Abstract
Tissue engineering aims to develop functionalized tissues for organ replacement or restoration. Biodegradable scaffolds have been used in tissue engineering to support cell growth and maintain mechanical and biological properties of tissue constructs. Ideally cells on these scaffolds adhere, proliferate, and deposit matrix at a rate that is consistent with scaffold degradation. However, the cellular rearrangement within these scaffolds often does not recapitulate the architecture of the native tissues. Directed assembly of tissue-like structures is an attractive alternative to scaffold-based approach for tissue engineering which potentially can build tissue constructs with biomimetic architecture and function. In directed assembly, shape-controlled microstructures are fabricated in which organized structures of different cell types can be used as tissue building blocks. To fabricate tissue building blocks, hydrogels are commonly used as biomaterials for cell encapsulation to mimic the matrix in vivo. The hydrogel-based tissue building blocks can be arranged in pre-defined architectures by various directed tissue assembly techniques. In this paper, recent advances in directed assembly-based tissue engineering are summarized as an emerging alternative to meet challenges associated with scaffold-based tissue engineering and future directions are addressed.
Introduction
Regenerative medicine is an emerging branch of medicine that aims to cure patients suffering from severe tissue and organ failure. Regenerative medicine has the potential to resolve the problems associated with transplantation such as the shortage of donor organs and the immune rejection. Despite significant progress in this area, however, three key challenges remain in generating artificial tissues for clinical use.Citation1 These include: (1) the inability to obtain an adequate number of organ-specific cells, (2) the inability to generate functional tissue constructs and (3) the lack of vasculature in engineered tissues.
With the recent advances in stem cell biology, it is becoming more realistic to obtain an adequate number of autologous or immunologically compatible cells. However, only a small fraction of injected cells are integrated into the host organs. To address this issue, engineering tissues using these cells before transplantation may be an important strategy. There have been many examples that utilize biodegradable porous scaffolds to engineer tissues, a few of which have already been used in the clinic.Citation2 While this approach has been widely used and has shown to be useful,Citation3 it requires cells to rearrange themselves for generating tissue-like complexity. This re-organization process, which is often essential for recreating the desired tissue function, would benefit from engineering systems that aim to aid it in recreating biomimetic tissue complexity. An emerging approach to generate tissues with tissue-like microarchitectures is based on the directed assembly of functional tissue units. This process aims to assemble cell aggregates or cell-laden structures for creating biomimetic hierarchical structures.Citation4
In this paper, we discuss the latest progress in directing the assembly of cell-containing structures to generate engineered tissues. We will first discuss organ structure and scaffold-based tissue engineering, followed by fabrication approaches of cell-laden microgels as building blocks. We will then discuss different methods for directed assembly of cell-laden hydrogels. The paper will be concluded with the future perspectives to directed assembly technology.
Organ structure and tissue regeneration.
Billions of cells are arranged in a highly organized manner to make functional tissues.Citation5–Citation9 During tissue development, formation of organ-specific tissue microarchitecture is influenced by spatio-temporal microenvironmental factors such as physical forces and chemical cues. Reciprocally, the tissue architecture guides the tissue development and function. The role of tissue microarchitecture is highly related to its resulting function and thus any attempts at recreating engineered tissues must aim to recreate biomimetic tissue structures.
Most tissues generate an organized complex architecture by assembly of repeating functional tissue units over several scales. Examples of functional tissue units are islets in the pancreas, nephrons in the kidney and lobules in the liver.Citation10 These functional tissue units are highly vascularized and contain many different cell types that are positioned relative to each other in a highly organized matter. Given this complexity, the architecture of the initial cell-laden construct is important for reacting this tissue structure.
Scaffold-based tissue engineering largely relies on migration, proliferation and differentiation of cells in a biodegradable porous scaffold. Although cells have a great capacity to rearrange and reorganize upon seeding on tissue engineering scaffolds, they may not fully reorganize themselves into tissue-like functional units. Directed assembly-based tissue engineering could be a potentially powerful tool to overcome these challenges and to recreate tissue complexity. In this approach, microfabrication techniques are used to control and organize the relative position of individual cells in length scales of around 1–10 µm while these fabricated constructs are assembled as building blocks that are in the order of 10–1,000 µm to obtain biomimetic repeating units. This assembly is further coupled with approaches such as micromolding of hydrogel microchannels to fabricate vascular structures in order to engineer milli- and centimeter-scale tissue constructs. Thus the directed assembly-based tissue engineering has significant potential to generate tissue constructs with controlled structures that range from microscale to organ level.
Scaffold-based tissue engineering.
Biodegradable scaffolds have been commonly used for engineering tissue constructs. A scaffold is a structural support that facilitates the formation of tissues from seeded cells.Citation11–Citation16 The scaffold ideally degrades at a rate similar to the rate of cell growth and matrix deposition such that it will completely be replaced by engineered tissue. Various scaffold features, such as structure, physical cues and mechanical properties can be modulated to regulate the tissue development and remodeling.Citation11–Citation17
Although scaffold-based tissue engineering is well established and proven to be useful, it has faced some challenges to build tissue constructs with biomimetic architecture and function. Cell-ECM interactions and cell-cell signals from specialized cells within close proximity play significant role to shape the microenvironment, to preserve the cell phenotype and to direct the cell differentiation. Therefore, the arrangement of cells as well as the structure and composition of microenvironment are important in maintaining the tissue structure and function. However, the cellular rearrangement within scaffolds often does not resemble the biomimetic structure of the native tissue. In addition, cell seeding of the scaffold as well as subsequent vascularization are major hurdles. These become even more complicated considering that organs contain multiple cell types in close proximity which are organized within microscale resolution in multiple scales.
Directed assembly-based tissue engineering.
