest. (B) In p53−/− erythroid cells, PIM1 kinase normally associates with RpS19. PIM1 kinase also phosphorylates the cell cycle inhibitor p27Kip1 at Thr157, thus marking it for degradation, allowing normal cell cycle progression. Iadevaia et al.Citation244 disrupted the RpS19/PIM1 kinase interaction, either by expressing RNAi against RpS19 or applying treatments known to induce nucleolar stress in human erythroleukemic TF-1 (p53+/+) and K562 (p53−/−) cell lines. PIM1 kinase, being unable to associate with the small subunit of the ribosome, is degraded, allowing p27Kip1 stabilization, cell cycle arrest, and apoptosis, even in K562 (p53−/−) cells.Citation244 (C) Russo et al.Citation267 overexpressed RpL3 in order to mimic a disruption of ribosome biogenesis. Upon RpL3 overexpression, a multi-protein complex containing RpL3, Sp1, and NPM formed at the p21 gene promoter, activating its expression, which resulted in cell cycle arrest or apoptosis. (D) Arf functions as a negative regulator of ribosome biogenesis. In Arf+/+ murine embryonic fibroblasts (MEFs), Arf associates with DDX5, a DEAD-box RNA helicase, sequestering it in the nucleoplasm, allowing normal ribosome production. In Arf−/− MEFs, DDX5 localizes to the rDNA promoters in the nucleolus, resulting in increased ribosome production. In p53−/−, Mdm2−/−, Arf−/− MEFs, exogenous expression of HA-Arf resulted in reduced ribosome production once again.Citation271
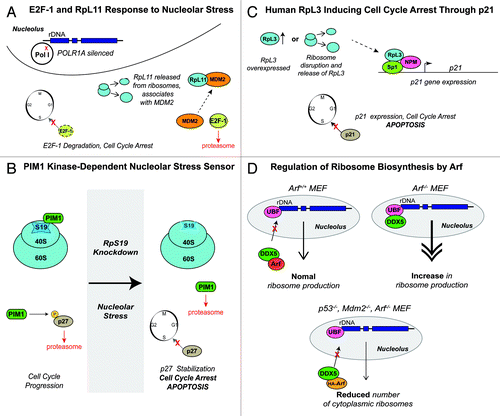
While considering the study by Donati et al.,Citation243 it is important to note that an inhibition in rRNA synthesis is not always associated with the downregulation of E2F-1. Previous studies investigating the link between nucleolar stress and the cell cycle have found that treatment with certain DNA-damaging drugs (actinomycin D, cisplatin, or etoposide) causes an accumulation of E2F-1.Citation249,Citation250 However, in current efforts to develop anti-neoplastic drugs that target p53-deficient cancer cells, the ability to specifically target Pol I activity, reduce rRNA synthesis, and downregulate E2F-1 to induce cell cycle arrest without genotoxic effects should prove most useful, in essence preventing new mutations that may give these cancer cells an ability to evade cell death.Citation243
Besides E2F-1 and Rb, other regulators affect p53-independent nucleolar stress. For example, PIM1 is a constitutively active serine/threonine kinase involved in cell cycle regulation and apoptosis.Citation251,Citation252 Two-hybrid screens and co-immunoprecipitation experiments showed that PIM1 interacts with RpS19 and co-sediments with ribosomes in sucrose gradients.Citation253 Iadevaia et al.Citation244 examined the PIM1/ribosome interaction by expressing RNAi against RpS19 or by applying treatments (such as actinomycin D, camtothecin, and cisplatinum) to disrupt ribosome synthesis in human erythroleukemic TF-1 (p53+/+) and K562 (p53−/−) cell lines. They then measured the resulting expression of PIM1 (), and showed that nucleolar stress caused a drop in PIM1 protein levels due to proteasomal degradation. With the loss of PIM1, levels of the cell cycle inhibitor p27Kip1 increased with a corresponding block in cell division, even in p53−/− cells (K562 cells).Citation244 PIM1 kinase phosphorylates Threonine-157 in p27Kip1 which marks the protein for degradation.Citation254 Indeed, lower levels of Thr157-phosphorylated p27Kip1 were present after PIM1 destabilization in both p53+/+ and p53−/− cells as measured by western blot. Therefore, a decrease of PIM1 activity leads to stabilization and accumulation of p27Kip1 and consequently cell cycle arrest regardless of the p53 status of the cell. It is currently unclear if PIM1-dependent sensing of nucleolar stress acts independently or concurrently with known p53-dependent pathways.Citation244
Another example involves PeBoW, the nucleolar complex in mammalian cells that plays an essential role in processing pre-rRNA during 60S ribosomal subunit assembly. The complex is comprised of pescadillo (Pes1), Bop1 (block of proliferation), and WDR12 (WD repeat) proteins.Citation225,Citation255 In a study linking the function of pescadillo to human breast cancer, Li et al.Citation245 surveyed seven breast cancer cell lines, all of which demonstrated upregulation of pescadillo at the mRNA and protein levels compared with normal breast epithelial tissue. Knockdown of pescadillo expression by RNAi in both p53−/− (MDA-MB-435) and p53+/+ (ZR-75–30) breast cancer cells led to an inhibition of cell division and to a decrease in the cells’ colony forming ability on soft agar.Citation245 Pescadillo depletion also led to decreased expression of the cell cycle protein cyclin D1, upregulation of the CDK inhibitor p27Kip1, and consequent reduction of pRb phosphorylation, which led to cell cycle arrest in both p53−/− and p53+/+ breast cancer cell lines.Citation245
Drug induced nucleolar stress in the absence of p53
More than 50% of human cancers lack functional p53.Citation256 For instance, deletion of p53 has been reported in 10% of newly diagnosed multiple myeloma (MM) tumors; also, the occurrence of a p53 deletion is much greater in advanced and extra-medullary tumors, which correlates with a poor prognosis.Citation257,Citation258 Therefore, drugs that trigger cell death in p53-null cells could have great potential in the treatment of many cancers. As cyclin dependent kinases (CDKs) represent a large family of proteins that regulate the cell cycle and transcription,Citation259 small molecule multi-targeted CDK inhibitors (CDKIs) have shown anti-tumor activity regardless of the p53 status of the tumor cell. The compound RGB-286638, a new type of indenopryazole family of cyclin dependent kinase (CDK) inhibitors, is potent against transcriptional-type CDKs in both p53-wild type and p53-null MM cells.Citation260
To investigate the dose- and time-dependent effects of RGB-286638 treatment, Cirstea et al.Citation260 used both p53-wild type (MM.1S, MM.1R, and H929) and p53-null MM cells (U266, OPM1, RPMI). Cell viability was measured 48 h after a 50 nM RGB-286638 treatment; p53-wild type cells (MM1.S, which had p53 stabilization and activation) were slightly more sensitive than p53-null cells. Apoptosis was induced in both p53-wild type and p53-null cells, as measured by cleavage of PARP and activation of caspases-8, -9, and -3 after a 4-h treatment with RGB-286638. Expression of shRNA to knock down p53 function in MM.1S cells led to a partial rescue of apoptosis (14% of cells), suggesting that p53 does have some role in RGB-286638-induced cell death. RGB-286638 treatment (50 nM) of p53-mutant cells caused reduced total RNA synthesis after 2 h and a significant reduction after 24 h as measured by [3H]-uridine incorporation. Additionally, RGB-286638 treatment (50 nM) of p53-wild type cells for 3 h triggered formation of numerous nuclear speckles as seen by immunofluorescence, which is indicative of nucleolar segregation. These results indicate that the CDK inhibitor, RGB-286638 can induce cell death in p53-wild type MM cells by activation of p53, as well as in p53-null MM cells through p53-independent mechanisms. Significant advances in targeting cancer cells that lack functional p53 requires a thorough understanding of predictably latent, more ancestral mechanisms that lead to cell cycle arrest or apoptosis in the absence of p53.
p53-independent autophagy
Autophagy is a pro-survival process that involves sequestration of cytoplasm into membrane bound vesicles that fuse with lysosomes resulting in the recycling of cellular components.Citation261 Boglev et al.Citation262 reported a newly discovered link between nucleolar stress and the induction of autophagy, which occurs in a p53-independent manner. Boglev et al.Citation262 described the novel zebra fish mutant titania (ttis450), a recessive lethal mutation located in pwp2h, the gene that encodes a subunit of the small ribosomal subunit processome. Pwp2h is especially necessary for 18S rRNA maturation during ribosome biogenesis. In yeast, Pwp2h was shown to be an essential scaffold component of the 90S pre-ribosomal processome, aiding in the interaction of proteins between the U3 snoRNP and the 5′ end of pre-rRNA.Citation263,Citation264 titanias450 larvae exhibit defects in intestinal, liver, pancreas, and craniofacial development. Northern blots and polysome profiles using titanias450 larvae showed an accumulation of rRNA processing intermediates and a reduction of 40S subunits and 80S monosomes respectively.Citation262 These results support previous findings that Pwp2h is necessary for proper maturation of the small ribosomal subunit. Transmission electron microscopic comparisons of intestinal epithelial tissues between titanias450 and wild type larvae indicated the presence of autophagosome- and autolysosome-like structures present in titanias450 larvae. Immunohistochemical studies were also conducted using mCherry-LC3IIl, the membrane-bound mammalian ortholog of Atg8a and a robust marker for autophagosome formation.Citation262 The results of this experiment confirmed the TEM results, showing intestinal epithelial cells that had lost Pwp2h function experienced significantly more autophagy compared with similar wild type tissues. Autophagy induction in titanias450 larvae extends their lifespan and prolongs the survival of the intestinal cells.Citation262 Expression of a p53 mutant, which is unable to bind DNA, failed to rescue the autophagy phenotype in titanias450 larvae. Autophagy induction in titanias450 larvae is also independent of the level of activation of the Tor pathway and the levels of RpS6, a downstream target of Tor activity.Citation262 From this study, we can conclude that autophagy is a survival mechanism that can be induced by ribosomal stress, even in a p53- and Tor- independent manner. In the context of cancer research, it is important to consider that certain therapeutic agents may promote autophagy, contributing to cancer cells’ evasion cell death.Citation262
Role of ribosomal proteins in p53-independent nucleolar stress
c-Myc is a master regulator of cell division, as it controls the transcription of all three RNA polymerases and therefore affects all steps in ribosome biogenesis. In particular, c-Myc facilitates the recruitment of SL1 to Pol I promoters and controls expression of UBF, which is essential for Pol I transcription.Citation265 c-Myc also facilitates expression of ribosomal proteins by increasing Pol II transcription and enhances Pol III activity by interacting with TFIIIB.Citation266 When overexpressed, RpL11 binds both c-Myc and its mRNA, which promotes degradation of this mRNA, thus revealing an elegant auto-regulatory feedback mechanism as c-Myc also activates expression of RpL11.Citation248 Overexpression of RpL11 was used to mimic what can occur in cells during ribosomal stress; specifically, free RpL11 is available to bind factors.Citation107 RpL11 and c-Myc were expressed from recombinant adenovirus, and BrdU incorporation was used to monitor cell cycle progression.Citation248 While overexpression of c-Myc alone significantly increased the number of U2OS cells undergoing DNA synthesis as expected, co-expression of RpL11 and c-Myc reduced the number of cells in S phase, suggesting that RpL11 blocked c-Myc function. The same experiment using p53-null MEF cells also generated RpL11-dependent cell cycle arrest. Immuno-precipitation analysis revealed that c-Myc and RpL11 interact in both Arf-null U2OS cells and in p53−/−mdm2−/− MEF cells. On the other hand, transient RNAi depletion of RpL11 led to an increase in c-Myc protein levels.Citation248 RpL11 may also inhibit c-Myc activity by preventing the recruitment of its co-activator, TRRAP, to c-Myc target genes transcribed by Pol I and Pol II.Citation248 These findings suggest an expanded role of RpL11, beyond its well-studied interaction with MDM2 during ribosomal stress leading to p53 activation and apoptosis (described above).
RpL11 is not the only ribosomal protein reported to have extra-ribosomal function during p53-independent nucleolar stress. Russo et al.Citation267 described a new p53-independent role for RpL3 in modulating cell cycle progression through its positive regulation of p21 expression (). p21 is a main inhibitor of cyclin dependent kinases and its p53-dependent expression results in cell cycle arrest at the G1/S phase transition. Russo et al.Citation267 overexpressed RpL3 in an attempt to mimic situations of altered ribosome biogenesis. As mentioned before, during nucleolar stress, certain ribosomal proteins can accumulate either by de-novo synthesis or by protection from degradation when ribosomes disassemble.Citation107,Citation110,Citation242 In human Calu-6 cells, which lack a functional p53, overexpression of RpL3 led to a corresponding dose-dependent increase in p21 expression as measured by RT-PCR.Citation267 ChIP assays suggested that RpL3 interacts with the promoter of p21, but mobility shift assays (EMSA) revealed that RpL3 does not bind to the promoter directly, so other factors must be involved.Citation267 ChIP assays identified nucleophosmin (NPM), a regulator of RpL3 alternative splicing, and Sp1, a known regulator of p21 expression, present at the p21 gene promoter with RpL3.Citation267-Citation269 In addition, luciferase expression assays engineered with the Sp1 promoter showed that RpL3 and Sp1 expression had a greater positive effect on p21 expression than did expression of either RpL3 or Sp1 alone.Citation267 These data suggest a model in which overexpression of RpL3 causes the formation of a multi-protein complex containing at minimum RpL3, NPM, and Sp1; this complex binds to the p21 promoter, causing an upregulation of p21 expression thus leading to either cell cycle arrest or mitochondrial-dependent apoptosis.Citation267
p53-independent functions of Arf
p19Arf functions as a tumor-suppressor in mammals, but it also functions in the regulation of ribosome biogenesis; specifically, Arf regulates nucleolar export of nucleophosmin (NPM1) as well as MDM2, suggesting that it plays a role as a nucleolar stress sensor or monitor of nucleolar function.Citation78 Sugimoto et al.Citation270 demonstrated that nucleolar Arf inhibits pre-rRNA processing, retarding the processing of 47/45S and 32S precursors. This experiment was conducted using NIH-3T3 cells which lack endogenous Ink4a-Arf alleles, so exogenous Arf was expressed using a zinc-inducible metallothionin promoter. Arf’s interference with pre-rRNA processing and its interaction with the 5.8S rRNA require the N-terminus of Arf, but neither MDM2 nor p53.Citation270 However, this finding did not quite elucidate the mechanism by which Arf regulates rRNA processing.
