Abstract
The major role of the eye lens is to transmit and focus images onto the retina. For this function, the lens needs to develop and maintain the correct shape, notably, the precise curvature and high-level order and organization of its elements. The lens is mainly comprised of highly elongated fiber cells with hexagonal cross-sectional profiles that facilitate regular packing. Collectively, they form concentrically arranged layers around the anterior-posterior polar axis, and their convex curvature contributes to the spheroidal shape of the lens. Although the lens has been a popular system for developmental studies, little is known about the mechanism(s) that underlies the development of its exquisite three-dimensional cellular architecture. In this review, we will describe our recent work, which shows how planar cell polarity (PCP) operates in lens and contributes to its morphogenesis. We believe that the lens will be a useful model system to study PCP in general and gain insights into mechanisms that generate high-level cellular order during development.
Introduction
Coordinating cells so that they move/orient/proliferate in a particular direction is clearly critical for normal morphogenesis of most, if not all, tissues and organs. This phenomenon is generally known as planar cell polarity (PCP) and was originally reported in epithelial sheets, notably in Drosophila wing (see review in this issue by Maung et al.). Given the growing recognition of the importance of cell-cell interactions for aligning cells with neighbors and polarizing cues for controlling their global orientation along tissue axes, there is intense interest in investigating the role of PCP signaling in regulating such phenomena. Elucidation of the fundamentals of PCP that operate in the various developmental systems will help identify common principals (and differences) that operate throughout the animal kingdom. Here, we review our studies on PCP in the mammalian lens.
The lens has a number of advantages for developmental studies, not the least of which is that it is comprised only of lens cells; blood vessels, nerves and connective tissue are absent. Lens cells are present in two main forms: squamous-cuboidal epithelial cells and highly elongated fiber cells.Citation1 We recently found that each fiber cell has a primary cilium on its hexagonally shaped apical surface, and that it is polarized to the side of each cell that faces the anterior pole.Citation2 There is also evidence that polarized behavior of lens fibers is regulated by core PCP proteins, since fiber cell alignment and orientation is disrupted in mice with mutations of genes coding for some of these proteins. Furthermore, the Wnt/Fz antagonist Sfrp2 disrupts fiber alignment, resulting in a phenotype that is reminiscent of Drosophila wing cell mutants.Citation2,Citation3 Thus, we feel that the lens, a relatively new member to the vertebrate PCP club, will provide a very useful system to resolve some fundamental issues in PCP signaling, especially for mammalian systems with requirements for very precise alignment and orientation of their cellular elements.
Lens Morphogenesis and Growth
The mammalian lens is an ectodermally derived tissue predominantly made up of highly elongated fiber cells that are regularly packed to form a spheroidal mass (). Anteriorly, the fibers are covered by a monolayer of cuboidal epithelial cells, and the complete structure is wrapped up in a thick basement membrane, the lens capsule. During embryogenesis, lenses arise from ectoderm situated next to bilateral evaginations of the neural tube, the optic vesicles (). Ectoderm situated next to the optic vesicle thickens to form the lens placode. This then invaginates together with the optic vesicle to form the lens pit and optic cup, respectively. The lens pit deepens and eventually pinches off from the surface to form the lens vesicle ( and D). Subsequently, posterior lens vesicle cells undergo coordinated elongation and differentiate into the primary fibers, whereas anterior vesicle cells give rise to a sheet of cuboidal epithelial cells ().Citation1 Primary fibers continue to elongate until their apical tips attach to the anterior epithelial layer so that the lens vesicle lumen is finally lost (). Thus, the divergent fates of these embryonic cells give the lens its distinctive polarity.
From this stage onwards, the lens grows rapidly by cell proliferation and differentiation. Epithelial cells divide and serve as progenitors for secondary fiber cells that initiate differentiation at the lens equator and progressively become added to the fiber mass ().Citation4–Citation6 Cells are not sloughed off from the lens, so lens growth continues throughout life as successive layers of secondary fibers accumulate; however, the nucleus of the lens remains comprised of primary fibers that arose during embryogenesis ().
Although the lens is comprised of only two types of cells, they are subdivided functionally into several zones (). During early stages of development, cells in the epithelial layer are relatively homogeneous in terms of proliferative activity, but as development proceeds, epithelial cell proliferation becomes progressively restricted to a zone just above the lens equator adjacent to the ciliary body known as the germinative zone (GZ).Citation4–Citation6 Most of the central epithelial (CE) cells enter a quiescent state (). As cell number increases by proliferation, epithelial cells migrate or are dislocated toward the equator where they withdraw from the cell cycle and start elongating and differentiating into lens fibers (TZ, transitional zone). Their protein expression profile changes dramatically during differentiation; for example, epithelial-specific proteins Pax6, E-cadherin, aPKC, Par3 and Par6 disappear, and fiber-specific proteins like β- and γ-crystallins, intermediate filaments CP49 and filensin begin to accumulate.Citation1,Citation7,Citation8 Furthermore, isotype changes are also evident; epithelial cells are positive for aquaporin 1 and Connexin 43, but these switch to aquaporin 0 (also known as major intrinsic protein; MIP) and Connexins 46 and 50, respectively.Citation9 N-cadherin and α-crystallins are common to both epithelial and fiber cells.