Directed assembly-based tissue engineering aims to address the uncontrolled arrangement of cells and remodeling of microenvironment structure. This approach also has the potential to address the tissue vascularization. Directed assembly employs biomimetic techniques by fabrication of small microstructures to arrange different cell types in close proximity of each other. These building blocks can be made from cell aggregates or cell containing hydrogel blocks.Citation18–Citation23 Hydrogel encapsulated cells can mimic functional tissue units so that the tissue function may be potentially preserved by the arrangement of these building blocks in a desired geometry with specific shape and size. Directed tissue assembly is a promising approach which provides enhanced level of control to organize tissue building blocks into desired patterns which may enhance the functionality of the final tissue construct. There are different techniques for making microengineered cell-laden hydrogels as tissue building blocks, which are discussed in the following section. It is important to note that cell aggregates are also a suitable building block of tissue-like constructs which can be directly assembled to form a tissue construct. Although these techniques are not discussed here, the readers are directed to a number of recent works in generating cell aggregates with controlled shape and size.Citation19,Citation20
Fabrication Techniques for Engineering Microscale Hydrogels
Cell-cell and cell-ECM (extracellular matrix) interactions shape the biomimetic structure of microenvironment which can direct the tissue structure and function. To provide a three-dimensional (3D) environment for cells, biocompatible hydrogels have been extensively used for engineering tissue constructs.Citation21,Citation22 Hydrogels are 3D crosslinked networks of hydrophilic polymers with high water content. In general, hydrogels are highly permeable to oxygen, nutrients and other water-soluble metabolitesCitation23 which make them ideally suited for cell encapsulation since they mimic the natural ECM.Citation24
Hydrogels can be derived from either natural sources such as fibrin,Citation25 hyaluronic acid (HA),Citation26 and agarose;Citation27 or be synthetically formed such as poly(ethylene glycol) (PEG).Citation28,Citation29 Both natural and synthetic hydrogels have been used for cell encapsulation and have been engineered to create structures with features between 0.1–10 mm.Citation30 A number of different techniques have been developed for controlling the shape of hydrogels with microscale resolution (i.e., microgels). These approaches utilize technologies such as emulsification, photolithography, microfluidics, micromolding, modular assembly and printing. Here we describe each of these techniques in detail.
Emulsification is a widely used technique for generating microgels. In this process a multi-phase mixture is stirred to generate small aqueous droplets of the hydrogel precursors within an organic phase.Citation30 Aqueous droplets are subsequently gelled by crosslinking via various mechanisms to generate spherical microgels (). This approach is highly scalable and can be used to easily generate microgels. However a key limitation of emulsification is that it is often limited to spherical microgels while a relatively wide distribution of microgel sizes is usually generated.
Photolithography is a technique that has been widely used in the microelectronics industry. In this process a thin film of a polymer is exposed to light through a mask. As the light reaches the photosensitive polymer through the transparent regions of a mask, polymer will be crosslinked through photopolymerizationCitation30 (). Synthetic and natural photocrosslinkable prepolymers have been developed to make hydrogels while a number of studies have demonstrated that photocrosslinkable microgels made from various types of synthetic polymers such as PEGCitation31,Citation32 can be used for cell encapsulation.Citation33
Microfluidics systems can also be used for generating microengineered hydrogels. Microfluidic devices which operate based on the movement of fluids through microscale channels provide a unique platform for generating hydrogels with controlled features. A common method of using microfluidic systems to generate microparticles is through the use of multiphase system. In this approach the surface tension and viscosity are used to create homogeneous particles that can be cross-linked to form microscale hydrogelsCitation31 (). Interestingly, microfluidic techniques and photolithography have been merged to generate other methods of fabricating microscale hydrogels by using the channels to deliver the hydrogel precursors to a region where they gel by light exposure. This approach can be used to generate microgels with capability of controlling their shapes and sizes in a continuous manner.Citation34
Micromolding is another technique for generating hydrogels with controlled featuresCitation35 (). Micromolding has become particularly appealing due to the development of methods such as soft lithography that has enabled easy fabrication of poly(dimethyl siloxane) (PDMS) molds from prefabricated silicon wafers.Citation30 To generate micromolded hydrogels, precursor polymers are initially molded and subsequently gelled to generate structures with different shapes and sizes (). Micromolding has also been used to generate microengineered hydrogels from a variety of materials including hyaluronic acid (HA),Citation36,Citation37 chitosanCitation38 and PEG.Citation39 In addition micromolding techniques have been recently adapted to micromold hydrogels such as alginate and fibrin that require the addition of crosslinkers such as divalent cations. These materials, which encompass a large class of hydrogels, were molded by using hydrogel micromolds that were capable of simultaneously controlling the shape of the hydrogel precursors and the delivery of crosslinking agents.Citation40
Printing technology can also be used to encapsulate cells in hydrogels.Citation41 In this process, standard or modified printers are used where paper and ink are replaced with substrate (usually polymer made) and cell-laden medium respectively.Citation42 In all of the hydrogel-based building block fabrication techniques that have been discussed so far in this section, the building blocks are first fabricated and then they will be organized in specific geometry using directed assembly. In contrast, in printing method, both steps of fabrication and assembly of hydrogels are merged. We discuss the tissue printing technique in detail in the next section. In we summarize some of the applications as well as pros and cons of these different methods.
Directed Assembly of Cell-Laden Hydrogels for Engineering Tissue Constructs
Directed assembly can be used to arrange tissue building blocks in a biomimetic manner to aid in subsequent tissue formation through dynamic tissue remodeling. The microengineered building blocks are expected to maintain both biomimetic architecture and function of the encapsulated cells which can be achieved by controlling cell-microenvironment interactions through substrate mechanics, biomaterial chemistry, applied physical forces and the degradation of surrounding materials.Citation30
Different techniques for fabricating hydrogel-based tissue building blocks were discussed in the previous section. In this section, we discuss different directed assembly techniques to assemble hydrogel-based building blocks into a tissue construct with controlled geometry and functionality.