To approach this mechanism, Saporita et al.Citation271 performed a proteomics screen using wild type and Arf −/− mouse embryonic fibroblasts (MEFs) to determine how levels of Arf might affect nucleolar composition and function. DDX5, a DEAD-box RNA helicase (also known as p68), was among the proteins enriched in the nucleolus upon Arf depletion. There, it associates with UBF at rDNA promoter sites.Citation36 Arf prevents DDX5 from localizing to nucleoli in a p53-independent fashion ().Citation271 In the Arf −/− MEFs, DDX5 occupancy at rDNA promoters was 2-fold greater than that in wild type cells. DDX5 localization at rDNA promoters resulted in an increase in rRNA synthesis and processing. Increased production of 47S rRNA transcripts still occurred upon expression of an ATP-binding mutant of DDX5, therefore the helicase activity of DDX5 is not necessary for Pol I transcription. Conversely, knockdown of DDX5 by shRNA reduced cell division of Arf −/− and p53−/− MEFs. To further investigate p53-independent functions of Arf, the authors used triple knockout MEFs (p53−/−, Mdm2−/−, Arf −/−), thus eliminating the entire Arf-MDM2-p53 cascade. Expression of HA-tagged Arf in these cells resulting in a reduced number of cytoplasmic ribosomes in the polysome fraction of sucrose gradients. These results are consistent with earlier observations indicating Arf actually inhibits ribosome biogenesis in a p53-independent manner. Saporita et al.Citation271 concluded that Arf acts to protect against non-oncogene driven ribosome biogenesis in order to prevent cell transformation.
p53-independent nucleolar stress in Drosophila melanogaster
To this point, our discussion has described p53-independent nucleolar (ribosomal) stress in yeast which lacks p53 and in vertebrate (mammalian) cells where the p53-independent pathways remain minor compared with the preferred MDM2- and p53-dependent pathways. C. elegans and Drosophila express p53, but they lack MDM2 and Arf, thus nucleolar stress in these organisms is necessarily quite different than in mammalian cells. Is p53 required for nucleolar stress in Drosophila? If p53 is not required for nucleolar stress in Drosophila, what forms of nucleolar stress occur in Drosophila, and how are they activated? If similar mechanisms still exist in mammalian cells but remain latently silent, can oncologists one day induce these pathways as therapies to target cancer cells that lack p53?
Functions of p53 in Drosophila melanogaster
In vertebrates, the p53 paralogs p63 and p73 present a challenge in studying p53 function.Citation272 D. melanogaster, however, contains one p53 gene (Dp53) within the haploid genome, and its protein product is highly conserved in gene structure compared with mammalian p53. The single Dp53 gene, however, contains two alternative promoters that can give rise to three possible protein isoforms: Dp53, DΔNp53 (discovered first), and Dp53ΔC (the third of which has not been experimentally confirmed).Citation273 Dp53-null flies are viable and fertile. Apart from a defect in primordial germ cells, no developmental defects are observed in these Dp53-null flies.Citation274,Citation275 While conserved in sequence, the activation of Dp53 in flies is quite different than activation of p53 in mammalian cells. There are no obvious MDM2 homologs in D. melanogaster, suggesting that either Dp53 is not regulated by protein turnover, or the sequence of the fly homolog of MDM2 is too dissimilar from its mammalian counterpart to have been identified already by sequence searches.Citation274 Additionally, Dp53 does not contain a conserved MDM2 binding domain as does mammalian p53 (), thus supporting the fact that Dp53 is not regulated by MDM2 association and protein degradation and as it is in mammals.Citation276
The consequence of activating Dp53 in D. melanogaster is also quite different than activating p53 in mammals; Dp53 is unable to induce cell cycle arrest upon radiation treatment nor is it able to activate decapo, the gene encoding the p21 homolog.Citation274,Citation277-Citation279 While cell cycle control may not respond to Dp53, the pro-apoptotic function of Dp53 seems to be conserved, as both Dp53 and DΔNp53 are capable of activating apoptosis, but through distinct mechanisms involving different pro-apoptotic regulators, reaper, hid, and grim, which are often referred to the RHG proteins. These are pro-apoptotic proteins as they inhibit Drosophila Inhibitor of Apoptosis (DIAP). Dp53 induces reaper while DΔNp53 induces hid.Citation280 Expression of Dp53 from the GMR promoter (specific for the eye imaginal disc) or from a modified upstream activating sequence (UAS)-Gal4 system induces apoptotic cell death of third instar eye disc cells, as visualized by anti-cleaved caspase-3 and TUNEL labeling.Citation280 This expression of Dp53 resulted in small, rough eyes with a glossy appearance; this phenotype can be rescued by co-expression of a dominant negative form of Dp53 or by expressing siRNA to silence Dp53.Citation281 Of the RHG proteins, hid is the primary mediator of apoptosis induced after expression of Dp53 in the eye disc; expression of null-mutants of hid suppressed most of the Dp53-induced cell death in the eye disc.Citation281 On the other hand, co-expression of Dp53 and the caspase inhibitor p35 (in order to block apoptosis) did not rescue the eye phenotype, so GMR-controlled expression of Dp53 causes cell differentiation defects independently from its apoptosis-inducing function in photoreceptor neurons and cone cells.Citation281
Interestingly, expression of either human p21 or Drosophila decapo from the GMR-promoter suppressed Dp53-induced apoptosis and developmental defects as well as a significant reduction of hid expression. This suggests that p21 and decapo act upstream of Dp53 (in contrast to in mammals, where p21 is a transcriptional target of p53). decapo expression did not affect Dp53 protein levels, indicating that it does not interfere with its expression or stability. Fan et al.Citation281 hypothesize that p21 may interact with Dp53’s ability to bind to downstream gene promoters (like hid) or by other indirect methods, but acknowledge that further investigation is required to decipher this mode of regulation.
p53-independent nucleolar stress in D. melanogaster
As described above, TIF-IA is a critical transcription initiation factor for RNA Pol I, and as in mammals it is required for ribosome biogenesis in Drosophila.Citation282 In flies, loss of TIF-IA induces p53-independent phenotypes; tifia−/− p53−/− had the same growth arrest as did tifia−/− larvae. This finding contrasts with studies using mammalian cells, where cell cycle arrest and apoptosis caused by the loss of TIF-IA could be rescued upon genetic loss of p53.Citation283
Brodsky et al.Citation274 described a DNA damage induced pathway that arrests the cell cycle and initiates apoptosis in Drosophila. After treating larval imaginal eye and wing discs with ionizing radiation (IR), Dp53 protein levels did not change, but its phosphorylation pattern changed; this phosphorylation is sufficient for its activation.Citation274 MNK (Chk2 in Drosophila) is required for IR induced phosphorylation and/or activation of Dp53, and MNK along with Dp53 are required for IR-induced gene expression in Drosophila.Citation274 Microarray and qPCR methods identified several genes activated by IR treatment. These include Mre11 and Rad50, two proteins that form part of a larger complex including NBS1.Citation284 This complex has been implicated in DNA repair mechanisms such as homologous recombination and non-homologous end joining (NHEJ), and with cell cycle delay. Ku70, Ku80 were also upregulated; these proteins are required for NHEJ. Reaper, Sickle, and Hid (the RHG proteins) were upregulated within 30 min of IR treatment. Mutant Dp53−/−, MNK−/− cells failed to activate apoptosis and gene expression upon IR treatment, suggesting that MNK is required for phosphorylation of p53 following IR-induced damage. As visualized by an antibody specific for mitotic cells (anti-histone H3), IR-induced cell cycle arrest at G2 still occurred in Dp53-deficient cells.Citation274 Also, decapo (p21 homolog) was repressed following IR treatment of Dp53-deficient cells, adding evidence to previous reports that Dp53 is not necessary for cell cycle arrest.Citation274 On the other hand, ATR and GPRS (Chk1 in Drosophila) were necessary for cell cycle arrest upon IR treatment.Citation274
Brodsky et al.Citation274 also observed that IR induced a p53-independent decrease in mRNA levels of at least 17 genes, many of which were developmental regulators. The authors favor a model that includes MNK-dependent cell cycle delay upon IR treatment with developmental gene induction as a secondary effect.Citation274 They conclude that MNK activates diverse pathways including Dp53-dependent gene expression. The phosphorylation of p53 by Chk2/MNK is conserved among metazoans. MNK, however, has Dp53-independent roles as well. For meiotic checkpoint regulation, MNK activation requires MEI-41 (ATR).Citation274 MNK, but not p53, is also necessary for damage-induced inactivation of centrosomes.Citation285 The presence of MDM2 in mammals likely adds an evolutionarily advanced layer of control over p53 timing and function.Citation274
McNamee and BrodskyCitation286 also described a Dp53-independent, but JNK- and HID-dependent apoptotic pathway that was activated upon haplo-insufficiency of ribosomal proteins after ionizing radiation (IR) treatment. In flies, ribosomal protein genes are spread throughout the genome,Citation198 therefore chromosomal breaks prior to mitosis often cause segmental aneuploidy of the ribosomal protein genes resulting in a Minute phenotype in the daughter cells. McNamee and BrodskyCitation286 treated larval wing discs with ionizing radiation (IR), and then counted the number of shorter, thinner Minute bristles after the adult flies eclosed. There was no difference in the number of defective (Minute) bristles after IR treatment and in several lines that either harbored Dp53−/− or Dp53+/+ genotypes. Thus, Dp53 function was not necessary for the elimination of cells that were haplo-insufficient for ribosomal protein genes (the Minute phenotype).
Phenotypes normally associated with the Minute phenotype involve increased expression of c-Jun N-terminal kinase (JNK) and Brinker (brk, the upstream kinase and activator of JNK), and reduced expression of the survival factor decapentaplegic (dpp).Citation287,Citation288 Indeed, brk expression was observed in wild type cells 4 h after IR treatment, which was sufficient to activate JNK-dependent apoptosis.Citation286,Citation289
McNamee and BrodskyCitation286 also monitored the activity of JNK after IR treatment of imaginal disc cells. An important downstream target of JNK signaling is puckered (puc), which encodes the JNK phosphatase that acts as a repressor of JNK function, forming a negative regulatory loop. Recall, IR-treated cells likely have mutations in genes encoding ribosomal proteins, which, as described earlier, would be considered Minute mutations. JNK activity in these cells can be modulated by way of changing puc expression. There was a 3- to 4-fold increase in apoptosis in puc-/+, p53−/− mutant wing discs compared with just p53−/− cells at 16 to 24 h following IR treatment as visualized by anti-cleaved caspase staining. So, with only one functional copy of puc present, negative regulation of JNK activity was reduced in p53−/− cells, and apoptosis was able to proceed more efficiently. Conversely, overexpression of puc led to greater negative regulation on JNK activity, and to reduced hid expression and apoptosis. These results indicate that the JNK pathway plays an important role in the elimination of Minute wing disc cells upon IR treatment. JNK activates the transcription of hid (which then leads to activation of the caspase pathway, including caspase-3). Therefore p53-independent apoptosis must use the same core apoptotic machinery that is activated in most Drosophila cell stress and developmental signals.Citation286
McNamee and BrodskyCitation286 further investigated cell cycle regulation along with reducing the level of negative feedback signaling after IR treatment of wing discs. Drosophila grp is the Chk1 kinase homolog.Citation290 Triple mutant wing discs (grp−/−, puc-/+, p53−/−) displayed higher levels of apoptosis, as visualized by anti-caspase 3 staining, compared with either grp−/−, Dp53−/− or puc-/+, Dp53−/− double mutant wing discs.Citation285 Therefore, decreasing the negative feedback signal from puc to JNK further increased the number of cells that underwent p53-independent apoptosis following IR.Citation286 In Dp53−/− and mnk−/− mutant discs, IR-induced apoptosis was seen only after cells recovered from damage induced cell cycle delay.Citation286 The apoptotic response was accelerated in these double mutant discs that failed to arrest at G2/M due to mutations in grp (Chk1). Overall, these results suggest that cell cycle arrest helps delay p53-independent activation of JNK signaling and apoptosis following IR.Citation286
McNamee and BrodskyCitation286 concluded that this p53-independent pathway acts in parallel with the canonical DNA damage response pathway to eliminate cells with altered genomes following IR. Also, JNK activation acts as compensation upon loss of p53 to preserve genome integrity, and that JNK may be activated upon loss of certain genes, such as the ribosomal genes in aneuploid cells. In support of these conclusions, Titen and GolicCitation291 reported that p53-independent apoptosis is activated upon telomere loss only if the resultant chromosome leads to aneuploidy.
Direct nucleolar stress responses induced in Drosophila
As described above, Nopp140 is an assembly nucleolar factor or chaperone for Box C/D and Box H/ACA snoRNP complexes that modify pre-rRNA during ribosome biogenesis. Loss of Nopp140 function upon expression of shRNA leads to several phenotypes in D. melanogaster. These include apoptosis within larval imaginal wing disc cells, autophagy of larval polyploid gut cells, an increase in activated phospho-JNK levels, a reduction of RpL23a and RpL34 protein levels as visualized by immuno-blot, reduction of cytoplasmic ribosomes as seen by TEM, and a reduction of protein synthesis as measured by metabolic labeling assays.Citation206 Apoptosis occurred after expressing Nopp140-RNAi within the larval wing discs as observed by immuno-fluorescence with an anti-cleaved caspase-3 antibody. When these larvae eclosed as flies, their wings showed a vestigial-like phenotype in which they were shriveled and blistered. Following the murine Treacher Collins study by Jones et al.Citation137 in which nucleolar stress was rescued by the depletion of p53 (described above), we reasoned that blocking Dp53 function would rescue this wing phenotype. However, expressing Nopp140-RNAi in the Dp53−/− background failed to rescue the apoptosis in wing disc cells and the resulting shriveled (vestigial) wing phenotype. Thus, a Dp53-independent, but JNK-dependent pathway must activate apoptosis upon depletion of Nopp140. Our findings supported the observations of McNamee and Brodsky.Citation286
Prospects
We have seen how ribosomal proteins in mammalian cells, released under nucleolar stress conditions, bind and suppress MDM2 leading to p53 stabilization and/or activation that then induces cell cycle arrest or apoptosis. Discovery of this phenomenon has rekindled our attempts at selectively inducing nucleolar stress in highly metabolic cancer cells, hopefully forcing these cells into cycle arrest or apoptosis while normal cells with reduced requirements for ribosome synthesis remain relatively unscathed. These are exciting times for nucleolar biologists as they work with structural biochemists and organic chemists to devise new drugs that specifically block ribosome biogenesis at the level of Pol I transcription or pre-rRNA processing in the hopes of using nucleolar stress as an effective cancer treatment. Many types of cancer cells, however, lack functional p53, and the question remains: can oncologists still use nucleolar stress as an effective strategy in treating these p53-minus cancers? We cited several examples of mammalian cells undergoing p53-independent cycle arrest upon nucleolar stress, but the detailed mechanisms of how this comes about remain sketchy. Future studies will likely reveal the details of p53-independent nucleolar stress mechanisms that either remain generally silent in mammalian cells due to evolutionary emergence of the predominant MDM2-p53 pathway, or are active in mammalian cells but have simply gone unrecognized. A careful examination of nucleolar stress in non-mammalian systems such as yeast that lacks p53 or C. elegans and Drosophila that express p53 but lack MDM2, may reveal conserved aspects of p53-independent nuclear stress pathways throughout the eukaryotes. Our full understanding of these p53-independent pathways may likewise reveal targets for effective cancer treatments.