At the lens equator, early differentiating fibers rotate along their apical-basal axis through about 90° and then start migrating, anteriorly along the lens epithelium and posteriorly along the capsule, toward the lens poles ( and ). During this process, their curvature changes from convex at the equator to concave in the outer cortical fibers (OCF). Subsequently, the inner cortical fibers (ICF) start to lose cellular organelles, i.e., Golgi, mitochondria and nuclei, components that might cause light scattering.Citation10
Fiber cells are hexagonal in cross-section and are arranged in a honeycomb-like pattern, forming an array of regularly aligned, concentric rings (). As fibers form all around the lens equator, they eventually meet and form end-to-end associations with equivalent fibers from other segments of the lens. Precise alignment/orientation of fibers results in formation of distinct suture lines (), and in rodents, as well as in many other mammalian species, these are characteristically Y-shaped.Citation11 As the lens grows by addition of successive waves of fiber cells, the suture lines that are formed reflect a history of ordered cell migration, alignment and orientation.
Regulation of Fiber Differentiation
As fiber differentiation is central to lens formation, considerable effort has been directed at elucidating its regulation. Recognition that epithelial cells elongate and differentiate into secondary fiber cells once they shift below the lens equator and become exposed to vitreous led to the quest for the “fiber differentiation factor” in this ocular medium. From extensive in vitro and in vivo studies, there is now compelling evidence that some as-yet-unspecified member(s) of the FGF growth factor family provides the stimulus for initiation of this process.Citation12–Citation17 Our studies have shown that FGF can induce lens epithelial cell proliferation, migration and fiber differentiation in a dose-dependent manner.Citation14 This has led us to hypothesize that in situ, lens growth patterns are regulated by a “FGF gradient” in the form of the differential distribution of FGF bioavailability in the eye.Citation1 In this model, schematically shown in , proliferating lens epithelial cells are exposed to lower levels of FGF activity in aqueous, whereas differentiating fiber cells are exposed to higher levels of FGF activity in the vitreous. FGF is present in both ocular media, but, consistent with our model, much more FGF is recoverable from vitreous than aqueous.Citation18 Thus, epithelial cells that shift below the equator become exposed to a higher concentration of FGF in vitreous and, as they elongate, become progressively incorporated into the fiber mass. This mechanism ensures that the lens retains its polarity as it grows throughout life.
FGF induces fiber differentiation when the required amount of recombinant protein (usually 100 ng/ml FGF2) is added to cultures of lens epithelial explants (). The epithelial cells withdraw from the cell cycle then initiate elongation and expression of fiber-specific proteins. However, in this context, elongating fibers do not exhibit the global alignment and orientation observed in vivo. Although small groups of aligned fibers often show similar orientation, different groups point in different directions (). Thus it appears that an intrinsic property of fibers promotes their coordinated behavior locally as they elongate in vitro; however, to achieve this globally, they appear to require some other overriding regulatory mechanism that only operates in vivo. Alignment and regular packing of fibers is likely to be facilitated by their hexagonal shape. As they elongate in situ the fibers also undergo directed migration at their apical and basal tips and orient appropriately to develop the curvature that is needed for generation of spheroidal lens shape. Clearly this involves regulating complex and dynamic cytoskeletal processes within individual cells as well as coordinating these processes within large fields of elongating fibers at similar stages of differentiation. Given the growing awareness of the importance of PCP signaling in regulating and coordinating oriented cell behavior in many developing systems, this pathway seemed a likely candidate for regulating at least some of these processes in lens development.