Directed assembly at a two-phase interface.
Our group has developed a number of approaches for using directed assembly to build tissue-like constructs with well-defined macroscale architectures from cell-laden microgels. For example, the directed assembly of cell-laden microgels can be achieved by harnessing the surface tension characteristics of hydrophilic hydrogels in a two-phase oil-aqueous solution reactorCitation43 (). First, cell-laden hydrogels were created in various sizes and shapes through photolithography. These micro-tissue modules were subsequently transferred to a hydrophobic mineral oil and mechanical agitation was applied to induce the aggregation of the hydrophilic microgels to form tissues of varying dimensions. The microgel assembly could then be stabilized through a secondary photopolymerization which allows the assembled construct to be cultured in the aqueous medium. The number of hydrogels per tissue was increased proportionally with the module aspect ratio which demonstrates the ability to partially regulate the ultimate size and shape of the resulting assembled tissue. Microgel assemblies with more intricate structures were fabricated by using the lock-and-key shaped microgels indicating the potential versatility of this technique. This technique presents the first attempt to address the challenges of creating higher order structures by directed assembly for tissue modules that are fragile or otherwise difficult to manipulate. Potential advantages of this approach include the ability to direct the structure of the constructed tissue based on the module geometry without the need for complicated assembly and manipulation. However, random or uncontrolled structures still may form such that fabricating tissue constructs with biologically relevant length scale using the current setup is challenging. The development of well-controllable and scaled-up bioreactor may solve some of these challenges. A recent work from our group has partially addressed the scaling-up issue by creating centimeter-scale cell-laden microgel assemblies at liquid-air interface of a hydrophobic solution.Citation44 The directed assembly was initiated by randomly positioning cell-laden microgels of specific shapes on the surface of a high density, hydrophobic solution (i.e., perfluorodecalin (PFDC)) (). The surface tension at the liquid-air interface induced the aggregation of the hydrophilic microgels on the surface of the hydrophobic medium. The resultant assembled hydrogel sheets consisting of tightly packed microgels were subsequently crosslinked into macroscale tissue-like structures. In addition, complex multigel building blocks were created by using a hierarchical approach and were assembled with precise spatial control over the cell distribution into tissues (). These experiments show that air-liquid interface forces can be used to spatially control the self-assembly of co-cultured tissue-like structures.
Directed assembly on hydrophilic templates.
Despite the success in directed assembly of hydrophilic microgels at a two-phase interface, hydrophobic medium is not suitable for cell/tissue culture as it requires an additional removal step. Therefore, the development of alternative approaches that can be used to assemble microgels in aqueous medium is of great benefit for building tissue constructs with clinical significance. Du et al. achieved directed assembly of cell-laden microgels within aqueous droplets on surfaces patterned with hydrophobic and hydrophilic regions.Citation45 Driven by surface-tension, the hydrophilic microgels assembled within patterned aqueous droplets, which are confined inside the hydrophilic patterns and stabilized by a secondary crosslinking (). Using this process microgel assemblies with well-regulated architecture were formed on the glass slide by controlling the size and shape of the surface patterns ( and C). The stabilized assembled microgels could be readily harvested from the surface as multi-cellular tissue constructs (). This “bottom-up” approach enables rapid and scalable fabrication of cell-laden microgel assemblies with predefined geometrical and biological features, which can potentially be used for microscale tissue engineering applications. Restricted in the flattened surface patterns, the final structure of the microgel assembly produced by this approach is limited to two-dimensional (2D) structures, which is applicable to mimic simple tissues (i.e., skin) while it lacks the complexity of 3D tissue structures.
Recent progress has been made to expand the directed assembly approach to generate 3D structures with higher complexity. Fernandez et al. developed a directed assembly process which occurs on a hydrophilic surface. The PDMS surface was used as a hydrophilic template which attracts and confines the microgel subunits.Citation46 As shown in , microgels made with specific shapes were first mixed in a pre-polymer solution and deposited on an oxygen-plasma treated PDMS surface. Due to hydrophilic affinity, the liquid wets the surface and drives the microgel subunits to cover the PDMS surface. Upon removal of the excess pre-polymer solution, microgels assemble into a closely packed sheet on the surface of the template to minimize the system energy which was driven by the capillary forces exerted by the remaining pre-polymer. Secondary photocrosslinking was used to stabilize the resulting assembly, which could be separated from its PDMS template to form a stand-alone microgel assembly. Due to the ease of molding PDMS to various 2D and 3D structures, this approach can be used to construct microgel assembly with a range of shapes and complexities such as tube, sphere and casqued (). Further applications of this approach have been also shown to build larger scaffolds in a layer-by-layer manner where double-layer tube with 5 mm in diameter was fabricated (). The entire directed assembly procedure is proven to be cell-friendly by assembling hepatic cells (HepG2) encapsulated PEG microgels into a tube-like constructs ().
Directed assembly within physical template.
As an alternative to hydrophilic template, physical template is a powerful tool to induce the directed assembly of cell-laden microgels into defined geometries and shapes within physical barriers. Bruzewicz et al. directed the assembly of cell-laden microgels by loosely packing the modules into microfluidic chambers (channels) as a physical template.Citation47 The microgel assemblies exhibited structures defined by the physical template (). The order in the packed assemblies increased as the width of the microchannels approached the width of the modules. The physical chamber allowed perfusion of the tissue assembly through the interconnected pores. One benefit of this assembly approach is the ability to form an assembly that contains multiple types of cells. The ability to precisely organize different types of cell-laden microgels into a 3D array enables fabrication of 3D tissue constructs with hierarchical structures. Artificial tissues with different structures containing multiple cell types (i.e., HepG2 cells and 3T3 fibroblasts) can be spatially organized in the small microfluidic channels (). Recovery and analysis of modules after 24 h under constant flow of medium showed that over 99% of encapsulated cells survived this interval in the microfluidic chamber. The physical template-based assembly enables the control of the final geometry of the microgel assembly but it is hard to control the order of microgel modules within the assembly when the template is much larger than the cell-laden modules.