Disclosure of Potential Conflicts of Interest
No potential conflict of interest was disclosed.
Acknowledgments
We apologize for not including many other excellent references describing nucleolar and ribosome stress. Portions of our work briefly described here were supported by the National Science Foundation, award MCB-0919709.
References
- Pederson T. The plurifunctional nucleolus. Nucleic Acids Res 1998; 26:3871 - 6; http://dx.doi.org/10.1093/nar/26.17.3871; PMID: 9705492
- Boisvert F-M, van Koningsbruggen S, Navascués J, Lamond AI. The multifunctional nucleolus. Nat Rev Mol Cell Biol 2007; 8:574 - 85; http://dx.doi.org/10.1038/nrm2184; PMID: 17519961
- Olson MOJ. Sensing cellular stress: another new function for the nucleolus?. Sci STKE 2004; 2004:pe10; PMID: 15026578
- Hein N, Hannan KM, George AJ, Sanij E, Hannan RD. The nucleolus: an emerging target for cancer therapy. Trends Mol Med 2013; 19:643 - 54; http://dx.doi.org/10.1016/j.molmed.2013.07.005; PMID: 23953479
- Drygin D, O’Brien SE, Hannan RD, McArthur GA, Von Hoff DD. Targeting the nucleolus for cancer-specific activation of p53. Drug Discov Today 2014; 19:259 - 65; http://dx.doi.org/10.1016/j.drudis.2013.08.012; PMID: 23993916
- Vlatković N, Boyd MT, Rubbi CP. Nucleolar control of p53: a cellular Achilles’ heel and a target for cancer therapy. Cell Mol Life Sci 2014; 71:771 - 91; http://dx.doi.org/10.1007/s00018-013-1361-x; PMID: 23685903
- Grummt I. The nucleolus—guardian of cellular homeostasis and genome integrity. Chromosoma 2013; 122:487 - 97; http://dx.doi.org/10.1007/s00412-013-0430-0; PMID: 24022641
- Montanaro L, Treré D, Derenzini M. The emerging role of RNA polymerase I transcription machinery in human malignancy: a clinical perspective. Onco Targets Ther 2013; 6:909 - 16; PMID: 23888116
- Parlato R, Kreiner G. Nucleolar activity in neurodegenerative diseases: a missing piece of the puzzle?. J Mol Med (Berl) 2013; 91:541 - 7; http://dx.doi.org/10.1007/s00109-012-0981-1; PMID: 23179684
- Quin JE, Devlin JR, Cameron D, Hannan KM, Pearson RB, Hannan RD. Targeting the nucleolus for cancer intervention. Biochim Biophys Acta 2014; 1842:802 - 16; http://dx.doi.org/10.1016/j.bbadis.2013.12.009; PMID: 24389329
- Golomb L, Volarevic S, Oren M. p53 and ribosome biogenesis stress: The essentials. FEBS Lett 2014; 588:2571 - 9; http://dx.doi.org/10.1016/j.febslet.2014.04.014; PMID: 24747423
- Tsai RYL, Pederson T. Connecting the nucleolus to the cell cycle and human disease. FASEB J 2014; 28:3290 - 6; http://dx.doi.org/10.1096/fj.14-254680; PMID: 24790035
- Busch H, Smetana K. The Nucleolus 1970 New York, Academic Press.
- Jordan EG, Cullis CA. 1982. The Nucleolus. Cambridge University Press.
- Hadjiolov AA. The nucleolus and ribosome biogenesis. Springer-Verlag, 1985.
- Olson MOJ. The Nucleolus. Springer, 2011.
- Perry RP, Errera M. The role of the nucleolus in ribonucleic acid-and protein synthesis. I. Incorporation of cytidine into normal and nucleolar inactivated HeLa cells. Biochim Biophys Acta 1961; 49:47 - 57; http://dx.doi.org/10.1016/0006-3002(61)90868-X; PMID: 13734590
- Perry RP. The cellular sites of synthesis of ribosomal and 4 S RNA. Proc Natl Acad Sci U S A 1962; 48:2179 - 86; http://dx.doi.org/10.1073/pnas.48.12.2179; PMID: 16591032
- Edström JE, Grampp W, Schor N. The intracellular distribution and heterogeneity of ribonucleic acid in starfish oocytes. J Biophys Biochem Cytol 1961; 11:549 - 57; http://dx.doi.org/10.1083/jcb.11.3.549; PMID: 13889253
- Warner JR, Soeiro R. Nascent ribosomes from HeLa cells. Proc Natl Acad Sci U S A 1967; 58:1984 - 90; http://dx.doi.org/10.1073/pnas.58.5.1984; PMID: 5237493
- Brown DD, Gurdon JB. Absence of ribosomal RNA synthesis in the anucleate mutant of Xenopus laevis.. Proc Natl Acad Sci U S A 1964; 51:139 - 46; http://dx.doi.org/10.1073/pnas.51.1.139; PMID: 14106673
- Ritossa FM, Spiegelman S. Localization of DNA complementary to ribosomal RNA in the nucleolus organizer region of Drosophila melanogaster.. Proc Natl Acad Sci U S A 1965; 53:737 - 45; http://dx.doi.org/10.1073/pnas.53.4.737; PMID: 14324529
- Ritossa FM, Atwood KC, Lindsley DL, Spiegelman S. On the chromosomal distribution of DNA complementary to ribosomal and soluble RNA. Natl Cancer Inst Monogr 1966; 23:449 - 72; PMID: 5963988
- Birnstiel ML, Wallace H, Sirlin JL, Fischberg M. Localization of the ribosomal DNA complements in the nucleolar organizer region of Xenopus laevis. Natl Cancer Inst Monogr 1966; 23:431 - 47; PMID: 5963987
- Heitz E. Über totale und partielle somatische heterpyknose, sowie strukturelle geschlectschromosemen bie Drosophila funebris.. Z Zellforsch Mikrosk Anat 1933; 19:720 - 42; http://dx.doi.org/10.1007/BF02450275
- McClintock B. The relation of a particular chromosomal element to the development of the nucleoli in Zea mays.. Z Zellforsch Mikrosk Anat 1934; 21:294 - 328; http://dx.doi.org/10.1007/BF00374060
- Miller OL Jr., Beatty BR. Visualization of nucleolar genes. Science 1969; 164:955 - 7; http://dx.doi.org/10.1126/science.164.3882.955; PMID: 5813982
- Voit R, Grummt I. The RNA polymerase I transcription machinery. In: Olson MOJ, ed. The Nucleolus, Protein Reviews. New York, NY: Springer, 2011:107-134
- Hernandez-Verdun D. Assembly and disassembly of the nucleolus during the cell cycle. Nucleus 2011; 2:189 - 94; http://dx.doi.org/10.4161/nucl.2.3.16246; PMID: 21818412
- French SL, Osheim YN, Schneider DA, Sikes ML, Fernandez CF, Copela LA, Misra VA, Nomura M, Wolin SL, Beyer AL. Visual analysis of the yeast 5S rRNA gene transcriptome: regulation and role of La protein. Mol Cell Biol 2008; 28:4576 - 87; http://dx.doi.org/10.1128/MCB.00127-08; PMID: 18474615
- Moss T, Stefanovsky VY. At the center of eukaryotic life. Cell 2002; 109:545 - 8; http://dx.doi.org/10.1016/S0092-8674(02)00761-4; PMID: 12062097
- Grummt I. Life on a planet of its own: regulation of RNA polymerase I transcription in the nucleolus. Genes Dev 2003; 17:1691 - 702; http://dx.doi.org/10.1101/gad.1098503R; PMID: 12865296
- Goodfellow SJ, Zomerdijk JCBM. Basic mechanisms in RNA polymerase I transcription of the ribosomal RNA genes. Subcell Biochem 2013; 61:211 - 36; http://dx.doi.org/10.1007/978-94-007-4525-4_10; PMID: 23150253
- Chen S, Seiler J, Santiago-Reichelt M, Felbel K, Grummt I, Voit R. Repression of RNA polymerase I upon stress is caused by inhibition of RNA-dependent deacetylation of PAF53 by SIRT7. Mol Cell 2013; 52:303 - 13; http://dx.doi.org/10.1016/j.molcel.2013.10.010; PMID: 24207024
- Grob A, Colleran C, McStay B. UBF an essential player in maintenance of active NORs and nucleolar formation. In: Olson MOJ, ed. The Nucleolus, Protein Reviews. New York, NY: Springer, 2011:83-103
- O’Sullivan AC, Sullivan GJ, McStay B. UBF binding in vivo is not restricted to regulatory sequences within the vertebrate ribosomal DNA repeat. Mol Cell Biol 2002; 22:657 - 68; http://dx.doi.org/10.1128/MCB.22.2.657-668.2002; PMID: 11756560
- Zhao J, Yuan X, Frödin M, Grummt I. ERK-dependent phosphorylation of the transcription initiation factor TIF-IA is required for RNA polymerase I transcription and cell growth. Mol Cell 2003; 11:405 - 13; http://dx.doi.org/10.1016/S1097-2765(03)00036-4; PMID: 12620228
- Mayer C, Zhao J, Yuan X, Grummt I. mTOR-dependent activation of the transcription factor TIF-IA links rRNA synthesis to nutrient availability. Genes Dev 2004; 18:423 - 34; http://dx.doi.org/10.1101/gad.285504; PMID: 15004009
- Mayer C, Bierhoff H, Grummt I. The nucleolus as a stress sensor: JNK2 inactivates the transcription factor TIF-IA and down-regulates rRNA synthesis. Genes Dev 2005; 19:933 - 41; http://dx.doi.org/10.1101/gad.333205; PMID: 15805466
- Mayer C, Grummt I. Cellular stress and nucleolar function. Cell Cycle 2005; 4:1036 - 8; http://dx.doi.org/10.4161/cc.4.8.1925; PMID: 16205120
- Staley JP, Woolford JL Jr.. Assembly of ribosomes and spliceosomes: complex ribonucleoprotein machines. Curr Opin Cell Biol 2009; 21:109 - 18; http://dx.doi.org/10.1016/j.ceb.2009.01.003; PMID: 19167202
- Bleichert F, Baserga SJ. The long unwinding road of RNA helicases. Mol Cell 2007; 27:339 - 52; http://dx.doi.org/10.1016/j.molcel.2007.07.014; PMID: 17679086
- Ginisty H, Sicard H, Roger B, Bouvet P. Structure and functions of nucleolin. J Cell Sci 1999; 112:761 - 72; PMID: 10036227
- Roger B, Moisand A, Amalric F, Bouvet P. Nucleolin provides a link between RNA polymerase I transcription and pre-ribosome assembly. Chromosoma 2003; 111:399 - 407; http://dx.doi.org/10.1007/s00412-002-0221-5; PMID: 12644954
- Rickards B, Flint SJ, Cole MD, LeRoy G. Nucleolin is required for RNA polymerase I transcription in vivo. Mol Cell Biol 2007; 27:937 - 48; http://dx.doi.org/10.1128/MCB.01584-06; PMID: 17130237
- Schneider DA. RNA polymerase I activity is regulated at multiple steps in the transcription cycle: recent insights into factors that influence transcription elongation. Gene 2012; 493:176 - 84; http://dx.doi.org/10.1016/j.gene.2011.08.006; PMID: 21893173
- Cong R, Das S, Bouvet P. The multiple properties and functions of nucleolin. In: Olson MOJ, ed. The Nucleolus, Protein Reviews. New York, NY: Springer, 2011:185-212
- Daniely Y, Dimitrova DD, Borowiec JA. Stress-dependent nucleolin mobilization mediated by p53-nucleolin complex formation. Mol Cell Biol 2002; 22:6014 - 22; http://dx.doi.org/10.1128/MCB.22.16.6014-6022.2002; PMID: 12138209
- Takagi M, Absalon MJ, McLure KG, Kastan MB. Regulation of p53 translation and induction after DNA damage by ribosomal protein L26 and nucleolin. Cell 2005; 123:49 - 63; http://dx.doi.org/10.1016/j.cell.2005.07.034; PMID: 16213212
- Politz JC, Polena I, Trask I, Bazett-Jones DP, Pederson T. A nonribosomal landscape in the nucleolus revealed by the stem cell protein nucleostemin. Mol Biol Cell 2005; 16:3401 - 10; http://dx.doi.org/10.1091/mbc.E05-02-0106; PMID: 15857956
- Bai B, Moore HM, Laiho M. CRM1 and its ribosome export adaptor NMD3 localize to the nucleolus and affect rRNA synthesis. Nucleus 2013; 4:315 - 25; http://dx.doi.org/10.4161/nucl.25342; PMID: 23782956
- Zemp I, Kutay U. Nuclear export and cytoplasmic maturation of ribosomal subunits. FEBS Lett 2007; 581:2783 - 93; http://dx.doi.org/10.1016/j.febslet.2007.05.013; PMID: 17509569
- Panse VG, Johnson AW. Maturation of eukaryotic ribosomes: acquisition of functionality. Trends Biochem Sci 2010; 35:260 - 6; http://dx.doi.org/10.1016/j.tibs.2010.01.001; PMID: 20137954
- Sengupta J, Bussiere C, Pallesen J, West M, Johnson AW, Frank J. Characterization of the nuclear export adaptor protein Nmd3 in association with the 60S ribosomal subunit. J Cell Biol 2010; 189:1079 - 86; http://dx.doi.org/10.1083/jcb.201001124; PMID: 20584915
- Lo D, Lu H. Nucleostemin: Another nucleolar “Twister” of the p53-MDM2 loop. Cell Cycle 2010; 9:3227 - 32; http://dx.doi.org/10.4161/cc.9.16.12605; PMID: 20703089