PCP in Lens Development
PCP is regulated by the so called non-canonical Wnt pathway and involves transmembrane Frizzled (Fz) receptors and downstream components, including Dishevelleds (Dvls), Daam1, Rho family small GTPases and JNK.Citation19 As Wnts are well-known ligands for Fz receptors in the canonical β-catenin signaling pathway, it was thought they may also have a role in establishing PCP. Although experimental evidence does not support such a role for Wnts in Drosophila,Citation20,Citation21 by contrast, in vertebrates, there are now many instances of Wnts regulating PCP signaling,Citation21,Citation22 and, indeed, there are now a few examples where Wnt gradients regulate PCP and provide global polarizing signals.Citation23,Citation24
Wnt/β-Catenin Pathway in Lens
Expression of Wnt pathway components has been extensively reported during lens development; for example, all Fzs (1–10), all Dvls (1–3), many Wnts and Wnt signaling regulators are expressed during lens morphogenesis.Citation25–Citation29 This observation initially led to investigations into the canonical Wnt/β-catenin pathway in lens development. Several transgenic mouse lines are available to detect endogenous Wnt signaling. Of these, the well-established reporter mouse lines TOP-galCitation30 and BAT-galCitation31 have never shown reporter activity at any stage of lens development. In contrast, studies using the TCF/Lef-LacZ reporter mouse have consistently shown a short phase of canonical signaling in most of the anterior cells of the lens vesicle from about E11.5-14.5.Citation32,Citation33 Outside of this, no reporter activity was detected in any of the other stages examined, E9.5 through to P0. Our own recent studies with this mouse line have also shown reporter activity in most of the anterior epithelial cells through stages E11.5–14.5; however, reporter activity was also detected in cells scattered throughout the epithelium at P0. Consistent with all other studies, no reporter activity was detected in fibers during embryonic development. Also, no reporter activity was detected in fibers in any of the postnatal stages examined (P15–40; Sugiyama Y et al. unpublished; ).
Results from functional studies are also consistent with this pattern. Indeed, for lens primordium induction, it appears that the Wnt/β-catenin pathway needs to be inhibited, as shown in experiments with transgenic mice that overexpressed constitutively active β-catenin in the presumptive lens region.Citation34 In these mice, lens induction was abolished, whereas in β-catenin-knockout mice, lens fate markers appeared, and the lens placode formed normally. Although lens formation begins in these β-catenin-knockout mice, morphogenesis is aberrant.Citation31,Citation34 Consistent with a role for β-catenin signaling in early stages of lens morphogenesis, mice with a mutation in lrp6 (an essential co-receptor for Fz in the canonical pathway) also show aberrant lens development, with the major defect being incomplete differentiation of the lens epithelial layer.Citation25 Together with the observation of reporter activity between E11.5 and E14.5 in the TCF/Lef reporter mice, this indicates a requirement for canonical signaling in the anterior cells of the lens vesicle for a short period, presumably to promote lens epithelial cell differentiation/maintenance.
Evidence for Wnt-Fz/PCP Signaling in the Lens
The characteristic spheroidal lens shape is established by E12.5, when coordinated elongation of primary fiber cells results in formation of a polarized lens structure. Thereafter, secondary fiber cells start to differentiate, increasing lens size by elongating and progressively adding new layers to the nucleus of primary fibers. As already described, the secondary fiber cells exhibit highly ordered patterns of alignment and orientation as they become added to the fiber mass. Where fibers from different segments of the lens meet at their poles, they form associations known as sutures, and these begin to be detected in mice around E16.5 (Sugiyama Y et al., unpublished).
Involvement of the PCP pathway in lens formation is indicated by the defective cell alignment and suture formation that is evident in the lenses of Looptail (Lp) and Crash (Crsh) mice. These mice have mutations in core PCP genes Van Gogh-like 2 (Vangl2) and Celsr1, respectively and are commonly used for assessing roles for PCP signaling in development.Citation35 Although lens formation appears to begin normally in Lp mice, i.e., lens epithelial and fiber cells differentiate to form the characteristic lens shape, defects in fiber cell organization become obvious by E16.5. By this stage, fibers do not show normal alignment and orientation in sagittal sections and do not form sutures at the anterior pole; these defects become more pronounced as development proceeds (). Crsh mice also show similar lens fiber defects.Citation2 These results indicate that lens fiber alignment and orientation is regulated by the PCP signaling pathway.
The central theme of PCP is coordination of polarization within the plane, and this is partly achieved through asymmetric recruitment of particular PCP proteins to specific cellular domains.Citation36–Citation39 This asymmetry was first recognized in insects, with Drosophila wing being a frequently cited example to show that Fz and Dvl (Dsh, in invertebrates) accumulate along the distal side of the hexagonally shaped cells, whereas Van Gogh (Vang) and Prickle (Pk) accumulate on the proximal side of cells. In lens cells, PCP proteins also show asymmetric distribution on the apical surfaces of lens fibers ( and F).Citation2 At the apical tips of the hexagonally shaped OCF, Fz6 and Vangl2 predominately associate with the short side that is proximal to the anterior pole of the lens, whereas Pk1 predominantly associates with the long sides of each cell, with some weak cytoplasmic reactivity also present. Dvl2 and Dvl3 tend to be localized to all sides of each cell, with a little more intense reactivity often associated with the short side that is proximal to the anterior pole of the lens. Dvl2 and Dvl3 also show some particulate localization in the cytoplasm. Inversin (Inv) localizes to the cell margin that is proximal to the anterior pole of the lens. This is consistent with Inv being a protein that is associated with cilia (see later).Citation40 Such polarized distribution of core PCP proteins in lens fibers and their reciprocal localization patterns, especially between Fz6/Vangl2 and Pk1, suggests Fz/PCP pathway involvement in regulating aspects of fiber cell alignment.