Physical manipulation of individual microgels into ordered patterns was achieved by using a micromanipulator.Citation37 An example is an alternating checkerboard pattern (), assembled with fluorescently red- and green-stained cells in separate sets of microgels. This approach is relatively inefficient and non-scalable for fabrication of large-scale tissue due to difficulties encountered in manipulating individual microgels.
McGuigan et al. assembled the modular components, consisting of submillimeter-sized collagen gel rods seeded with endothelial cells (ECs) in a chamber to build micro-vascularized tissueCitation48 (). The EC-covered collagen modular units (with HepG2 encapsulated) were assembled into a larger tube and perfused with medium or whole blood. The resultant tissue assembly consists of interconnected cellular modules, which are perfusable and non-coagulative due to endothelialization ( and H). Random assembly of microgel modules is rapid and simple, but lacks the control over the final structure of the hydrogel aggregate.
Microfluidic-directed assembly is a promising approach for parallel fabrication of devices made up of many small components with high degree of accuracy. Chung et al. developed “railed microfluidics,” a fully deterministic way to guide and assemble microstructures inside fluidic channels.Citation49 They fabricated grooves (rails) on the top surface of the PDMS channels as guidance for the movement of microstructures which were fabricated with complementary polymeric microstructures to fit with the grooves ().
By using the rails as a guiding mechanism, complex one- and two-dimensional microsystems, each composed of more than 50 hydrogel-based microstructures (each sized smaller than 50 µm), were fluidically self-assembled (). Furthermore, they achieved heterogeneous directed-assembly of two types of living cell-laden hydrogel building blocks by taking advantages of rail-guided self-assembly and cross-solution movement ( and D). Owing to its simplicity and flexibility, railed microfluidics will not only impact current self-assembly but also encourage innovation in a wide range of application areas in the future.
An attractive technique to modularly assemble the large porous cell-laden hydrogels is introduced by Liu et al.Citation50 Poly(ethylene glycol) diacrylate (PEGDA) made cell-laden microgels were first fabricated using photolithography, and then physically bonded in the presence of a cross-linker carrying multiple thiol groups through a Michael-type addition reaction. The tissue construct was assembled through the reaction between the thiol groups on the crosslinker and the acrylate groups on the surface of the building block microgels. It was reported that by using this approach, cells were uniformly be distributed and remained viable in centimeter-sized assembled tissue constructs when perfused for several days.
Directed assembly by tissue printing.
Tissue printing is an attractive scaffold free technique with the great potential of constructing delicate 3D tissue-like structures.Citation51–Citation61 (). An automated 3D bio-printer ideally is capable of generating a 3D computer model of organ, cutting the 3D model along a cross section to many 2D thin slices with cell thickness, and printing the slices layer by layer to construct the 3D tissue similar to the computer designed model using receiver's precursor cells. Recently, commercial 3D bio-printers are being developed for manufacturing human tissue and organs for research and surgical applications.Citation53 There has been considerable effort to print 3D tissue constructs to overcome the shortcomings of scaffold based tissue engineering and maintain the biomimetic architecture of microenvironment. Tissue printing usually combines methods of rapid prototyping with cell-gel encapsulation to print viable patterns using standard or modified printers.
To make complex 3D tissue constructs, a computer can be effectively used to control the position of printer head in Z-axis to place cells next to each other. This is like an inkjet printer with capability of printing in the third dimension, so in place of just making simple 2D tissue constructs, a 3D bioprinter is able to print more complex 3D tissues. This technique relies on capability of cells for self assembly when they are placed next to each other while suitable microenvironment such as natural cues and growth factors is provided. Clusters of several thousand of cells are encapsulated in microgels to form droplets which can be printed according to the desired 3D computer model. Cells in encapsulated microgels fuse, arrange themselves and self-assemble to form a tissue-like construct similar to the computer design when they are placed close to each other.
For sterile use bio-printer should fit inside a standard biosafety cabinet. Some of them have two print heads, one for placing cells and another for printing a hydrogel matrix. A typical bioprinter is equipped with a software interface to design the computer model of desired tissue and a laser calibration system, which may be controlled by computer to position the print head within micrometer precision.Citation53–Citation55
The applications of inkjet technology to tissue printing have been rapidly advanced in past few years; however the usage of inkjet tissue printing technique is hampered by some limitations including the size of the jetting needle, the cell concentration, the viscosity of the material and the viability of the printed cells.Citation56,Citation57 Although inkjet tissue printing has faced some shortcomings, there are some other jet-based techniques which can overcome disadvantages of the inkjet printing.
Electrospray or electrohydrodynamic jetting (EHDJ) and electrospinning are emerging alternatives to inkjet printing.Citation58,Citation59 EHDJ is a physical phenomenon which is generated by applying a potential difference between a grounded electrode and a needle filled with a liquid medium.Citation58 The medium exiting the needle goes through a high-intensity electric field where jet droplets will form. The size of jet droplets depends on the flow rate and properties of the liquid medium and the potential difference between electrode and the needle.Citation58,Citation59 EHDJ and Electrospinning have recently been successfully employed to print living cells by Jayasinghe et al.Citation57–Citation59 They could deposit cell droplets with a few microns diameter to make viable tissue constructs. Electrospraying and electrospinning could overcome the shortcomings of inkjet tissue printing such as size of droplets, resolution and cell viability. These techniques can be potentially employed for precise cell deposition with applications of tissue fabrication at the micrometer and nanometer scales.