- Armache JP, Jarasch A, Anger AM, Villa E, Becker T, Bhushan S, Jossinet F, Habeck M, Dindar G, Franckenberg S, et al. Cryo-EM structure and rRNA model of a translating eukaryotic 80S ribosome at 5.5-A resolution. Proc Natl Acad Sci U S A 2010; a 107:19748 - 53; http://dx.doi.org/10.1073/pnas.1009999107; PMID: 20980660
- Armache JP, Jarasch A, Anger AM, Villa E, Becker T, Bhushan S, Jossinet F, Habeck M, Dindar G, Franckenberg S, et al. Localization of eukaryote-specific ribosomal proteins in a 5.5-Å cryo-EM map of the 80S eukaryotic ribosome. Proc Natl Acad Sci U S A 2010; b 107:19754 - 9; http://dx.doi.org/10.1073/pnas.1010005107; PMID: 20974910
- Ben-Shem A, Garreau de Loubresse N, Melnikov S, Jenner L, Yusupova G, Yusupov M. The structure of the eukaryotic ribosome at 3.0 Å resolution. Science 2011; 334:1524 - 9; http://dx.doi.org/10.1126/science.1212642; PMID: 22096102
- Klinge S, Voigts-Hoffmann F, Leibundgut M, Arpagaus S, Ban N. Crystal structure of the eukaryotic 60S ribosomal subunit in complex with initiation factor 6. Science 2011; 334:941 - 8; http://dx.doi.org/10.1126/science.1211204; PMID: 22052974
- Rabl J, Leibundgut M, Ataide SF, Haag A, Ban N. Crystal structure of the eukaryotic 40S ribosomal subunit in complex with initiation factor 1. Science 2011; 331:730 - 6; http://dx.doi.org/10.1126/science.1198308; PMID: 21205638
- Wilson DN, Doudna Cate JH. The structure and function of the eukaryotic ribosome. Cold Spring Harb Perspect Biol 2012; 4:a011536; http://dx.doi.org/10.1101/cshperspect.a011536; PMID: 22550233
- Klinge S, Voigts-Hoffmann F, Leibundgut M, Ban N. Atomic structures of the eukaryotic ribosome. Trends Biochem Sci 2012; 37:189 - 98; http://dx.doi.org/10.1016/j.tibs.2012.02.007; PMID: 22436288
- Rubbi CP, Milner J. Disruption of the nucleolus mediates stabilization of p53 in response to DNA damage and other stresses. EMBO J 2003; 22:6068 - 77; http://dx.doi.org/10.1093/emboj/cdg579; PMID: 14609953
- Kruhlak M, Crouch EE, Orlov M, Montaño C, Gorski SA, Nussenzweig A, Misteli T, Phair RD, Casellas R. The ATM repair pathway inhibits RNA polymerase I transcription in response to chromosome breaks. Nature 2007; 447:730 - 4; http://dx.doi.org/10.1038/nature05842; PMID: 17554310
- Boulon S, Westman BJ, Hutten S, Boisvert F-M, Lamond AI. The nucleolus under stress. Mol Cell 2010; 40:216 - 27; http://dx.doi.org/10.1016/j.molcel.2010.09.024; PMID: 20965417
- Brooks CL, Gu W. p53 ubiquitination: Mdm2 and beyond. Mol Cell 2006; 21:307 - 15; http://dx.doi.org/10.1016/j.molcel.2006.01.020; PMID: 16455486
- Quelle DE, Zindy F, Ashmun RA, Sherr CJ. Alternative reading frames of the INK4a tumor suppressor gene encode two unrelated proteins capable of inducing cell cycle arrest. Cell 1995; 83:993 - 1000; http://dx.doi.org/10.1016/0092-8674(95)90214-7; PMID: 8521522
- Weber JD, Taylor LJ, Roussel MF, Sherr CJ, Bar-Sagi D. Nucleolar Arf sequesters Mdm2 and activates p53. Nat Cell Biol 1999; 1:20 - 6; http://dx.doi.org/10.1038/8991; PMID: 10559859
- Llanos S, Clark PA, Rowe J, Peters G. Stabilization of p53 by p14ARF without relocation of MDM2 to the nucleolus. Nat Cell Biol 2001; 3:445 - 52; http://dx.doi.org/10.1038/35074506; PMID: 11331871
- Argentini M, Barboule N, Wasylyk B. The contribution of the acidic domain of MDM2 to p53 and MDM2 stability. Oncogene 2001; 20:1267 - 75; http://dx.doi.org/10.1038/sj.onc.1204241; PMID: 11313871
- Zhang Y, Wolf GW, Bhat K, Jin A, Allio T, Burkhart WA, Xiong Y. Ribosomal protein L11 negatively regulates oncoprotein MDM2 and mediates a p53-dependent ribosomal-stress checkpoint pathway. Mol Cell Biol 2003; 23:8902 - 12; http://dx.doi.org/10.1128/MCB.23.23.8902-8912.2003; PMID: 14612427
- Kawai H, Wiederschain D, Yuan ZM. Critical contribution of the MDM2 acidic domain to p53 ubiquitination. Mol Cell Biol 2003; 23:4939 - 47; http://dx.doi.org/10.1128/MCB.23.14.4939-4947.2003; PMID: 12832479
- Meulmeester E, Frenk R, Stad R, de Graaf P, Marine J-C, Vousden KH, Jochemsen AG. Critical role for a central part of Mdm2 in the ubiquitylation of p53. Mol Cell Biol 2003; 23:4929 - 38; http://dx.doi.org/10.1128/MCB.23.14.4929-4938.2003; PMID: 12832478
- Sherr CJ. Divorcing ARF and p53: an unsettled case. Nat Rev Cancer 2006; 6:663 - 73; http://dx.doi.org/10.1038/nrc1954; PMID: 16915296
- Savkur RS, Olson MOJ. Preferential cleavage in pre-ribosomal RNA byprotein B23 endoribonuclease. Nucleic Acids Res 1998; 26:4508 - 15; http://dx.doi.org/10.1093/nar/26.19.4508; PMID: 9742256
- Colombo E, Alcalay M, Pelicci PG. Nucleophosmin and its complex network: a possible therapeutic target in hematological diseases. Oncogene 2011; 30:2595 - 609; http://dx.doi.org/10.1038/onc.2010.646; PMID: 21278791
- Yip SP, Siu PM, Leung PHM, Zhao Y, Yung BYM. The multifunctional nucleolar protein nucleophosmin/NPM/B23 and the nucleoplasmin family of proteins. In: Olson MOJ, ed. The Nucleolus, Protein Reviews. New York, NY: Springer, 2011:213-252.
- Brady SN, Yu Y, Maggi LB Jr., Weber JD. ARF impedes NPM/B23 shuttling in an Mdm2-sensitive tumor suppressor pathway. Mol Cell Biol 2004; 24:9327 - 38; http://dx.doi.org/10.1128/MCB.24.21.9327-9338.2004; PMID: 15485902
- Itahana K, Bhat KP, Jin A, Itahana Y, Hawke D, Kobayashi R, Zhang Y. Tumor suppressor ARF degrades B23, a nucleolar protein involved in ribosome biogenesis and cell proliferation. Mol Cell 2003; 12:1151 - 64; http://dx.doi.org/10.1016/S1097-2765(03)00431-3; PMID: 14636574
- Korgaonkar C, Hagen J, Tompkins V, Frazier AA, Allamargot C, Quelle FW, Quelle DE. Nucleophosmin (B23) targets ARF to nucleoli and inhibits its function. Mol Cell Biol 2005; 25:1258 - 71; http://dx.doi.org/10.1128/MCB.25.4.1258-1271.2005; PMID: 15684379
- Colombo E, Bonetti P, Lazzerini Denchi E, Martinelli P, Zamponi R, Marine J-C, Helin K, Falini B, Pelicci PG. Nucleophosmin is required for DNA integrity and p19Arf protein stability. Mol Cell Biol 2005; 25:8874 - 86; http://dx.doi.org/10.1128/MCB.25.20.8874-8886.2005; PMID: 16199867
- Tsai RYL. New frontiers in nucleolar research: nucleostemin and related proteins. In: Olson MOJ, ed. The Nucleolus, Protein Reviews. New York, NY: Springer, 2011:301-320.
- Tsai RYL, McKay RDG. A nucleolar mechanism controlling cell proliferation in stem cells and cancer cells. Genes Dev 2002; 16:2991 - 3003; http://dx.doi.org/10.1101/gad.55671; PMID: 12464630
- Tsai RYL, McKay RDG. A multistep, GTP-driven mechanism controlling the dynamic cycling of nucleostemin. J Cell Biol 2005; 168:179 - 84; http://dx.doi.org/10.1083/jcb.200409053; PMID: 15657390
- Meng L, Yasumoto H, Tsai RYL. Multiple controls regulate nucleostemin partitioning between nucleolus and nucleoplasm. J Cell Sci 2006; 119:5124 - 36; http://dx.doi.org/10.1242/jcs.03292; PMID: 17158916
- Misteli T. Going in GTP cycles in the nucleolus. J Cell Biol 2005; 168:177 - 8; http://dx.doi.org/10.1083/jcb.200412038; PMID: 15657389
- Romanova L, Grand A, Zhang L, Rayner S, Katoku-Kikyo N, Kellner S, Kikyo N. Critical role of nucleostemin in pre-rRNA processing. J Biol Chem 2009; 284:4968 - 77; http://dx.doi.org/10.1074/jbc.M804594200; PMID: 19106111
- Hsu JK, Lin T, Tsai RYL. Nucleostemin prevents telomere damage by promoting PML-IV recruitment to SUMOylated TRF1. J Cell Biol 2012; 197:613 - 24; http://dx.doi.org/10.1083/jcb.201109038; PMID: 22641345
- Meng L, Lin T, Peng G, Hsu JK, Lee S, Lin SY, Tsai RYL. Nucleostemin deletion reveals an essential mechanism that maintains the genomic stability of stem and progenitor cells. Proc Natl Acad Sci U S A 2013; 110:11415 - 20; http://dx.doi.org/10.1073/pnas.1301672110; PMID: 23798389
- Lin T, Meng L, Lin T-C, Wu LJ, Pederson T, Tsai RYL. Nucleostemin and GNL3L exercise distinct functions in genome protection and ribosome synthesis, respectively. J Cell Sci 2014; 127:2302 - 12; http://dx.doi.org/10.1242/jcs.143842; PMID: 24610951
- Du X, Rao MR, Chen XQ, Wu W, Mahalingam S, Balasundaram D. The homologous putative GTPases Grn1p from fission yeast and the human GNL3L are required for growth and play a role in processing of nucleolar pre-rRNA. Mol Biol Cell 2006; 17:460 - 74; http://dx.doi.org/10.1091/mbc.E05-09-0848; PMID: 16251348
- Kudron MM, Reinke V. C. elegans nucleostemin is required for larval growth and germline stem cell division. PLoS Genet 2008; 4:e1000181; http://dx.doi.org/10.1371/journal.pgen.1000181; PMID: 18725931
- Rosby R, Cui Z, Rogers E, deLivron MA, Robinson VL, DiMario PJ. Knockdown of the Drosophila GTPase nucleostemin 1 impairs large ribosomal subunit biogenesis, cell growth, and midgut precursor cell maintenance. Mol Biol Cell 2009; 20:4424 - 34; http://dx.doi.org/10.1091/mbc.E08-06-0592; PMID: 19710426
- Dai M-S, Sun X-X, Lu H. Aberrant expression of nucleostemin activates p53 and induces cell cycle arrest via inhibition of MDM2. Mol Cell Biol 2008; 28:4365 - 76; http://dx.doi.org/10.1128/MCB.01662-07; PMID: 18426907
- Ma H, Pederson T. Depletion of the nucleolar protein nucleostemin causes G1 cell cycle arrest via the p53 pathway. Mol Biol Cell 2007; 18:2630 - 5; http://dx.doi.org/10.1091/mbc.E07-03-0244; PMID: 17494866
- Lo D, Dai MS, Sun XX, Zeng SX, Lu H. Ubiquitin- and MDM2 E3 ligase-independent proteasomal turnover of nucleostemin in response to GTP depletion. J Biol Chem 2012; 287:10013 - 20; http://dx.doi.org/10.1074/jbc.M111.335141; PMID: 22318725
- Ma H, Pederson T. Nucleostemin: a multiplex regulator of cell-cycle progression. Trends Cell Biol 2008; 18:575 - 9; http://dx.doi.org/10.1016/j.tcb.2008.09.003; PMID: 18951797
- Avitabile D, Bailey B, Cottage CT, Sundararaman B, Joyo A, McGregor M, Gude N, Truffa S, Zarrabi A, Konstandin M, et al. Nucleolar stress is an early response to myocardial damage involving nucleolar proteins nucleostemin and nucleophosmin. Proc Natl Acad Sci U S A 2011; 108:6145 - 50; http://dx.doi.org/10.1073/pnas.1017935108; PMID: 21444791
- Hariharan N, Sussman MA. Stressing on the nucleolus in cardiovascular disease. Biochim Biophys Acta 2014; 1842:798 - 801; http://dx.doi.org/10.1016/j.bbadis.2013.09.016; PMID: 24514103
- Zhang Y, Lu H. Signaling to p53: ribosomal proteins find their way. Cancer Cell 2009; 16:369 - 77; http://dx.doi.org/10.1016/j.ccr.2009.09.024; PMID: 19878869
- Warner JR, McIntosh KB. How common are extraribosomal functions of ribosomal proteins?. Mol Cell 2009; 34:3 - 11; http://dx.doi.org/10.1016/j.molcel.2009.03.006; PMID: 19362532
- Yadavilli S, Mayo LD, Higgins M, Lain S, Hegde V, Deutsch WA. Ribosomal protein S3: A multi-functional protein that interacts with both p53 and MDM2 through its KH domain. DNA Repair (Amst) 2009; 8:1215 - 24; http://dx.doi.org/10.1016/j.dnarep.2009.07.003; PMID: 19656744
- Chen D, Zhang Z, Li M, Wang W, Li Y, Rayburn ER, Hill DL, Wang H, Zhang R. Ribosomal protein S7 as a novel modulator of p53-MDM2 interaction: binding to MDM2, stabilization of p53 protein, and activation of p53 function. Oncogene 2007; 26:5029 - 37; http://dx.doi.org/10.1038/sj.onc.1210327; PMID: 17310983