Polarized Primary Cilia in Lens Cells
As polarization of the primary cilium/basal bodies has been shown to occur during the establishment of PCP,Citation41 this morphological feature can provide a useful marker for this pathway. Although lens fiber cells are well-known for their highly ordered alignment and have a honeycomb packing arrangement reminiscent of the Drosophila wing, it was shown only recently, using whole mount preparations, that each fiber has a polarized primary cilium, and importantly, during fiber differentiation, these become globally oriented toward the anterior pole of the lens,Citation2 thus providing direct evidence that PCP operates in the lens. In general, apical surfaces of the lens fibers are not readily visualized, since they are closely attached to the epithelial cell surface (see and ). However, when epithelial whole-mount preparations are made, the apical tips of the fibers often remain attached to the surface of the epithelial cells and so can be viewed “en masse” by confocal microscopy. This method enabled us to detect the polarization of the cilium/centrosome in the lens fiber cells.Citation42 This polarization first becomes evident in the TZ, where epithelial-fiber differentiation is initiated and the cells begin to elongate and align in regular rows (). As differentiation progresses in the lens cortex, polarization is readily observed, and virtually all OCF show a polarized cilium/centrosome. In the inner cortical fibers (ICF), the cilium/centrosome, although prominent and polarized in some cells, appears more randomly distributed indicating that polarity progressively diminishes as fibers continue maturation in this region.
A degree of asymmetric localization of the cilium/centrosome is also evident in lens epithelial cells. In the central epithelium (CE), some cells show polarized distribution of the cilium/centrosome; however, in the proliferative GZ, no polarization is evident ().
These observations may indicate an important role for primary cilia in PCP formation in the lens, particularly in the OCF region. Links between polarization of cilia and PCP have already been established in other systems.Citation43–Citation45 Given that primary cilia have been reported to function as antenna to detect extracellular signals,Citation46,Citation47 one intriguing possibility in the lens is that the asymmetrically localized cilia in the fibers are involved in receiving a guidance signal from the anterior pole of the lens.
An Extracellular Signaling Cue to Guide Lens Fiber Cell Migration?
The idea for the existence of extracellular guidance cues in lens came from analysis of transgenic mice that overexpress secreted frizzled-related protein 2 (Sfrp2) specifically in the lens.Citation3 Although Sfrps can have diverse functions depending on context,Citation48 a well-characterized role is to antagonize Wnt cascades by binding to Wnts or Fz receptors.Citation49 The overexpression of Sfrp2 in fiber cells led to the development of cataracts. Lens fiber elongation was attenuated, and at their anterior and posterior tips, they did not orient or migrate toward the lens poles (). As a result, fibers did not develop the characteristic convex curvature that is central to the development of normal three-dimensional lens architecture. Microtubules, microfilaments and intermediate filaments were disorganized in the fiber cells of these transgenic mice. In addition, components of the PCP pathway, including Dvl, Cdc42, Rac1, RhoA and JNK were disturbed and, in the case of Dvl and the small GTPases, were downregulated/inhibited.Citation3 Immunoprecipitation experiments also provided evidence that Sfrp2 was associated with Wnt ligands in these lenses.Citation3 Overall, this result is consistent with Wnt-Fz/PCP signaling having a role in regulating the complex organization of the cytoskeleton that is required for normal lens fiber differentiation and maturation.
Importantly, while groups of fibers in Sfrp2-overexpressing lenses showed local coordination of alignment/orientation and polarization of cilia, this did not operate globally, and groups of locally aligned fibers often oriented in different directions. This resulted in aberrant suture-like arrangements being commonly observed in these lenses ().Citation2 This aberrant pattern of global (but not local) alignment is reminiscent of the phenotypes in the wing hair and body hair patterns in many of the Drosophila and mouse PCP mutants. In these, global patterning is disrupted, but there is still evidence of local ordering ().Citation50,Citation51 This suggests that excess Sfrp2 in the lens disturbs the overall coordination of alignment of fibers by disrupting global planar polarity that is regulated by a Fz/PCP pathway.