Conclusions and Future Perspectives
Directed assembly of tissue constructs is an emerging strategy with appealing results for engineering functional tissues. This approach aims to mimic the structure of native tissues often constructed of multiple functional units organized in multiple scales with specific geometry to preserve tissue function. Encapsulated cells in hydrogels closely mimic tissue units which can be combined to make a large tissue construct with desired architecture and function through regulating the formation of local microenvironment while maintaining dynamic tissue remodeling. This can potentially be used to study the mechanisms of in vitro tissue organogenesis through designing tissue architectures capable of producing sufficient ECM and dynamic remodeling with predictable function.
In this method, populations of different cell types can be encapsulated in hydrogel blocks to form tissue units where these tissue units can be assembled to make a tissue construct with specific geometric patterns. Such technique preserves the desired shape and size of the microstructured building blocks while it provides a better control on spatial cell arrangement within these blocks.
Although microscale technologies enable precise control of the micro-architecture of the modular tissues, controlling the macro-architecture and cellular function of the tissue assembly remain as the major challenges of directed assembly-based tissue engineering. Other challenges to overcome in this field include: establishment of biomimetic microenvironments within the microgels (i.e., materials with biochemical and mechanical properties and functional microvasculature), scaling-up the assembly techniques for producing construct of tissue-level dimensions and successful integration and remodeling of the assembled tissues with the native tissue environment after transplantation. It is foreseen that directed tissue assembly possesses immense potential for advancing the field of tissue engineering and there will be increased integration of directed assembly-based approach with conventional scaffold-based approach to create more complex functional tissues with physiological architecture for clinical applications. In future in vitro directed assembly of cell-laden hydrogels from autologous cells can potentially eliminate allogenic organ transplantation, patient waiting lists and complications after transplantation due to immunosuppressive therapy.
Figures and Tables
Figure 1 Microscale hydrogels as tissue building blocks: (A–D) schematic diagram of the techniques to microengineer hydrogels with controlled size and shape: emulsification (A), photolithography (B), microfluidics (C) and micromolding (D). (E) Versatility in microgel shapes: microgels can be molded into various shapes such as square, prisms, disks and strings.Citation37
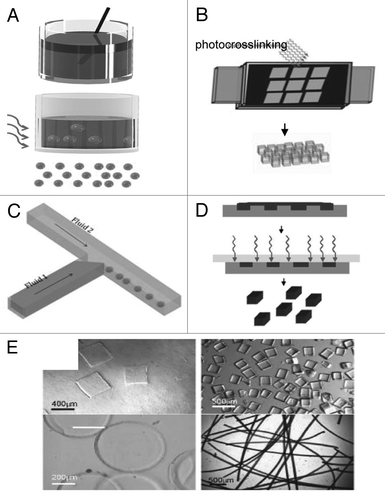
Figure 2 Directed assembly of cell-laden microgels at a two-phase interface. (A–F) Directed assembly in a two-phase reactor. (A and B) Schematic diagram of microgel assembly process: Microgel assembly in a dish containing mineral oil is induced by mechanical agitation (A). Secondary crosslinking is used to stabilize the microgel assemblies by a secondary photocrosslinking (B). Directed assembly of lock-and-key-shaped microgels with cross-shaped microgels stained with green and rod-shaped microgels stained with red (C and D) as well as cross-shaped microgels containing red-stained cells and rod-shaped microgels containing green-stained cells (E and F) (Scale bars, 200 µm). (G–M) Directed assembly on the surface of hydrophobic medium. (G) Microgels are initially randomly distributed on the surface of a high-density hydrophobic medium. Driven by surface tension, microgels self assembled into a sheet-like structure to minimize free energy. Secondary photopolymerization stabilized the structure by crosslinking the microgels to each other (scale bar: 2 cm). (H–J) Assembly of complex building blocks using a central microgel with one cell type surrounded by building blocks of a second cell type. (K–M) Assembly of complex building blocks with controlled co-culture using lock-and-key shaped microgels to better direct the assembly process (scale bar: 1 mm).
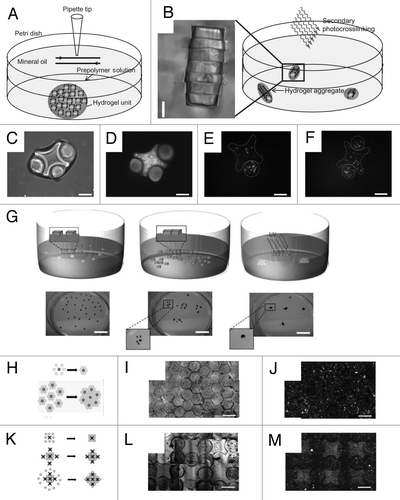
Figure 3 Directed assembly of cell-laden microgels on hydrophilic template (A–D). Surface-directed assembly of microgels: hydrophobic (OTS) patterns on a hydrophilic glass induce the directed assembly of the microgels on the hydrophilic patterns which are stabilized by secondary crosslinking (A). Microgel assembly can be achieved with different shapes defined by the patterns such as a square pattern (B) or “MIT” pattern (C). Assembling cell-laden microgels in shaped-defined bulk hydrogel with the red-labeled cells encapsulated in microgels and the green-labeled cells encapsulated in bulk hydrogel (D). (E–H) Directed assembly of microgels on the PDMS mold with high hydrophilic affinity (E) which can be used to generate microgel assembly with various shapes and complexities such as tube, solid ball, casquet (F) and double-layer tubes (G). Hepatocytes encapsulated in PEG microgels assembled to form a 5 mm diameter tube (Scale Bar: 1.5 mm) (H).