- Zhu YH, Zhang CW, Lu L, Demidov ON, Sun L, Yang L, Bulavin DV, Xiao ZC. Wip1 regulates the generation of new neural cells in the adult olfactory bulb through p53-dependent cell cycle control. Stem Cells 2009; 27:1433 - 42; http://dx.doi.org/10.1002/stem.65; PMID: 19489034
- Marechal V, Elenbaas B, Piette J, Nicolas J-C, Levine AJ. The ribosomal L5 protein is associated with mdm-2 and mdm-2-p53 complexes. Mol Cell Biol 1994; 14:7414 - 20; PMID: 7935455
- Dai M-S, Lu H. Inhibition of MDM2-mediated p53 ubiquitination and degradation by ribosomal protein L5. J Biol Chem 2004; 279:44475 - 82; http://dx.doi.org/10.1074/jbc.M403722200; PMID: 15308643
- Bursać S, Brdovčak MC, Pfannkuchen M, Orsolić I, Golomb L, Zhu Y, Katz C, Daftuar L, Grabušić K, Vukelić I, et al. Mutual protection of ribosomal proteins L5 and L11 from degradation is essential for p53 activation upon ribosomal biogenesis stress. Proc Natl Acad Sci U S A 2012; 109:20467 - 72; http://dx.doi.org/10.1073/pnas.1218535109; PMID: 23169665
- Lohrum MAE, Ludwig RL, Kubbutat MHG, Hanlon M, Vousden KH. Regulation of HDM2 activity by the ribosomal protein L11. Cancer Cell 2003; 3:577 - 87; http://dx.doi.org/10.1016/S1535-6108(03)00134-X; PMID: 12842086
- Bhat KP, Itahana K, Jin A, Zhang Y. Essential role of ribosomal protein L11 in mediating growth inhibition-induced p53 activation. EMBO J 2004; 23:2402 - 12; http://dx.doi.org/10.1038/sj.emboj.7600247; PMID: 15152193
- Fumagalli S, Di Cara A, Neb-Gulati A, Natt F, Schwemberger S, Hall J, Babcock GF, Bernardi R, Pandolfi PP, Thomas G. Absence of nucleolar disruption after impairment of 40S ribosome biogenesis reveals an rpL11-translation-dependent mechanism of p53 induction. Nat Cell Biol 2009; 11:501 - 8; http://dx.doi.org/10.1038/ncb1858; PMID: 19287375
- Dai M-S, Zeng SX, Jin Y, Sun X-X, David L, Lu H. Ribosomal protein L23 activates p53 by inhibiting MDM2 function in response to ribosomal perturbation but not to translation inhibition. Mol Cell Biol 2004; 24:7654 - 68; http://dx.doi.org/10.1128/MCB.24.17.7654-7668.2004; PMID: 15314173
- Warner JR. In the absence of ribosomal RNA synthesis, the ribosomal proteins of HeLa cells are synthesized normally and degraded rapidly. J Mol Biol 1977; 115:315 - 33; http://dx.doi.org/10.1016/0022-2836(77)90157-7; PMID: 592369
- Lam YW, Lamond AI, Mann M, Andersen JS. Analysis of nucleolar protein dynamics reveals the nuclear degradation of ribosomal proteins. Curr Biol 2007; 17:749 - 60; http://dx.doi.org/10.1016/j.cub.2007.03.064; PMID: 17446074
- Andersen JS, Lam YW, Leung AKL, Ong SE, Lyon CE, Lamond AI, Mann M. Nucleolar proteome dynamics. Nature 2005; 433:77 - 83; http://dx.doi.org/10.1038/nature03207; PMID: 15635413
- Sloan KE, Bohnsack MT, Watkins NJ. The 5S RNP couples p53 homeostasis to ribosome biogenesis and nucleolar stress. Cell Rep 2013; 5:237 - 47; http://dx.doi.org/10.1016/j.celrep.2013.08.049; PMID: 24120868
- Donati G, Peddigari S, Mercer CA, Thomas G. 5S ribosomal RNA is an essential component of a nascent ribosomal precursor complex that regulates the Hdm2-p53 checkpoint. Cell Rep 2013; 4:87 - 98; http://dx.doi.org/10.1016/j.celrep.2013.05.045; PMID: 23831031
- Burger K, Mühl B, Harasim T, Rohrmoser M, Malamoussi A, Orban M, Kellner M, Gruber-Eber A, Kremmer E, Hölzel M, et al. Chemotherapeutic drugs inhibit ribosome biogenesis at various levels. J Biol Chem 2010; 285:12416 - 25; http://dx.doi.org/10.1074/jbc.M109.074211; PMID: 20159984
- Andrews WJ, Panova T, Normand C, Gadal O, Tikhonova IG, Panov KI. Old drug, new target: ellipticines selectively inhibit RNA polymerase I transcription. J Biol Chem 2013; 288:4567 - 82; http://dx.doi.org/10.1074/jbc.M112.411611; PMID: 23293027
- Drygin D, Siddiqui-Jain A, O’Brien S, Schwaebe M, Lin A, Bliesath J, Ho CB, Proffitt C, Trent K, Whitten JP, et al. Anticancer activity of CX-3543: a direct inhibitor of rRNA biogenesis. Cancer Res 2009; 69:7653 - 61; http://dx.doi.org/10.1158/0008-5472.CAN-09-1304; PMID: 19738048
- Drygin D, Lin A, Bliesath J, Ho CB, O’Brien SE, Proffitt C, Omori M, Haddach M, Schwaebe MK, Siddiqui-Jain A, et al. Targeting RNA polymerase I with an oral small molecule CX-5461 inhibits ribosomal RNA synthesis and solid tumor growth. Cancer Res 2011; 71:1418 - 30; http://dx.doi.org/10.1158/0008-5472.CAN-10-1728; PMID: 21159662
- Welch WJ, Suhan JP. Morphological study of the mammalian stress response: characterization of changes in cytoplasmic organelles, cytoskeleton, and nucleoli, and appearance of intranuclear actin filaments in rat fibroblasts after heat-shock treatment. J Cell Biol 1985; 101:1198 - 211; http://dx.doi.org/10.1083/jcb.101.4.1198; PMID: 3900086
- Liu Y, Liang S, Tartakoff AM. Heat shock disassembles the nucleolus and inhibits nuclear protein import and poly(A)+ RNA export. EMBO J 1996; 15:6750 - 7; PMID: 8978700
- Názer E, Verdún RE, Sánchez DO. Severe heat shock induces nucleolar accumulation of mRNAs in Trypanosoma cruzi.. PLoS One 2012; 7:e43715; http://dx.doi.org/10.1371/journal.pone.0043715; PMID: 22952745
- Mekhail K, Rivero-Lopez L, Khacho M, Lee S. Restriction of rRNA synthesis by VHL maintains energy equilibrium under hypoxia. Cell Cycle 2006; 5:2401 - 13; http://dx.doi.org/10.4161/cc.5.20.3387; PMID: 17102617
- Zatsepina OV, Dudnic OA, Todorov IT, Thiry M, Spring H, Trendelenburg MF. Experimental induction of prenucleolar bodies (PNBs) in interphase cells: interphase PNBs show similar characteristics as those typically observed at telophase of mitosis in untreated cells. Chromosoma 1997; 105:418 - 30; http://dx.doi.org/10.1007/BF02510478; PMID: 9211969
- Cioce M, Boulon S, Matera AG, Lamond AI. UV-induced fragmentation of Cajal bodies. J Cell Biol 2006; 175:401 - 13; http://dx.doi.org/10.1083/jcb.200604099; PMID: 17088425
- Xiao L, Grove A. Coordination of Ribosomal Protein and Ribosomal RNA Gene Expression in Response to TOR Signaling. Curr Genomics 2009; 10:198 - 205; http://dx.doi.org/10.2174/138920209788185261; PMID: 19881913
- Tsang CK, Bertram PG, Ai W, Drenan R, Zheng XFS. Chromatin-mediated regulation of nucleolar structure and RNA Pol I localization by TOR. EMBO J 2003; 22:6045 - 56; http://dx.doi.org/10.1093/emboj/cdg578; PMID: 14609951
- Narla A, Ebert BL. Ribosomopathies: human disorders of ribosome dysfunction. Blood 2010; 115:3196 - 205; http://dx.doi.org/10.1182/blood-2009-10-178129; PMID: 20194897
- Armistead J, Triggs-Raine B. Diverse diseases from a ubiquitous process: the ribosomopathy paradox. FEBS Lett 2014; 588:1491 - 500; http://dx.doi.org/10.1016/j.febslet.2014.03.024; PMID: 24657617
- Dixon J, Jones NC, Sandell LL, Jayasinghe SM, Crane J, Rey J-P, Dixon MJ, Trainor PA. Tcof1/Treacle is required for neural crest cell formation and proliferation deficiencies that cause craniofacial abnormalities. Proc Natl Acad Sci U S A 2006; 103:13403 - 8; http://dx.doi.org/10.1073/pnas.0603730103; PMID: 16938878
- Sakai D, Trainor PA. Treacher Collins syndrome: unmasking the role of Tcof1/treacle. Int J Biochem Cell Biol 2009; 41:1229 - 32; http://dx.doi.org/10.1016/j.biocel.2008.10.026; PMID: 19027870
- Dixon J, Edwards SJ, Anderson I, Brass A, Scambler PJ, Dixon MJ. Identification of the complete coding sequence and genomic organization of the Treacher Collins syndrome gene. Genome Res 1997; 7:223 - 34; http://dx.doi.org/10.1101/gr.7.3.223; PMID: 9074926
- He F, DiMario P. Structure and function of Nopp140 and Treacle. Chapter 11, The Nucleolus. Springer, 2011.
- Lin C-I, Yeh N-H. Treacle recruits RNA polymerase I complex to the nucleolus that is independent of UBF. Biochem Biophys Res Commun 2009; 386:396 - 401; http://dx.doi.org/10.1016/j.bbrc.2009.06.050; PMID: 19527688
- Gonzales B, Henning D, So RB, Dixon J, Dixon MJ, Valdez BC. The Treacher Collins syndrome (TCOF1) gene product is involved in pre-rRNA methylation. Hum Mol Genet 2005; 14:2035 - 43; http://dx.doi.org/10.1093/hmg/ddi208; PMID: 15930015
- Jones NC, Lynn ML, Gaudenz K, Sakai D, Aoto K, Rey J-P, Glynn EF, Ellington L, Du C, Dixon J, et al. Prevention of the neurocristopathy Treacher Collins syndrome through inhibition of p53 function. Nat Med 2008; 14:125 - 33; http://dx.doi.org/10.1038/nm1725; PMID: 18246078
- Dauwerse JG, Dixon J, Seland S, Ruivenkamp CAL, van Haeringen A, Hoefsloot LH, Peters DJ, Boers AC, Daumer-Haas C, Maiwald R, et al. Mutations in genes encoding subunits of RNA polymerases I and III cause Treacher Collins syndrome. Nat Genet 2011; 43:20 - 2; http://dx.doi.org/10.1038/ng.724; PMID: 21131976
- Ellis SR. Nucleolar stress in Diamond Blackfan anemia pathophysiology. Biochim Biophys Acta 2014; 1842:765 - 8; http://dx.doi.org/10.1016/j.bbadis.2013.12.013; PMID: 24412987
- Mitchell JR, Wood E, Collins K. A telomerase component is defective in the human disease dyskeratosis congenita. Nature 1999; 402:551 - 5; http://dx.doi.org/10.1038/990141; PMID: 10591218
- Rashid R, Liang B, Baker DL, Youssef OA, He Y, Phipps K, Terns RM, Terns MP, Li H. Crystal structure of a Cbf5-Nop10-Gar1 complex and implications in RNA-guided pseudouridylation and dyskeratosis congenita. Mol Cell 2006; 21:249 - 60; http://dx.doi.org/10.1016/j.molcel.2005.11.017; PMID: 16427014
- Thumati NR, Zeng X-L, Au HHT, Jang CJ, Jan E, Wong JMY. Severity of X-linked dyskeratosis congenita (DKCX) cellular defects is not directly related to dyskerin (DKC1) activity in ribosomal RNA biogenesis or mRNA translation. Hum Mutat 2013; 34:1698 - 707; http://dx.doi.org/10.1002/humu.22447; PMID: 24115260
- Ruggero D, Grisendi S, Piazza F, Rego E, Mari F, Rao PH, Cordon-Cardo C, Pandolfi PP. Dyskeratosis congenita and cancer in mice deficient in ribosomal RNA modification. Science 2003; 299:259 - 62; http://dx.doi.org/10.1126/science.1079447; PMID: 12522253
- Mochizuki T, Miyazaki H, Hara T, Furuya T, Imamura T, Watabe T, Miyazono K. Roles for the MH2 domain of Smad7 in the specific inhibition of transforming growth factor-beta superfamily signaling. J Biol Chem 2004; 279:31568 - 74; http://dx.doi.org/10.1074/jbc.M313977200; PMID: 15148321
- Marrone A, Mason PJ. Dyskeratosis congenita. Cell Mol Life Sci 2003; 60:507 - 17; http://dx.doi.org/10.1007/s000180300042; PMID: 12737310
- Yamaguchi H, Calado RT, Ly H, Kajigaya S, Baerlocher GM, Chanock SJ, Lansdorp PM, Young NS. Mutations in TERT, the gene for telomerase reverse transcriptase, in aplastic anemia. N Engl J Med 2005; 352:1413 - 24; http://dx.doi.org/10.1056/NEJMoa042980; PMID: 15814878
- Vulliamy T, Marrone A, Goldman F, Dearlove A, Bessler M, Mason PJ, Dokal I. The RNA component of telomerase is mutated in autosomal dominant dyskeratosis congenita. Nature 2001; 413:432 - 5; http://dx.doi.org/10.1038/35096585; PMID: 11574891
- Marrone A, Walne A, Tamary H, Masunari Y, Kirwan M, Beswick R, Vulliamy T, Dokal I. Telomerase reverse-transcriptase homozygous mutations in autosomal recessive dyskeratosis congenita and Hoyeraal-Hreidarsson syndrome. Blood 2007; 110:4198 - 205; http://dx.doi.org/10.1182/blood-2006-12-062851; PMID: 17785587