One interpretation of this result is that overexpressed Sfrp2 nullifies a global polarizing signal (GPS). We propose that this is a Wnt ligand that is normally concentrated at the anterior pole of the lens, and this coordinates global alignment/orientation of fiber cells to the poles so that they form sutures ( and B). Overexpressed Sfrp2 may associate with Fz receptors on the fibers and block signaling events (); alternatively, the excess Sfrp2 may sequester Wnts and disturb their normal distribution pattern (). Either way, the guidance cue would be abrogated. There are several candidates for the guidance cue, as we and others have shown that numerous Wnts are expressed in the lens.Citation25–Citation29
Potential Targets of PCP Pathway
By using the phenotype of the lens of mutant mice as an indicator, it may be possible to identify genes that interact with the PCP pathway. For example, analysis of the Abl-interactor-2 (Abi2)-knockout mouse indicates that Abi2 has an important role in regulating cytoskeletal dynamics in lens fibers.Citation52 This mouse has a remarkably similar lens phenotype to the overexpressing Sfrp2 mouse, in that lens fibers do not develop the convex curvature characteristic of the normal lens.Citation3 Interestingly, Abi2 protein also shows asymmetric distribution on the short side of each fiber, similar to that for Fz6 and Vangl2.Citation2 Thus, Abi2 may be one of the downstream targets of PCP signaling pathway. It has also been reported that the Discs-large (Dlg)-knockout mouse has abnormal fiber alignment and curvature.Citation53 Involvement in regulating PCP in the lens is also a possibility, given evidence from previous studies indicating a role for Dlg in establishing polarized cell behavior in Drosophila.Citation54
Future Directions
Although the highly ordered alignment and orientation of fibers has long been recognized, and its organization has been described in detail, it is only recently that the PCP pathway has been implicated in coordinating development of its three-dimensional cellular architecture. Given the high level of cellular order and organization required for lens function and because of its relatively simple cellular composition, we expect that the lens will provide a useful model system to examine some of the major outstanding questions in PCP biology. For example, key questions of general relevance that we are addressing at the moment include (1) Is there a guidance signal (e.g., our putative GPS) that induces directed migration and orientation of lens cells? (2) If such a guidance signal exists, what is its identity? (3) Does the primary cilium work as a cellular antenna that picks up this putative guidance cue? and (4) Does the primary cilium interact with PCP signaling components to achieve polarized cell behavior?
Understanding mechanisms that drive PCP in the lens also has important implications for dealing with some of its clinical problems. Cataract is a common disease, where lens transparency is compromised. During surgery, the opaque fiber mass is removed, and an artificial lens is fitted within the lens capsular bag. Although generally very effective in restoring visual acuity, as with all surgery, complications occur, and one of the most common is posterior capsule opacification (PCO).Citation55 Essentially, this is the development of a secondary cataract from aberrant proliferation and differentiation of residual epithelial cells that remain behind after surgery. Now, with our growing knowledge of factors that guide cells down the normal differentiation pathway, there is the potential to promote lens regeneration after cataract surgery. While administration of FGF is the key to initiating the fiber differentiation process, effective lens regeneration strategies will require information on how to promote the assembly of the differentiating fibers into the ordered three-dimensional structure that transmits and focuses light onto retina. A better understanding of how PCP signaling operates in the normal lens will provide background information that will help us make progress toward this goal.
Figures and Tables
Figure 1 Lens morphogenesis during embryogenesis. The lens (A) arises from the surface ectoderm adjacent to the optic vesicles, outpocketings of the neural tube. This ectoderm thickens to form the lens placode (B, at E9.5 in mouse) and then invaginates to form the lens pit (C, E10.5). The lens pit finally separates from the ectoderm to form the lens vesicle (D, E11.5). Posterior epithelial cells of the lens vesicle cease dividing and start elongating toward the anterior surface, eventually closing the lumen of the lens vesicle (E, E12.5). These elongating cells are called primary fibers and constitute the central region of mature lens (indicated with light gray in D–F). Epithelial cells of the lens vesicle continue to proliferate as the lens expands, while those below the lens equator stop dividing and begin their program of differentiation into secondary fiber cells (E, in dark gray). This begins about E12.5,Citation6 and these newly formed secondary fibers progressively accumulate around the central mass of primary fibers, so that eventually the primary fibers are internalized and comprise the lens nucleus (F). At the equator, the apical tips of differentiating fibers form a fulcrum as they elongate and rotate through their apical-basal axis by about 90° (A).