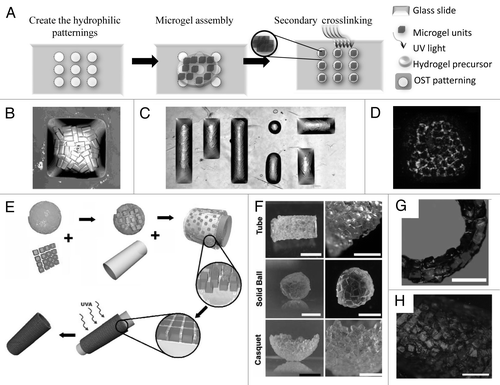
Figure 4 Directed assembly of cell-laden microgels within physical template. (A–D) Templated packing of glass beads (260 µm diameter) in microchannels (A) 2 mm, (B) 500 µm and (C) 250 µm wide. The beads are most ordered when confined to the narrowest channels. Templated assembly of gel modules containing three differently labeled groups of cells (D). (E) Microgel arrangement and assembly by a micromanipulator. Rhodamine (red) and FITC (green) stained cells are encapsulated in separate HA microgels and subsequently arranged in an alternating checkerboard pattern. (F–H) HepG2 cells encapsulated collagen modular units are coated with a confluent layer of endothelial cells and packed inside a perfused chamber (F). The resultant tissue assembly consists of interconnected cellular modules (G–H) which are perfusable and non-coagulative due to endothelialization.
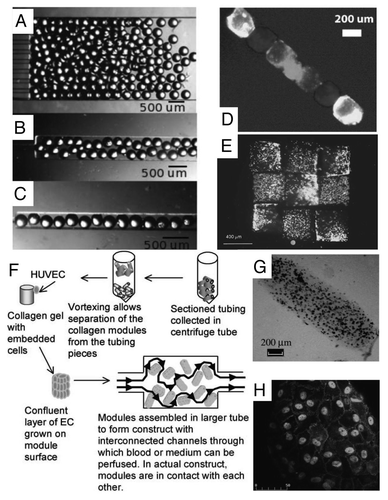
Figure 5 Microfluidic-guided assembly of cell-laden hydrogel building blocks. (A) Guided movement of microtrains along a sinusoidal-shaped rail in a microfluidic channel. (B) The complex self-assembly such as Greek temple was completed simultaneously by applying fluidic force inside a railed microfluidic channel. Each microstructure (37 microstructures in total) is fabricated independently on a corresponding rail. (C) Heterogeneous assembly process on the basis of the cross-solution movement scheme. (D) Assembled 3 × 3 matrix with two different living cells using cell-containing PEG-DA hydrogel building blocks.
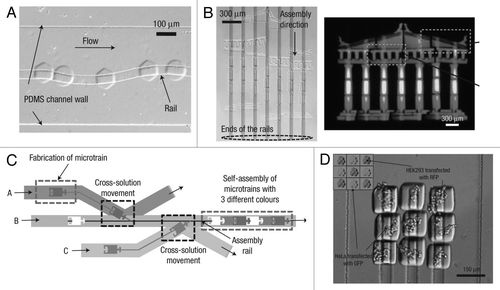
Figure 6 Tissue building blocks generated by tissue printing method. (A) Sequentially deposited collagen type I gel. (B) Manually printed tube with collagen type I. (C) Mathmatical model of aggregates before fusion. (D) Fused aggregates. (E) Ten aggregates before fusion. (F) Final disc-like structure after fusion.Citation42 (G) Computer aided design-based presentation model of cell printer. (H) Image of the actual cell printer and (I) part of the print head with nozzles.Citation42 (J and K) Macroscopic images of printed alginate cell tube constructs. (J) Constructs with SMC immediately after seeding. (K) SMC cell tube after 13 days of incubation.Citation61
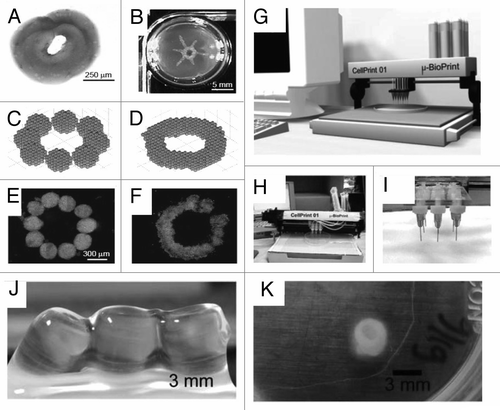
Table 1 Comparison of different techniques for generating hydrogel-based building blocks
Acknowledgements
This paper was partially supported by the National Institutes of Health (NIH EB007249, DE019024 and HL092836), the institute for Soldier Nanotechnology and the Charles Stark Draper Laboratory. Nezamoddin N. Kachouie is a recipient of Natural Sciences and Engineering Research Council of Canada Postdoctoral Fellowship (NSERC-PDF).