- Pereboom TC, van Weele LJ, Bondt A, MacInnes AW. A zebrafish model of dyskeratosis congenita reveals hematopoietic stem cell formation failure resulting from ribosomal protein-mediated p53 stabilization. Blood 2011; 118:5458 - 65; http://dx.doi.org/10.1182/blood-2011-04-351460; PMID: 21921046
- Gazda HT, Preti M, Sheen MR, O’Donohue MF, Vlachos A, Davies SM, Kattamis A, Doherty L, Landowski M, Buros C, et al. Frameshift mutation in p53 regulator RPL26 is associated with multiple physical abnormalities and a specific pre-ribosomal RNA processing defect in Diamond-Blackfan anemia. Hum Mutat 2012; 33:1037 - 44; http://dx.doi.org/10.1002/humu.22081; PMID: 22431104
- Vlachos A, Dahl N, Dianzani I, Lipton JM. Clinical utility gene card for: Diamond-Blackfan anemia--update 2013. Eur J Hum Genet 2013; 21:21; http://dx.doi.org/10.1038/ejhg.2013.34; PMID: 23463023
- Farrar JE, Vlachos A, Atsidaftos E, Carlson-Donohoe H, Markello TC, Arceci RJ, Ellis SR, Lipton JM, Bodine DM. Ribosomal protein gene deletions in Diamond-Blackfan anemia. Blood 2011; 118:6943 - 51; http://dx.doi.org/10.1182/blood-2011-08-375170; PMID: 22045982
- Landowski M, O’Donohue MF, Buros C, Ghazvinian R, Montel-Lehry N, Vlachos A, Sieff CA, Newburger PE, Niewiadomska E, Matysiak M, et al. Novel deletion of RPL15 identified by array-comparative genomic hybridization in Diamond-Blackfan anemia. Hum Genet 2013; 132:1265 - 74; http://dx.doi.org/10.1007/s00439-013-1326-z; PMID: 23812780
- Lipton JM, Ellis SR. Diamond-Blackfan anemia: diagnosis, treatment, and molecular pathogenesis. Hematol Oncol Clin North Am 2009; 23:261 - 82; http://dx.doi.org/10.1016/j.hoc.2009.01.004; PMID: 19327583
- Perdahl EB, Naprstek BL, Wallace WC, Lipton JM. Erythroid failure in Diamond-Blackfan anemia is characterized by apoptosis. Blood 1994; 83:645 - 50; PMID: 8298126
- Dutt S, Narla A, Lin K, Mullally A, Abayasekara N, Megerdichian C, Wilson FH, Currie T, Khanna-Gupta A, Berliner N, et al. Haploinsufficiency for ribosomal protein genes causes selective activation of p53 in human erythroid progenitor cells. Blood 2011; 117:2567 - 76; http://dx.doi.org/10.1182/blood-2010-07-295238; PMID: 21068437
- Jaako P, Flygare J, Olsson K, Quere R, Ehinger M, Henson A, Ellis S, Schambach A, Baum C, Richter J, et al. Mice with ribosomal protein S19 deficiency develop bone marrow failure and symptoms like patients with Diamond-Blackfan anemia. Blood 2011; 118:6087 - 96; http://dx.doi.org/10.1182/blood-2011-08-371963; PMID: 21989989
- Chakraborty A, Uechi T, Higa S, Torihara H, Kenmochi N. Loss of ribosomal protein L11 affects zebrafish embryonic development through a p53-dependent apoptotic response. PLoS One 2009; 4:e4152; http://dx.doi.org/10.1371/journal.pone.0004152; PMID: 19129914
- Danilova N, Sakamoto KM, Lin S. Ribosomal protein L11 mutation in zebrafish leads to haematopoietic and metabolic defects. Br J Haematol 2011; 152:217 - 28; http://dx.doi.org/10.1111/j.1365-2141.2010.08396.x; PMID: 21114664
- Taylor AM, Humphries JM, White RM, Murphey RD, Burns CE, Zon LI. Hematopoietic defects in rps29 mutant zebrafish depend upon p53 activation. Exp Hematol 2012; 40:228 - 37, e5; http://dx.doi.org/10.1016/j.exphem.2011.11.007; PMID: 22120640
- Torihara H, Uechi T, Chakraborty A, Shinya M, Sakai N, Kenmochi N. Erythropoiesis failure due to RPS19 deficiency is independent of an activated Tp53 response in a zebrafish model of Diamond-Blackfan anaemia. Br J Haematol 2011; 152:648 - 54; http://dx.doi.org/10.1111/j.1365-2141.2010.08535.x; PMID: 21223253
- Yadav GV, Chakraborty A, Uechi T, Kenmochi N. Ribosomal protein deficiency causes Tp53-independent erythropoiesis failure in zebrafish. Int J Biochem Cell Biol 2014; 49:1 - 7; http://dx.doi.org/10.1016/j.biocel.2014.01.006; PMID: 24417973
- Ebert BL, Pretz J, Bosco J, Chang CY, Tamayo P, Galili N, Raza A, Root DE, Attar E, Ellis SR, et al. Identification of RPS14 as a 5q- syndrome gene by RNA interference screen. Nature 2008; 451:335 - 9; http://dx.doi.org/10.1038/nature06494; PMID: 18202658
- Ebert BL. Deletion 5q in myelodysplastic syndrome: a paradigm for the study of hemizygous deletions in cancer. Leukemia 2009; 23:1252 - 6; http://dx.doi.org/10.1038/leu.2009.53; PMID: 19322210
- Boocock GRB, Morrison JA, Popovic M, Richards N, Ellis L, Durie PR, Rommens JM. Mutations in SBDS are associated with Shwachman-Diamond syndrome. Nat Genet 2003; 33:97 - 101; http://dx.doi.org/10.1038/ng1062; PMID: 12496757
- Johnson AW, Ellis SR. Of blood, bones, and ribosomes: is Swachman-Diamond syndrome a ribosomopathy?. Genes Dev 2011; 25:898 - 900; http://dx.doi.org/10.1101/gad.2053011; PMID: 21536731
- Finch AJ, Hilcenko C, Basse N, Drynan LF, Goyenechea B, Menne TF, González Fernández A, Simpson P, D’Santos CS, Arends MJ, et al. Uncoupling of GTP hydrolysis from eIF6 release on the ribosome causes Shwachman-Diamond syndrome. Genes Dev 2011; 25:917 - 29; http://dx.doi.org/10.1101/gad.623011; PMID: 21536732
- Rujkijyanont P, Adams S-L, Beyene J, Dror Y. Bone marrow cells from patients with Shwachman-Diamond syndrome abnormally express genes involved in ribosome biogenesis and RNA processing. Br J Haematol 2009; 145:806 - 15; http://dx.doi.org/10.1111/j.1365-2141.2009.07692.x; PMID: 19438500
- Ganapathi KA, Austin KM, Lee C-S, Dias A, Malsch MM, Reed R, Shimamura A. The human Shwachman-Diamond syndrome protein, SBDS, associates with ribosomal RNA. Blood 2007; 110:1458 - 65; http://dx.doi.org/10.1182/blood-2007-02-075184; PMID: 17475909
- Ridanpää M, van Eenennaam H, Pelin K, Chadwick R, Johnson C, Yuan B, vanVenrooij W, Pruijn G, Salmela R, Rockas S, et al. Mutations in the RNA component of RNase MRP cause a pleiotropic human disease, cartilage-hair hypoplasia. Cell 2001; 104:195 - 203; http://dx.doi.org/10.1016/S0092-8674(01)00205-7; PMID: 11207361
- Lygerou Z, Allmang C, Tollervey D, Séraphin B. Accurate processing of a eukaryotic precursor ribosomal RNA by ribonuclease MRP in vitro. Science 1996; 272:268 - 70; http://dx.doi.org/10.1126/science.272.5259.268; PMID: 8602511
- van Eenennaam H, Jarrous N, van Venrooij WJ, Pruijn GJM. Architecture and function of the human endonucleases RNase P and RNase MRP. IUBMB Life 2000; 49:265 - 72; http://dx.doi.org/10.1080/15216540050033113; PMID: 10995027
- von Kobbe C, Bohr VA. A nucleolar targeting sequence in the Werner syndrome protein resides within residues 949-1092. J Cell Sci 2002; 115:3901 - 7; http://dx.doi.org/10.1242/jcs.00076; PMID: 12244128
- Rossi ML, Ghosh AK, Bohr VA. Roles of Werner syndrome protein in protection of genome integrity. DNA Repair (Amst) 2010; 9:331 - 44; http://dx.doi.org/10.1016/j.dnarep.2009.12.011; PMID: 20075015
- Shiratori M, Suzuki T, Itoh C, Goto M, Furuichi Y, Matsumoto T. WRN helicase accelerates the transcription of ribosomal RNA as a component of an RNA polymerase I-associated complex. Oncogene 2002; 21:2447 - 54; http://dx.doi.org/10.1038/sj.onc.1205334; PMID: 11971179
- Machwe A, Orren DK, Bohr VA. Accelerated methylation of ribosomal RNA genes during the cellular senescence of Werner syndrome fibroblasts. FASEB J 2000; 14:1715 - 24; http://dx.doi.org/10.1096/fj.99-0926com; PMID: 10973920
- Ellis NA, German J. Molecular genetics of Bloom’s syndrome. Hum Mol Genet 1996; 5:1457 - 63; PMID: 8875252
- Yankiwski V, Marciniak RA, Guarente L, Neff NF. Nuclear structure in normal and Bloom syndrome cells. Proc Natl Acad Sci U S A 2000; 97:5214 - 9; http://dx.doi.org/10.1073/pnas.090525897; PMID: 10779560
- Grierson PM, Lillard K, Behbehani GK, Combs KA, Bhattacharyya S, Acharya S, Groden J. BLM helicase facilitates RNA polymerase I-mediated ribosomal RNA transcription. Hum Mol Genet 2012; 21:1172 - 83; http://dx.doi.org/10.1093/hmg/ddr545; PMID: 22106380
- Grierson PM, Acharya S, Groden J. Collaborating functions of BLM and DNA topoisomerase I in regulating human rDNA transcription. Mutat Res 2013; 743-744:89 - 96; http://dx.doi.org/10.1016/j.mrfmmm.2012.12.002; PMID: 23261817
- Tikoo S, Madhavan V, Hussain M, Miller ES, Arora P, Zlatanou A, Modi P, Townsend K, Stewart GS, Sengupta S. Ubiquitin-dependent recruitment of the Bloom syndrome helicase upon replication stress is required to suppress homologous recombination. EMBO J 2013; 32:1778 - 92; http://dx.doi.org/10.1038/emboj.2013.117; PMID: 23708797
- Sondalle SB, Baserga SJ. Human diseases of the SSU processome. Biochim Biophys Acta 2014; 1842:758 - 64; http://dx.doi.org/10.1016/j.bbadis.2013.11.004; PMID: 24240090
- Hetman M, Pietrzak M. Emerging roles of the neuronal nucleolus. Trends Neurosci 2012; 35:305 - 14; http://dx.doi.org/10.1016/j.tins.2012.01.002; PMID: 22305768
- Parlato R, Liss B. How Parkinson’s disease meets nucleolar stress. Biochim Biophys Acta 2014; 1842:791 - 7; http://dx.doi.org/10.1016/j.bbadis.2013.12.014; PMID: 24412806
- Tsoi H, Chan HYE. Expression of expanded CAG transcripts triggers nucleolar stress in Huntington’s disease. Cerebellum 2013; 12:310 - 2; http://dx.doi.org/10.1007/s12311-012-0447-6; PMID: 23315009
- Tsoi H, Chan HYE. Roles of the nucleolus in the CAG RNA-mediated toxicity. Biochim Biophys Acta 2014; 1842:779 - 84; http://dx.doi.org/10.1016/j.bbadis.2013.11.015; PMID: 24269666
- Pietrzak M, Rempala G, Nelson PT, Zheng J-J, Hetman M. Epigenetic silencing of nucleolar rRNA genes in Alzheimer’s disease. PLoS One 2011; 6:e22585; http://dx.doi.org/10.1371/journal.pone.0022585; PMID: 21799908
- Li S, Hu GF. Angiogenin-mediated rRNA transcription in cancer and neurodegeneration. Int J Biochem Mol Biol 2010; 1:26 - 35; PMID: 20827423
- Rieker C, Engblom D, Kreiner G, Domanskyi A, Schober A, Stotz S, Neumann M, Yuan X, Grummt I, Schütz G, et al. Nucleolar disruption in dopaminergic neurons leads to oxidative damage and parkinsonism through repression of mammalian target of rapamycin signaling. J Neurosci 2011; 31:453 - 60; http://dx.doi.org/10.1523/JNEUROSCI.0590-10.2011; PMID: 21228155
- Vilotti S, Codrich M, Dal Ferro M, Pinto M, Ferrer I, Collavin L, Gustincich S, Zucchelli S. Parkinson’s disease DJ-1 L166P alters rRNA biogenesis by exclusion of TTRAP from the nucleolus and sequestration into cytoplasmic aggregates via TRAF6. PLoS One 2012; 7:e35051; http://dx.doi.org/10.1371/journal.pone.0035051; PMID: 22532838
- Lee C-C, Tsai Y-T, Kao C-W, Lee L-W, Lai H-J, Ma T-H, Chang YS, Yeh NH, Lo SJ. Mutation of a Nopp140 gene dao-5 alters rDNA transcription and increases germ cell apoptosis in C. elegans. Cell Death Dis 2014; 5:e1158; http://dx.doi.org/10.1038/cddis.2014.114; PMID: 24722283
- Fuhrman LE, Goel AK, Smith J, Shianna KV, Aballay A. Nucleolar proteins suppress Caenorhabditis elegans innate immunity by inhibiting p53/CEP-1. PLoS Genet 2009; 5:e1000657; http://dx.doi.org/10.1371/journal.pgen.1000657; PMID: 19763173
- Morgan TH, Bridges CB, Sturtevant AH. The genetics of Drosophila.. Bibliographica Genetica 1925; 2:1 - 262
- Ritossa F. The bobbed locus. In Ashburner M, Novitski E, eds. The Genetics and Biology of Drosophila. London: Academic Press, 1976: 1b:801-846.