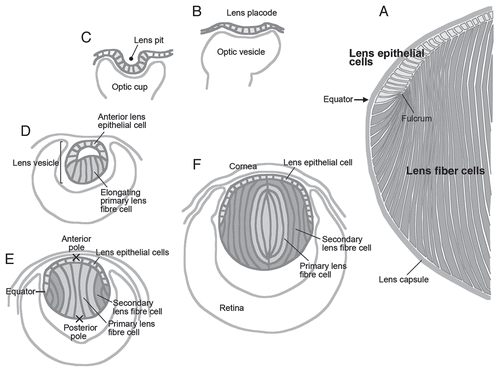
Figure 2 Cellular compartments of the lens. (A) Sagittal center section of a neonatal mouse eye. Lens epithelial cells are divided into central epithelium (CE) and germinative zone (GZ). Lens fibers are divided into transitional zone (TZ), outer cortical fibers (OCF), inner cortical fibers (ICF) and nuclear fibers (NF) according to their relative location. (B and C) Horizontal sections from regions indicated by the horizontal gray lines in the lens diagram (A). Suture lines are visible in horizontal sections taken through the anterior pole (B), whereas ordered alignment of hexagonal cross sections of fibers are observed in the horizontal sections taken through the equator (C). Adapted from Sugiyama et al.Citation2
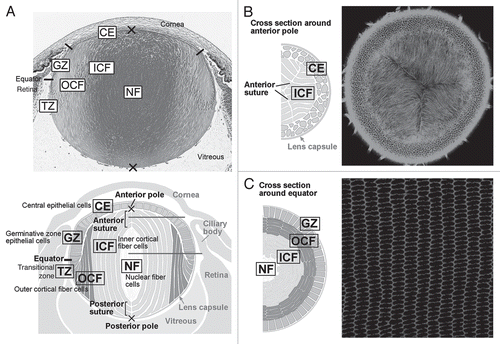
Figure 3 FGF, the key factor for lens fiber differentiation. (A) In the ocular environment, there is a gradient of FGF bioactivity, e.g., more in vitreous than in aqueous. (B) Rat lens epithelial cells in explant culture maintain their characteristic cobblestone-like appearance in serum-free medium. (C) A fiber differentiating dose of FGF causes the dramatic morphological transformation of cuboidal epithelial cells into elongated fiber cells. Cultured cells are visualized with immunostaining of β-catenin (to show cell boundaries) and pericentrin (to show primary cilium/centrosome location). Each primary cilium/centrosome is located at the apical tip of a fiber; their presence in clusters (C) indicates groups of elongating fibers with similar orientation (arrows). However, the primary cilium/centrosome clusters are widely scattered throughout the explant, indicating that different groups of fibers orient in different directions; i.e., orientation is not globally coordinated.
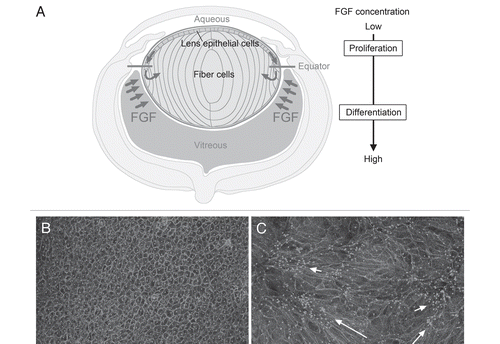
Figure 4 Restricted activation of canonical Wnt/β-catenin pathway during lens development. Mouse TCF/lef reporter gene assay shows transient strong Wnt/β-catenin pathway activity in most anterior lens epithelial cells around E13.5 (A). Reporter activity is absent from lens fibers at E13.5 (A) and P3 (B). In contrast, the reporter gene activity first detected in the peripheral region of the optic cup (A) extends through embryonic development and remains prominent in this region postnatally, particularly where the ciliary body differentiates (B).
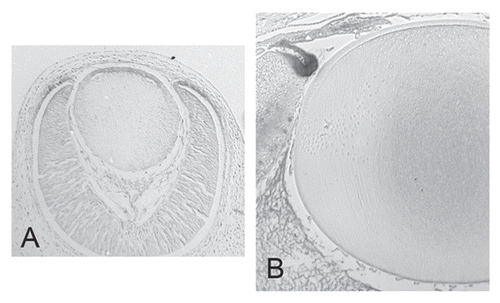
Figure 5 PCP proteins in the lens. (A–D) Disruption of lens fiber orientation in core PCP protein mutant. (A and C) Sagittal histological sections of P0 mouse eyes stained with hematoxylin and eosin. The Looptail homozygous (Lp/Lp) lens clearly has abnormal dimensions, being flatter and wider than the lens of the wild-type littermate (+/+). (B and D) After phalloidin staining, confocal microscopy from the anterior polar aspect shows that fibers in the wild-type lens exhibit regular parallel alignment and orientation so that they form a distinctive Y-shaped suture. In contrast, fibers in the Lp/Lp lens are not regularly aligned and lack the orientation required to form a normal Y-shaped suture. (E and F) PCP proteins are partitioned to cellular domains. At apical tips of OCF, Fz6 and Vangl2 are predominantly associated with the short side of each cell proximal to anterior pole. In contrast, Pk1 is predominantly localized to the long sides. Dvl2/Dvl3 tend to be localized to all sides of each cell, with some cells showing a little more intense reactivity associated with the short side proximal to anterior pole. In (F), β-catenin (light gray) demarcates cell margins and co-localizes with Pk1, Dvl2 and Dvl3. Inv localizes similarly to the primary cilium/centrosome (see pericentrin localization in ). Arrow points to anterior pole. Adapted from Sugiyama et al.Citation2 See the online version of this article to view color figures.