References
- Khademhosseini A, Vacanti JP, Langer R. Progress in tissue engineering. Sci Am 2009; 300:64 - 71
- Langer R, Vacanti JP. Tissue engineering. Science 1993; 260:920 - 926
- Mikos AG, Herring SW, Ochareon P, Elisseeff J, Lu HH, Kandel R, et al. Engineering complex tissues. Tissue Eng 2006; 12:3307 - 3339
- Nichol JW, Khademhosseini A. Modular tissue engineering: Engineering biological tissues from the bottom up. Soft Matter 2009; 5:1312 - 1319
- Bissell MJ, Radisky DC, Rizki A, Weaver VM, Petersen OW. The organizing principle: microenvironmental influences in the normal and malignant breast. Differentiation 2002; 70:537 - 546
- Bissell MJ, Rizki A, Mian IS. Tissue architecture: the ultimate regulator of breast epithelial function. Curr Opin Cell Biol 2003; 15:753 - 762
- Engler AJ, Humbert PO, Wehrle-Haller B, Weaver VM. Multiscale modeling of form and function. Science 2009; 324:208 - 212
- Ingber DE. Mechanical control of tissue growth: function follows form. Proc Natl Acad Sci USA 2005; 102:11571 - 11572
- Rivron NC, Rouwkema J, Truckenmuller R, Karperien M, De Boer J, Van Blitterswijk CA. Tissue assembly and organization: developmental mechanisms in micro-fabricated tissues. Biomaterials 2009; 30:4851 - 4858
- Guyton AC, Hall JE. Text Book of Medical Physiology 2006; 11th Edition
- Kim BS, Mooney DJ. Scaffolds for engineering smooth muscle under cyclic mechanical strain conditions. J Biomech Eng 2000; 122:210 - 215
- Mooney D, Hansen L, Vacanti J, Langer R, Farmer S, Ingber D. Switching from differentiation to growth in hepatocytes: control by extracellular matrix. J Cell Physiol 1992; 151:497 - 505
- Bottaro DP, Liebmann-Vinson A, Heidaran MA. Molecular signaling in bioengineered tissue microenvironments. Ann NY Acad Sci 2002; 961:143 - 153
- Hollister SJ. Porous scaffold design for tissue engineering. Nat Mater 2005; 4:518 - 524
- Grande DA, Halberstadt C, Naughton G, Schwartz R, Manji R. Evaluation of matrix scaffolds for tissue engineering of articular cartilage grafts. J Biomed Mater Res 1997; 34:211 - 220
- Schreiber RE, Dunkelman NS, Naughton G, Ratcliffe A. A method for tissue engineering of cartilage by cell seeding on bioresorbable scaffolds. Ann NY Acad Sci 1999; 875:398 - 404
- Levenberg S, Burdick JA, Kraehenbuehl T, Langer R. Neurotrophin-induced differentiation of human embryonic stem cells on three-dimensional polymeric scaffolds. Tissue Eng 2005; 11:506 - 512
- Elbert DL, Hubbell JA. Self-assembly and steric stabilization at heterogeneous, biological surfaces using adsorbing block copolymers. Chem Biol 1998; 5:177 - 183
- Livoti CM, Morgan JR. Self-assembly and tissue fusion of toroid-shaped minimal building units. Tissue Eng Part A 2010; 16:2051 - 2061
- Rago AP, Chai PR, Morgan JR. Encapsulated arrays of self-assembled microtissues: an alternative to spherical microcapsules. Tissue Eng Part A 2009; 15:387 - 395
- Khademhosseini A, Langer R, Borenstein J, Vacanti JP. Microscale technologies for tissue engineering and biology. Proc Natl Acad Sci USA 2006; 103:2480 - 2487
- Nelson CM, Tien J. Microstructured extracellular matrices in tissue engineering and development. Curr Opin Biotechnol 2006; 17:518 - 523
- Nguyen KT, West JL. Photopolymerizable hydrogels for tissue engineering applications. Biomaterials 2002; 23:4307 - 4314
- Burdick JA, Mason MN, Hinman AD, Thorne K, Anseth KS. Delivery of osteoinductive growth factors from degradable PEG hydrogels influences osteoblast differentiation and mineralization. J Control Release 2002; 83:53 - 63
- Sakiyama SE, Schense JC, Hubbell JA. Incorporation of heparin-binding peptides into fibrin gels enhances neurite extension: an example of designer matrices in tissue engineering. FASEB J 1999; 13:2214 - 2224
- Burdick JA, Chung C, Jia X, Randolph MA, Langer R. Controlled degradation and mechanical behavior of photopolymerized hyaluronic acid networks. Biomacromolecules 2005; 6:386 - 391
- Khademhosseini A, May MH, Sefton MV. Conformal coating of mammalian cells immobilized onto magnetically driven beads. Tissue Eng 2005; 11:1797 - 1806
- Halstenberg S, Panitch A, Rizzi S, Hall H, Hubbell JA. Biologically engineered protein-graft-poly(ethylene glycol) hydrogels: A cell adhesive and plasmin-degradable biosynthetic material for tissue repair. Biomacromolecules 2002; 3:710 - 723
- Elisseeff J, McIntosh W, Fu K, Blunk BT, Langer R. Controlled-release of IGF-I and TGF-beta1 in a photopolymerizing hydrogel for cartilage tissue engineering. J Orthop Res 2001; 19:1098 - 1104
- Khademhosseini A, Langer R. Microengineered hydrogels for tissue engineering. Biomaterials 2007; 28:5087 - 5092
- Koh WG, Revzin A, Pishko MV. Poly(ethylene glycol) hydrogel microstructures encapsulating living cells. Langmuir 2002; 18:2459 - 2462
- Liu VA, Bhatia SN. Three-dimensional photopatterning of hydrogels containing living cells. Biomed Microdevices 2002; 4:257 - 266
- Peppas NA, Hilt JZ, Khademhosseini A, Langer R. Hydrogels in biology and medicine: From molecular principles to bionanotechnology. Adv Mater 2006; 18:1345 - 1360
- Panda P, Ali S, Lo E, Chung BG, Hatton TA, Khademhosseini A, et al. Stop-flow lithography to generate cell-laden microgel particles. Lab Chip 2008; 8:1056 - 1061
- Ling Y, Rubin J, Deng Y, Huang C, Demirci U, Karp JM, Khademhosseini A. A cell-laden microfluidic hydrogel. Lab Chip 2007; 7:756 - 762