- Lambertsson A. The minute genes in Drosophila and their molecular functions. Adv Genet 1998; 38:69 - 134; http://dx.doi.org/10.1016/S0065-2660(08)60142-X; PMID: 9677706
- Marygold SJ, Roote J, Reuter G, Lambertsson A, Ashburner M, Millburn GH, Harrison PM, Yu Z, Kenmochi N, Kaufman TC, et al. The ribosomal protein genes and Minute loci of Drosophila melanogaster.. Genome Biol 2007; 8:R216; http://dx.doi.org/10.1186/gb-2007-8-10-r216; PMID: 17927810
- Giordano E, Peluso I, Senger S, Furia M. minifly, a Drosophila gene required for ribosome biogenesis. J Cell Biol 1999; 144:1123 - 33; http://dx.doi.org/10.1083/jcb.144.6.1123; PMID: 10087258
- Perrin L, Demakova O, Fanti L, Kallenbach S, Saingery S, Mal’ceva NI, Pimpinelli S, Zhimulev I, Pradel J. Dynamics of the sub-nuclear distribution of Modulo and the regulation of position-effect variegation by nucleolus in Drosophila.. J Cell Sci 1998; 111:2753 - 61; PMID: 9718368
- Perrin L, Romby P, Laurenti P, Bérenger H, Kallenbach S, Bourbon H-M, Pradel J. The Drosophila modifier of variegation modulo gene product binds specific RNA sequences at the nucleolus and interacts with DNA and chromatin in a phosphorylation-dependent manner. J Biol Chem 1999; 274:6315 - 23; http://dx.doi.org/10.1074/jbc.274.10.6315; PMID: 10037720
- Perrin L, Benassayag C, Morello D, Pradel J, Montagne J. Modulo is a target of Myc selectively required for growth of proliferative cells in Drosophila.. Mech Dev 2003; 120:645 - 55; http://dx.doi.org/10.1016/S0925-4773(03)00049-2; PMID: 12834864
- Marinho J, Casares F, Pereira PS. The Drosophila Nol12 homologue viriato is a dMyc target that regulates nucleolar architecture and is required for dMyc-stimulated cell growth. Development 2011; 138:349 - 57; http://dx.doi.org/10.1242/dev.054411; PMID: 21177347
- Chan HYE, Brogna S, O’Kane CJ. Dribble, the Drosophila KRR1p homologue, is involved in rRNA processing. Mol Biol Cell 2001; 12:1409 - 19; http://dx.doi.org/10.1091/mbc.12.5.1409; PMID: 11359931
- Hofmann A, Brünner M, Schwendemann A, Strödicke M, Karberg S, Klebes A, Saumweber H, Korge G. The winged-helix transcription factor JUMU regulates development, nucleolus morphology and function, and chromatin organization of Drosophila melanogaster.. Chromosome Res 2010; 18:307 - 24; http://dx.doi.org/10.1007/s10577-010-9118-y; PMID: 20213139
- Zaffran S, Chartier A, Gallant P, Astier M, Arquier N, Doherty D, Gratecos D, Sémériva M. A Drosophila RNA helicase gene, pitchoune, is required for cell growth and proliferation and is a potential target of d-Myc. Development 1998; 125:3571 - 84; PMID: 9716523
- Cui Z, DiMario PJ. RNAi knockdown of Nopp140 induces Minute-like phenotypes in Drosophila.. Mol Biol Cell 2007; 18:2179 - 91; http://dx.doi.org/10.1091/mbc.E07-01-0074; PMID: 17392509
- James A, Cindass R Jr., Mayer D, Terhoeve S, Mumphrey C, DiMario P. Nucleolar stress in Drosophila melanogaster: RNAi-mediated depletion of Nopp140. Nucleus 2013; 4:123 - 33; http://dx.doi.org/10.4161/nucl.23944; PMID: 23412656
- Kauffman T, Tran J, DiNardo S. Mutations in Nop60B, the Drosophila homolog of human dyskeratosis congenita 1, affect the maintenance of the germ-line stem cell lineage during spermatogenesis. Dev Biol 2003; 253:189 - 99; http://dx.doi.org/10.1016/S0012-1606(02)00013-1; PMID: 12645924
- Tortoriello G, de Celis JF, Furia M. Linking pseudouridine synthases to growth, development and cell competition. FEBS J 2010; 277:3249 - 63; http://dx.doi.org/10.1111/j.1742-4658.2010.07731.x; PMID: 20608977
- Chen C-C, Greene E, Bowers SR, Mellone BG. A role for the CAL1-partner Modulo in centromere integrity and accurate chromosome segregation in Drosophila.. PLoS One 2012; 7:e45094; http://dx.doi.org/10.1371/journal.pone.0045094; PMID: 23028777
- Grewal SS, Li L, Orian A, Eisenman RN, Edgar BA. Myc-dependent regulation of ribosomal RNA synthesis during Drosophila development. Nat Cell Biol 2005; 7:295 - 302; http://dx.doi.org/10.1038/ncb1223; PMID: 15723055
- Tafforeau L, Zorbas C, Langhendries J-L, Mullineux S-T, Stamatopoulou V, Mullier R, Wacheul L, Lafontaine DLJ. The complexity of human ribosome biogenesis revealed by systematic nucleolar screening of Pre-rRNA processing factors. Mol Cell 2013; 51:539 - 51; http://dx.doi.org/10.1016/j.molcel.2013.08.011; PMID: 23973377
- Neumüller RA, Gross T, Samsonova AA, Vinayagam A, Buckner M, Founk K, Hu Y, Sharifpoor S, Rosebrock AP, Andrews B, et al. Conserved regulators of nucleolar size revealed by global phenotypic analyses. Sci Signal 2013; 6:ra70; http://dx.doi.org/10.1126/scisignal.2004145; PMID: 23962978
- Woolford JL Jr., Baserga SJ. Ribosome biogenesis in the yeast Saccharomyces cerevisiae. Genetics 2013; 195:643 - 81; http://dx.doi.org/10.1534/genetics.113.153197; PMID: 24190922
- Wool IG. The structure and function of eukaryotic ribosomes. Annu Rev Biochem 1979; 48:719 - 54; http://dx.doi.org/10.1146/annurev.bi.48.070179.003443; PMID: 382996
- Zeevi D, Sharon E, Lotan-Pompan M, Lubling Y, Shipony Z, Raveh-Sadka T, Keren L, Levo M, Weinberger A, Segal E. Compensation for differences in gene copy number among yeast ribosomal proteins is encoded within their promoters. Genome Res 2011; 21:2114 - 28; http://dx.doi.org/10.1101/gr.119669.110; PMID: 22009988
- Koerte A, Chong T, Li X, Wahane K, Cai M. Suppression of the yeast mutation rft1-1 by human p53. J Biol Chem 1995; 270:22556 - 64; http://dx.doi.org/10.1074/jbc.270.38.22556; PMID: 7673248
- Di Ventura B, Funaya C, Antony C, Knop M, Serrano L. Reconstitution of Mdm2-dependent post-translational modifications of p53 in yeast. PLoS One 2008; 3:e1507; http://dx.doi.org/10.1371/journal.pone.0001507; PMID: 18231594
- Zhou J, Lancaster L, Trakhanov S, Noller HF. Crystal structure of release factor RF3 trapped in the GTP state on a rotated conformation of the ribosome. RNA 2012; 18:230 - 40; http://dx.doi.org/10.1261/rna.031187.111; PMID: 22187675
- Zhou J, Lancaster L, Donohue JP, Noller HF. Crystal structures of EF-G-ribosome complexes trapped in intermediate states of translocation. Science 2013; 340:1236086; http://dx.doi.org/10.1126/science.1236086; PMID: 23812722
- Bernstein KA, Baserga SJ. The small subunit processome is required for cell cycle progression at G1. Mol Biol Cell 2004; 15:5038 - 46; http://dx.doi.org/10.1091/mbc.E04-06-0515; PMID: 15356263
- Bernstein KA, Bleichert F, Bean JM, Cross FR, Baserga SJ. Ribosome biogenesis is sensed at the Start cell cycle checkpoint. Mol Biol Cell 2007; 18:953 - 64; http://dx.doi.org/10.1091/mbc.E06-06-0512; PMID: 17192414
- Strezoska Z, Pestov DG, Lau LF. Bop1 is a mouse WD40 repeat nucleolar protein involved in 28S and 5. 8S RRNA processing and 60S ribosome biogenesis. Mol Cell Biol 2000; 20:5516 - 28; http://dx.doi.org/10.1128/MCB.20.15.5516-5528.2000; PMID: 10891491
- Hölzel M, Rohrmoser M, Schlee M, Grimm T, Harasim T, Malamoussi A, Gruber-Eber A, Kremmer E, Hiddemann W, Bornkamm GW, et al. Mammalian WDR12 is a novel member of the Pes1-Bop1 complex and is required for ribosome biogenesis and cell proliferation. J Cell Biol 2005; 170:367 - 78; http://dx.doi.org/10.1083/jcb.200501141; PMID: 16043514
- Iwanami N, Higuchi T, Sasano Y, Fujiwara T, Hoa VQ, Okada M, Talukder SR, Kunimatsu S, Li J, Saito F, et al. WDR55 is a nucleolar modulator of ribosomal RNA synthesis, cell cycle progression, and teleost organ development. PLoS Genet 2008; 4:e1000171; http://dx.doi.org/10.1371/journal.pgen.1000171; PMID: 18769712
- Lapik YR, Fernandes CJ, Lau LF, Pestov DG. Physical and functional interaction between Pes1 and Bop1 in mammalian ribosome biogenesis. Mol Cell 2004; 15:17 - 29; http://dx.doi.org/10.1016/j.molcel.2004.05.020; PMID: 15225545
- Lerch-Gaggl AF, Sun K, Duncan SA. Light chain 1 of microtubule-associated protein 1B can negatively regulate the action of Pes1. J Biol Chem 2007; 282:11308 - 16; http://dx.doi.org/10.1074/jbc.M610977200; PMID: 17308336
- Oeffinger M, Tollervey D. Yeast Nop15p is an RNA-binding protein required for pre-rRNA processing and cytokinesis. EMBO J 2003; 22:6573 - 83; http://dx.doi.org/10.1093/emboj/cdg616; PMID: 14657029
- Thapa M, Bommakanti A, Shamsuzzaman M, Gregory B, Samsel L, Zengel JM, Lindahl L. Repressed synthesis of ribosomal proteins generates protein-specific cell cycle and morphological phenotypes. Mol Biol Cell 2013; 24:3620 - 33; http://dx.doi.org/10.1091/mbc.E13-02-0097; PMID: 24109599
- Fumagalli S, Ivanenkov VV, Teng T, Thomas G. Suprainduction of p53 by disruption of 40S and 60S ribosome biogenesis leads to the activation of a novel G2/M checkpoint. Genes Dev 2012; 26:1028 - 40; http://dx.doi.org/10.1101/gad.189951.112; PMID: 22588717
- Wang J, Leung JW, Gong Z, Feng L, Shi X, Chen J. PHF6 regulates cell cycle progression by suppressing ribosomal RNA synthesis. J Biol Chem 2013; 288:3174 - 83; http://dx.doi.org/10.1074/jbc.M112.414839; PMID: 23229552
- Tabb AL, Utsugi T, Wooten-Kee CR, Sasaki T, Edling SA, Gump W, Kikuchi Y, Ellis SR. Genes encoding ribosomal proteins Rps0A/B of Saccharomyces cerevisiae interact with TOM1 mutants defective in ribosome synthesis. Genetics 2001; 157:1107 - 16; PMID: 11238398
- Miles TD, Jakovljevic J, Horsey EW, Harnpicharnchai P, Tang L, Woolford JL Jr.. Ytm1, Nop7, and Erb1 form a complex necessary for maturation of yeast 66S preribosomes. Mol Cell Biol 2005; 25:10419 - 32; http://dx.doi.org/10.1128/MCB.25.23.10419-10432.2005; PMID: 16287855
- Kinoshita Y, Jarell AD, Flaman JM, Foltz G, Schuster J, Sopher BL, Irvin DK, Kanning K, Kornblum HI, Nelson PS, et al. Pescadillo, a novel cell cycle regulatory protein abnormally expressed in malignant cells. J Biol Chem 2001; 276:6656 - 65; http://dx.doi.org/10.1074/jbc.M008536200; PMID: 11071894
- Jorgensen P, Tyers M. How cells coordinate growth and division. Curr Biol 2004; 14:R1014 - 27; http://dx.doi.org/10.1016/j.cub.2004.11.027; PMID: 15589139
- Jorgensen P, Nishikawa JL, Breitkreutz BJ, Tyers M. Systematic identification of pathways that couple cell growth and division in yeast. Science 2002; 297:395 - 400; http://dx.doi.org/10.1126/science.1070850; PMID: 12089449
- Costanzo M, Nishikawa JL, Tang X, Millman JS, Schub O, Breitkreuz K, Dewar D, Rupes I, Andrews B, Tyers M. CDK activity antagonizes Whi5, an inhibitor of G1/S transcription in yeast. Cell 2004; 117:899 - 913; http://dx.doi.org/10.1016/j.cell.2004.05.024; PMID: 15210111
- Skotheim JM, Di Talia S, Siggia ED, Cross FR. Positive feedback of G1 cyclins ensures coherent cell cycle entry. Nature 2008; 454:291 - 6; http://dx.doi.org/10.1038/nature07118; PMID: 18633409
- Gómez-Herreros F, de Miguel-Jiménez L, Morillo-Huesca M, Delgado-Ramos L, Muñoz-Centeno MC, Chávez S. TFIIS is required for the balanced expression of the genes encoding ribosomal components under transcriptional stress. Nucleic Acids Res 2012; 40:6508 - 19; http://dx.doi.org/10.1093/nar/gks340; PMID: 22544605
- Gómez-Herreros F, Rodríguez-Galán O, Morillo-Huesca M, Maya D, Arista-Romero M, de la Cruz J, Chávez S, Muñoz-Centeno MC. Balanced production of ribosome components is required for proper G1/S transition in Saccharomyces cerevisiae. J Biol Chem 2013; 288:31689 - 700; http://dx.doi.org/10.1074/jbc.M113.500488; PMID: 24043628
- Shaw RJ, Reines D. Saccharomyces cerevisiae transcription elongation mutants are defective in PUR5 induction in response to nucleotide depletion. Mol Cell Biol 2000; 20:7427 - 37; http://dx.doi.org/10.1128/MCB.20.20.7427-7437.2000; PMID: 11003640
- Shaw RJ, Wilson JL, Smith KT, Reines D. Regulation of an IMP dehydrogenase gene and its overexpression in drug-sensitive transcription elongation mutants of yeast. J Biol Chem 2001; 276:32905 - 16; http://dx.doi.org/10.1074/jbc.M105075200; PMID: 11441018
- Sun XX, Dai MS, Lu H. Mycophenolic acid activation of p53 requires ribosomal proteins L5 and L11. J Biol Chem 2008; 283:12387 - 92; http://dx.doi.org/10.1074/jbc.M801387200; PMID: 18305114