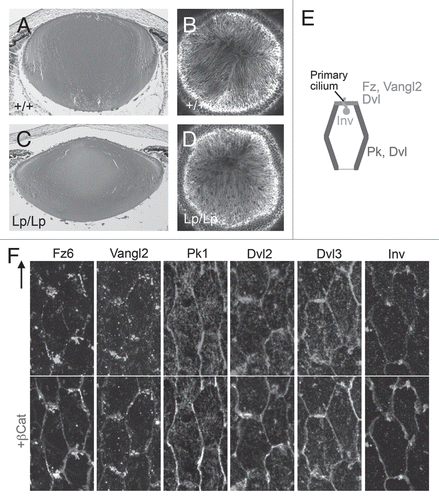
Figure 6 Primary cilium/centrosome polarization in lens cells. Pericentrin (white) immunoreactivity localizes the primary cilium/centrosome, and β-catenin (light gray) localization demarcates cell margins in rat lens whole mounts. In TZ, OCF (and to some extent in CE), the primary cilium/centrosome is clearly associated with the cell margin proximal to the anterior pole. Arrow points to anterior pole. Diagrammatic summary with arrows represents regions of lens exhibiting PCP. Note that the apical tips of OCF and TZ, which show PCP, are adherant to the apical surfaces of GZ, which does not show PCP. Adapted from Sugiyama et al.Citation2 See the online version of this article to view color figures.
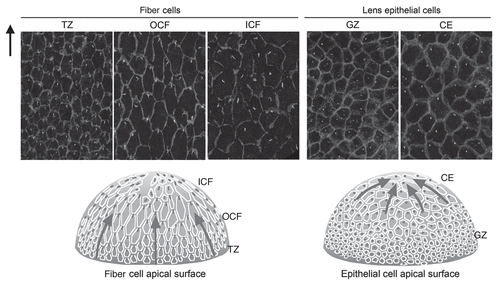
Figure 7 Overexpression of Sfrp2 disrupts global alignment of lens fibers. (A) Sagittal sections of the P3 mouse eye show that overexpression of Sfrp2 (Sfrp2+) in lens alters its shape and leads to a flatter anterior surface and a pointed posterior pole. Elongating fibers do not develop convex curvature as seen in wild-type (WT) lenses, but rather show a concave configuration. With this abnormal curvature and orientation, sutures do not form at poles. (B) Confocal microscopy of lectin-stained lenses viewed in horizontal cross section though the anterior pole show that the distinctive Y-shaped suture present in the WT lens is absent from the Sfrp2+ littermate. (C and D) Histological cross sections of lenses stained for β-catenin show that compared with WT lens, fiber orientation in the Sfrp2+ lens appears to be random. However, groups of fibers exhibit some local order and when groups with differing orientations meet, suture-like arrangements (arrows in C) or boundaries (arrows in D) are evident. (E) Pericentrin (white) immunoreactivity localizes the primary cilium/centrosome and β-catenin (light gray); localization demarcates cell margins in lens whole mounts from WT and Sfrp2+ littermates. Note that in virtually all cells in both WT and Sfrp2+ lenses, the primary cilium/centrosome is clearly polarized to one side of the cell. This is consistent with PCP still operating locally within individual groups of fibers in Sfrp2+ lenses, but in the absence of a putative GPS, there is no coordination of polarized behavior between the different groups. Adapted from Sugiyama et al.Citation2 See the online version of this article to view color figures.
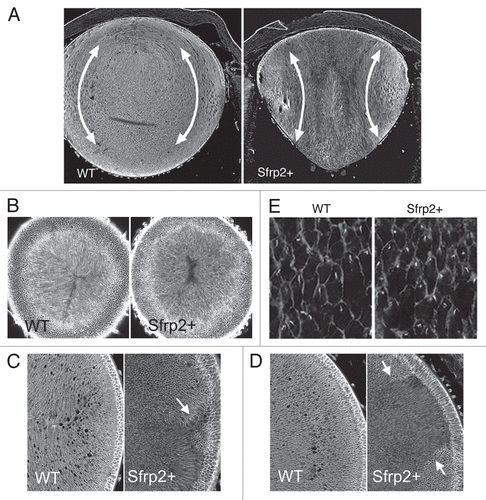
Figure 8 Proposed mechanism regulating global polarization of fibers. (A and B) In wild type (WT), lens fibers undergo directed migration in response to a GPS present at highest concentration at the anterior pole. This results in global alignment/orientation toward the pole. (C and D) Overexpressed Sfrp2 (Sfrp2+) associates with Fz receptors on fibers preventing their activation and blocking response to the putative Wnt guidance signal (C), or Sfrp2+ sequesters Wnts and disrupts its normal distribution gradient (D). In either case, while some local alignment/orientation still occurs, global alignment/orientation is abrogated.