- Khademhosseini A, Eng G, Yeh J, Fukuda J, Blumling J, Langer R, et al. Micromolding of photocrosslinkable hyaluronic acid for cell encapsulation and entrapment. J Biomed Mater Res A 2006; 79:522 - 532
- Yeh J, Ling YB, Karp JM, Gantz J, Chandawarkar A, Eng G, et al. Micromolding of shape-controlled, harvestable cell-laden hydrogels. Biomaterials 2006; 27:5391 - 5398
- Fukuda J, Khademhosseini A, Yeo Y, Yang X, Yeh J, Eng G, et al. Micromolding of photocrosslinkable chitosan hydrogel for spheroid microarray and co-cultures. Biomaterials 2006; 27:5259 - 5267
- Karp JM, Yeh J, Eng G, Fukuda J, Blumling J, Suh KY, et al. Controlling size, shape and homogeneity of embryoid bodies using poly(ethylene glycol) microwells. Lab Chip 2007; 7:786 - 794
- Franzesi GT, Ni B, Ling YB, Khademhosseini A. A controlled-release strategy for the generation of cross-linked hydrogel microstructures. J Am Chem Soc 2006; 128:15064 - 15065
- Lee W, Lee V, Polio S, Keegan P, Lee JH, Fischer K, et al. On-demand three-dimensional freeform fabrication of multi-layered hydrogel scaffold with fluidic channels. Biotechnol Bioeng 105:1178 - 1186
- Mironov V, Boland T, Trusk T, Forgacs G, Markwald RR. Organ printing: computer-aided jet-based 3D tissue engineering. Trends Biotechnol 2003; 21:157 - 161
- Du Y, Lo E, Ali S, Khademhosseini A. Directed assembly of cell-laden microgels for fabrication of 3D tissue constructs. Proc Natl Acad Sci USA 2008; 105:9522 - 9527
- Zamanian B, Masaeli M, Nichol JW, Khabiry M, Hancock MJ, Bae H, et al. Interface directed self assembly of cell-laden microgels. Small 2010; 6:937 - 944
- Du Y, Ghodousi M, Lo E, Vidula MK, Emiroglu O, Khademhosseini A. Surface-directed assembly of cell-laden microgels. Biotechnol Bioeng 105:655 - 662
- Fernandez JG, Khademhosseini A. Micro-masonry: Construction of 3D structures by microscale self-assembly. Adv Mater 2010; 22:2538 - 2541
- Bruzewicz DA, McGuigan AP, Whitesides GM. Fabrication of a modular tissue construct in a microfluidic chip. Lab Chip 2008; 8:663 - 671
- McGuigan AP, Sefton MV. Vascularized organoid engineered by modular assembly enables blood perfusion. Proc Natl Acad Sci USA 2006; 103:11461 - 11466
- Chung SE, Park W, Shin S, Lee SA, Kwon S. Guided and fluidic self-assembly of microstructures using railed microfluidic channels. Nat Mater 2008; 7:581 - 587
- Liu B, Liu Y, Lewis AK, Shen W. Modularly assembled porous cell-laden hydrogels. Biomaterials 31:4918 - 4925
- Mironov V, Visconti RP, Kasyanov V, Forgacs G, Drake CJ, Markwald RR. Organ printing: tissue spheroids as building blocks. Biomaterials 2009; 30:2164 - 2174
- Neagu A, Forgac G. Vossoughi J. Fusion of cell aggregates: a mathematical model. Biomedical Engineering. Recent Development 2002; Washington, DC Medical and Engineering Publishers, Inc
- http://www.organovo.com
- Making a Bit of me: A machine that prints organs is coming to market. Economist 2010; 2
- Norotte C, Marga F, Niklason L, Forgacs G. Scaffold-free vascular tissue engineering using bioprinting. Biomaterials 2009; 30:5910 - 5917
- Nair K, Gandhi M, Khalil S, Yan KC, Marcolongo M, Barbee K, et al. Characterization of cell viability during bioprinting processes. Biotechnol J 2009; 4:1168 - 1177
- Jayasinghe SN. Bio-electrosprays: the development of a promising tool for regenerative and therapeutic medicine. Biotechnol J 2007; 2:934 - 937
- Jayasinghe SN, Qureshi AN, Eagles PA. Electrohydrodynamic jet processing: an advanced electric-field-driven jetting phenomenon for processing living cells. Small 2006; 2:216 - 219
- Townsend-Nicholson A, Jayasinghe SN. Cell electrospinning: a unique biotechnique for encapsulating living organisms for generating active biological micro-threads/scaffolds. Biomacromolecules 2006; 7:3364 - 3369
- Wilson WC Jr, Boland T. Cell and organ printing 1: protein and cell printers. Anat Rec 2003; 272:491 - 496
- Kesari P, Xu T, Boland T. Layer-by-layer printing of cells and its application to tissue engineering. Nanoscale Mater Sci Biol Med 2005; 111 - 117
- Hahn MS, Miller JS, West JL. Three-dimensional biochemical and biomechanical patterning of hydrogels for guiding cell behavior. Adv Mater 2006; 18:2679
- Luo Y, Shoichet MS. A photolabile hydrogel for guided three-dimensional cell growth and migration. Nat Mater 2004; 3:249 - 253
- Roh KH, Martin DC, Lahann J. Biphasic Janus particles with nanoscale anisotropy. Nat Mater 2005; 4:759 - 763
- Burdick JA, Khademhosseini A, Langer R. Fabrication of gradient hydrogels using a microfluidics/photopolymerization process. Langmuir 2004; 20:5153 - 5156
- Dendukuri D, Pregibon DC, Collins J, Hatton TA, Doyle PS. Continuous-flow lithography for high-throughput microparticle synthesis. Nat Mater 2006; 5:365 - 369
- Dendukuri D, Tsoi K, Hatton TA, Doyle PS. Controlled synthesis of nonspherical microparticles using microfluidics. Langmuir 2005; 21:2113 - 2116
- Pregibon DC, Toner M, Doyle PS. Multifunctional encoded particles for high-throughput biomolecule analysis. Science 2007; 315:1393 - 1396
- Stachowiak AN, Bershteyn A, Tzatzalos E, Irvine DJ. Bioactive hydrogels with an ordered cellular structure combine interconnected macroporosity and robust mechanical properties. Adv Mater 2005; 17:399
- Golden AP, Tien J. Fabrication of microfluidic hydrogels using molded gelatin as a sacrificial element. Lab Chip 2007; 7:720 - 725