- Donati G, Brighenti E, Vici M, Mazzini G, Treré D, Montanaro L, Derenzini M. Selective inhibition of rRNA transcription downregulates E2F-1: a new p53-independent mechanism linking cell growth to cell proliferation. J Cell Sci 2011; 124:3017 - 28; http://dx.doi.org/10.1242/jcs.086074; PMID: 21878508
- Iadevaia V, Caldarola S, Biondini L, Gismondi A, Karlsson S, Dianzani I, Loreni F. PIM1 kinase is destabilized by ribosomal stress causing inhibition of cell cycle progression. Oncogene 2010; 29:5490 - 9; http://dx.doi.org/10.1038/onc.2010.279; PMID: 20639905
- Li J, Yu L, Zhang H, Wu J, Yuan J, Li X, Li M. Down-regulation of pescadillo inhibits proliferation and tumorigenicity of breast cancer cells. Cancer Sci 2009; 100:2255 - 60; http://dx.doi.org/10.1111/j.1349-7006.2009.01325.x; PMID: 19764998
- Zhang H, Shertok S, Miller K, Taylor L, Martin-Deleon PA. Sperm dysfunction in the Rb(6.16)- and Rb(6.15)-bearing mice revisited: involvement of Hyalp1 and Hyal5. Mol Reprod Dev 2005; 72:404 - 10; http://dx.doi.org/10.1002/mrd.20360; PMID: 16078272
- Zhang Z, Wang H, Li M, Agrawal S, Chen X, Zhang R. MDM2 is a negative regulator of p21WAF1/CIP1, independent of p53. J Biol Chem 2004; 279:16000 - 6; http://dx.doi.org/10.1074/jbc.M312264200; PMID: 14761977
- Dai M-S, Arnold H, Sun X-X, Sears R, Lu H. Inhibition of c-Myc activity by ribosomal protein L11. EMBO J 2007; 26:3332 - 45; http://dx.doi.org/10.1038/sj.emboj.7601776; PMID: 17599065
- Lin WC, Lin FT, Nevins JR. Selective induction of E2F1 in response to DNA damage, mediated by ATM-dependent phosphorylation. Genes Dev 2001; 15:1833 - 44; PMID: 11459832
- Stevens C, Smith L, La Thangue NB. Chk2 activates E2F-1 in response to DNA damage. Nat Cell Biol 2003; 5:401 - 9; http://dx.doi.org/10.1038/ncb974; PMID: 12717439
- Wang Z, Bhattacharya N, Weaver M, Petersen K, Meyer M, Gapter L, Magnuson NS. Pim-1: a serine/threonine kinase with a role in cell survival, proliferation, differentiation and tumorigenesis. J Vet Sci 2001; 2:167 - 79; PMID: 12441685
- Bachmann M, Möröy T. The serine/threonine kinase Pim-1. Int J Biochem Cell Biol 2005; 37:726 - 30; http://dx.doi.org/10.1016/j.biocel.2004.11.005; PMID: 15694833
- Chiocchetti A, Gibello L, Carando A, Aspesi A, Secco P, Garelli E, Loreni F, Angelini M, Biava A, Dahl N, et al. Interactions between RPS19, mutated in Diamond-Blackfan anemia, and the PIM-1 oncoprotein. Haematologica 2005; 90:1453 - 62; PMID: 16266891
- Larrea MD, Wander SA, Slingerland JM. p27 as Jekyll and Hyde: regulation of cell cycle and cell motility. Cell Cycle 2009; 8:3455 - 61; http://dx.doi.org/10.4161/cc.8.21.9789; PMID: 19829074
- Grimm T, Hölzel M, Rohrmoser M, Harasim T, Malamoussi A, Gruber-Eber A, Kremmer E, Eick D. Dominant-negative Pes1 mutants inhibit ribosomal RNA processing and cell proliferation via incorporation into the PeBoW-complex. Nucleic Acids Res 2006; 34:3030 - 43; http://dx.doi.org/10.1093/nar/gkl378; PMID: 16738141
- Soussi T, Dehouche K, Béroud C. p53 website and analysis of p53 gene mutations in human cancer: forging a link between epidemiology and carcinogenesis. Hum Mutat 2000; 15:105 - 13; http://dx.doi.org/10.1002/(SICI)1098-1004(200001)15:1<105::AID-HUMU19>3.0.CO;2-G; PMID: 10612830
- Avet-Loiseau H, Attal M, Moreau P, Charbonnel C, Garban F, Hulin C, Leyvraz S, Michallet M, Yakoub-Agha I, Garderet L, et al. Genetic abnormalities and survival in multiple myeloma: the experience of the Intergroupe Francophone du Myélome. Blood 2007; 109:3489 - 95; http://dx.doi.org/10.1182/blood-2006-08-040410; PMID: 17209057
- Jakubowiak AJ, Siegel DS, Martin T, Wang M, Vij R, Lonial S, Trudel S, Kukreti V, Bahlis N, Alsina M, et al. Treatment outcomes in patients with relapsed and refractory multiple myeloma and high-risk cytogenetics receiving single-agent carfilzomib in the PX-171-003-A1 study. Leukemia 2013; 27:2351 - 6; http://dx.doi.org/10.1038/leu.2013.152; PMID: 23670297
- Malumbres M, Harlow E, Hunt T, Hunter T, Lahti JM, Manning G, Morgan DO, Tsai LH, Wolgemuth DJ. Cyclin-dependent kinases: a family portrait. Nat Cell Biol 2009; 11:1275 - 6; http://dx.doi.org/10.1038/ncb1109-1275; PMID: 19884882
- Cirstea IC, Gremer L, Dvorsky R, Zhang SC, Piekorz RP, Zenker M, Ahmadian MR. Diverging gain-of-function mechanisms of two novel KRAS mutations associated with Noonan and cardio-facio-cutaneous syndromes. Hum Mol Genet 2013; 22:262 - 70; http://dx.doi.org/10.1093/hmg/dds426; PMID: 23059812
- Mizushima N, Komatsu M. Autophagy: renovation of cells and tissues. Cell 2011; 147:728 - 41; http://dx.doi.org/10.1016/j.cell.2011.10.026; PMID: 22078875
- Boglev Y, Badrock AP, Trotter AJ, Du Q, Richardson EJ, Parslow AC, Markmiller SJ, Hall NE, de Jong-Curtain TA, Ng AY, et al. Autophagy induction is a Tor- and Tp53-independent cell survival response in a zebrafish model of disrupted ribosome biogenesis. PLoS Genet 2013; 9:e1003279; http://dx.doi.org/10.1371/journal.pgen.1003279; PMID: 23408911
- Baserga R. The path less traveled by. Endocrinology 1997; 138:2217 - 8; http://dx.doi.org/10.1210/endo.138.6.5252; PMID: 9165003
- Dosil M, Bustelo XR. Functional characterization of Pwp2, a WD family protein essential for the assembly of the 90 S pre-ribosomal particle. J Biol Chem 2004; 279:37385 - 97; http://dx.doi.org/10.1074/jbc.M404909200; PMID: 15231838
- Poortinga G, Hannan KM, Snelling H, Walkley CR, Jenkins A, Sharkey K, Wall M, Brandenburger Y, Palatsides M, Pearson RB, et al. MAD1 and c-MYC regulate UBF and rDNA transcription during granulocyte differentiation. EMBO J 2004; 23:3325 - 35; http://dx.doi.org/10.1038/sj.emboj.7600335; PMID: 15282543
- van Riggelen J, Yetil A, Felsher DW. MYC as a regulator of ribosome biogenesis and protein synthesis. Nat Rev Cancer 2010; 10:301 - 9; http://dx.doi.org/10.1038/nrc2819; PMID: 20332779
- Russo A, Esposito D, Catillo M, Pietropaolo C, Crescenzi E, Russo G. Human rpL3 induces G₁/S arrest or apoptosis by modulating p21 (waf1/cip1) levels in a p53-independent manner. Cell Cycle 2013; 12:76 - 87; http://dx.doi.org/10.4161/cc.22963; PMID: 23255119
- Russo A, Catillo M, Esposito D, Briata P, Pietropaolo C, Russo G. Autoregulatory circuit of human rpL3 expression requires hnRNP H1, NPM and KHSRP. Nucleic Acids Res 2011; 39:7576 - 85; http://dx.doi.org/10.1093/nar/gkr461; PMID: 21705779
- Koutsodontis G, Moustakas A, Kardassis D. The role of Sp1 family members, the proximal GC-rich motifs, and the upstream enhancer region in the regulation of the human cell cycle inhibitor p21WAF-1/Cip1 gene promoter. Biochemistry 2002; 41:12771 - 84; http://dx.doi.org/10.1021/bi026141q; PMID: 12379120
- Sugimoto M, Kuo ML, Roussel MF, Sherr CJ. Nucleolar Arf tumor suppressor inhibits ribosomal RNA processing. Mol Cell 2003; 11:415 - 24; http://dx.doi.org/10.1016/S1097-2765(03)00057-1; PMID: 12620229
- Saporita AJ, Chang HC, Winkeler CL, Apicelli AJ, Kladney RD, Wang J, Townsend RR, Michel LS, Weber JD. RNA helicase DDX5 is a p53-independent target of ARF that participates in ribosome biogenesis. Cancer Res 2011; 71:6708 - 17; http://dx.doi.org/10.1158/0008-5472.CAN-11-1472; PMID: 21937682
- Stiewe T. The p53 family in differentiation and tumorigenesis. Nat Rev Cancer 2007; 7:165 - 8; http://dx.doi.org/10.1038/nrc2072; PMID: 17332760
- Marcel V, Dichtel-Danjoy M-L, Sagne C, Hafsi H, Ma D, Ortiz-Cuaran S, Olivier M, Hall J, Mollereau B, Hainaut P, et al. Biological functions of p53 isoforms through evolution: lessons from animal and cellular models. Cell Death Differ 2011; 18:1815 - 24; http://dx.doi.org/10.1038/cdd.2011.120; PMID: 21941372
- Brodsky MH, Weinert BT, Tsang G, Rong YS, McGinnis NM, Golic KG, Rio DC, Rubin GM. Drosophila melanogaster MNK/Chk2 and p53 regulate multiple DNA repair and apoptotic pathways following DNA damage. Mol Cell Biol 2004; 24:1219 - 31; http://dx.doi.org/10.1128/MCB.24.3.1219-1231.2004; PMID: 14729967
- Yamada Y, Davis KD, Coffman CR. Programmed cell death of primordial germ cells in Drosophila is regulated by p53 and the Outsiders monocarboxylate transporter. Development 2008; 135:207 - 16; http://dx.doi.org/10.1242/dev.010389; PMID: 18057102
- Sekelsky JJ, Brodsky MH, Burtis KC. DNA repair in Drosophila: insights from the Drosophila genome sequence. J Cell Biol 2000; 150:F31 - 6; http://dx.doi.org/10.1083/jcb.150.2.F31; PMID: 10908583
- Ollmann M, Young LM, Di Como CJ, Karim F, Belvin M, Robertson S, Whittaker K, Demsky M, Fisher WW, Buchman A, et al. Drosophila p53 is a structural and functional homolog of the tumor suppressor p53. Cell 2000; 101:91 - 101; http://dx.doi.org/10.1016/S0092-8674(00)80626-1; PMID: 10778859
- Akdemir F, Christich A, Sogame N, Chapo J, Abrams JM. p53 directs focused genomic responses in Drosophila.. Oncogene 2007; 26:5184 - 93; http://dx.doi.org/10.1038/sj.onc.1210328; PMID: 17310982
- Brodsky MH, Nordstrom W, Tsang G, Kwan E, Rubin GM, Abrams JM. Drosophila p53 binds a damage response element at the reaper locus. Cell 2000; 101:103 - 13; http://dx.doi.org/10.1016/S0092-8674(00)80627-3; PMID: 10778860
- Dichtel-Danjoy ML, Ma D, Dourlen P, Chatelain G, Napoletano F, Robin M, Corbet M, Levet C, Hafsi H, Hainaut P, et al. Drosophila p53 isoforms differentially regulate apoptosis and apoptosis-induced proliferation. Cell Death Differ 2013; 20:108 - 16; http://dx.doi.org/10.1038/cdd.2012.100; PMID: 22898807
- Fan Y, Bergmann A. The cleaved-Caspase-3 antibody is a marker of Caspase-9-like DRONC activity in Drosophila. Cell Death Differ 2010; 17:534 - 9; http://dx.doi.org/10.1038/cdd.2009.185; PMID: 19960024
- Grewal SS, Evans JR, Edgar BA. Drosophila TIF-IA is required for ribosome synthesis and cell growth and is regulated by the TOR pathway. J Cell Biol 2007; 179:1105 - 13; http://dx.doi.org/10.1083/jcb.200709044; PMID: 18086911
- Yuan X, Zhou Y, Casanova E, Chai M, Kiss E, Gröne H-J, Schütz G, Grummt I. Genetic inactivation of the transcription factor TIF-IA leads to nucleolar disruption, cell cycle arrest, and p53-mediated apoptosis. Mol Cell 2005; 19:77 - 87; http://dx.doi.org/10.1016/j.molcel.2005.05.023; PMID: 15989966
- D’Amours D, Jackson SP. The Mre11 complex: at the crossroads of dna repair and checkpoint signalling. Nat Rev Mol Cell Biol 2002; 3:317 - 27; http://dx.doi.org/10.1038/nrm805; PMID: 11988766
- Takada S, Kelkar A, Theurkauf WE. Drosophila checkpoint kinase 2 couples centrosome function and spindle assembly to genomic integrity. Cell 2003; 113:87 - 99; http://dx.doi.org/10.1016/S0092-8674(03)00202-2; PMID: 12679037
- McNamee LM, Brodsky MH. p53-independent apoptosis limits DNA damage-induced aneuploidy. Genetics 2009; 182:423 - 35; http://dx.doi.org/10.1534/genetics.109.102327; PMID: 19364807
- Moreno E, Basler K, Morata G. Cells compete for decapentaplegic survival factor to prevent apoptosis in Drosophila wing development. Nature 2002; 416:755 - 9; http://dx.doi.org/10.1038/416755a; PMID: 11961558
- Tyler DM, Li W, Zhuo N, Pellock B, Baker NE. Genes affecting cell competition in Drosophila.. Genetics 2007; 175:643 - 57; http://dx.doi.org/10.1534/genetics.106.061929; PMID: 17110495
- Martín FA, Pérez-Garijo A, Moreno E, Morata G. The brinker gradient controls wing growth in Drosophila.. Development 2004; 131:4921 - 30; http://dx.doi.org/10.1242/dev.01385; PMID: 15371310
- Fogarty P, Campbell SD, Abu-Shumays R, Phalle BS, Yu KR, Uy GL, Goldberg ML, Sullivan W. The Drosophila grapes gene is related to checkpoint gene chk1/rad27 and is required for late syncytial division fidelity. Curr Biol 1997; 7:418 - 26; http://dx.doi.org/10.1016/S0960-9822(06)00189-8; PMID: 9197245
- Titen SW, Golic KG. Telomere loss provokes multiple pathways to apoptosis and produces genomic instability in Drosophila melanogaster.. Genetics 2008; 180:1821 - 32; http://dx.doi.org/10.1534/genetics.108.093625; PMID: 18845846