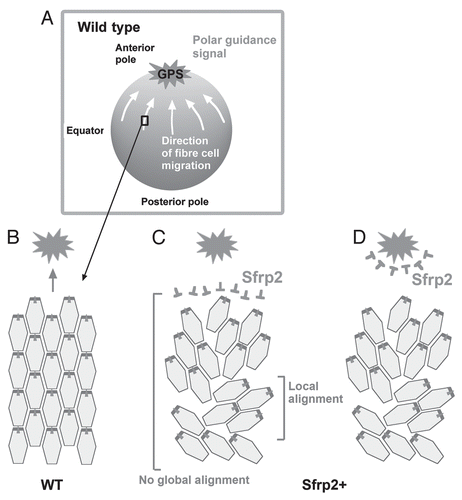
Acknowledgments
The authors would like to acknowledge the support of the National Institutes of Health (R01 EY0-3177), National Health and Medical Research Council (NHMRC), the Sydney Foundation for Medical Research and Ophthalmic Research Institute of Australia.
References
- Lovicu FJ, McAvoy JW. Growth factor regulation of lens development. Dev Biol 2005; 280:1 - 14; PMID: 15766743; http://dx.doi.org/10.1016/j.ydbio.2005.01.020
- Sugiyama Y, Stump RJ, Nguyen A, Wen L, Chen Y, Wang Y, et al. Secreted frizzled-related protein disrupts PCP in eye lens fiber cells that have polarized primary cilia. Dev Biol 2010; 338:193 - 201; PMID: 19968984; http://dx.doi.org/10.1016/j.ydbio.2009.11.033
- Chen Y, Stump RJ, Lovicu FJ, Shimono A, McAvoy JW. Wnt signaling is required for organization of the lens fiber cell cytoskeleton and development of lens three-dimensional architecture. Dev Biol 2008; 324:161 - 176; PMID: 18824165; http://dx.doi.org/10.1016/j.ydbio.2008.09.002
- McAvoy JW. Cell division, cell elongation and distribution of alpha-, beta- and gamma-crystallins in the rat lens. J Embryol Exp Morphol 1978; 44:149 - 165; PMID: 650132
- McAvoy JW. Cell division, cell elongation and the co-ordination of crystallin gene expression during lens morphogenesis in the rat. J Embryol Exp Morphol 1978; 45:271 - 281; PMID: 353215
- Kallifatidis G, Boros J, Shin EH, McAvoy JW, Lovicu FJ. The fate of dividing cells during lens morphogenesis, differentiation and growth. Exp Eye Res 2011; 92:502 - 511; PMID: 21440542; http://dx.doi.org/10.1016/j.exer.2011.03.012
- Sugiyama Y, Akimoto K, Robinson ML, Ohno S, Quinlan RA. A cell polarity protein aPKCλ is required for eye lens formation and growth. Dev Biol 2009; 336:246 - 256; PMID: 19835853; http://dx.doi.org/10.1016/j.ydbio.2009.10.010
- Song S, Landsbury A, Dahm R, Liu Y, Zhang Q, Quinlan RA. Functions of the intermediate filament cytoskeleton in the eye lens. J Clin Invest 2009; 119:1837 - 1848; PMID: 19587458; http://dx.doi.org/10.1172/JCI38277
- Jiang JX. Gap junctions or hemichannel-dependent and independent roles of connexins in cataractogenesis and lens development. Curr Mol Med 2010; 10:851 - 863; PMID: 21091421; http://dx.doi.org/10.2174/156652410793937750
- Bassnett S. On the mechanism of organelle degradation in the vertebrate lens. Exp Eye Res 2009; 88:133 - 139; PMID: 18840431; http://dx.doi.org/10.1016/j.exer.2008.08.017
- Kuszak JR, Zoltoski RK, Tiedemann CE. Development of lens sutures. Int J Dev Biol 2004; 48:889 - 902; PMID: 15558480; http://dx.doi.org/10.1387/ijdb.041880jk
- Chamberlain CG, McAvoy JW. Induction of lens fiber differentiation by acidic and basic fibroblast growth factor (FGF). Growth Factors 1989; 1:125 - 134; PMID: 2624777; http://dx.doi.org/10.3109/08977198909029122
- Chamberlain CG, McAvoy JW. Evidence that fibroblast growth factor promotes lens fiber differentiation. Curr Eye Res 1987; 6:1165 - 1169; PMID: 3665571; http://dx.doi.org/10.3109/02713688709034890
- McAvoy JW, Chamberlain CG. Fibroblast growth factor (FGF) induces different responses in lens epithelial cells depending on its concentration. Development 1989; 107:221 - 228; PMID: 2632221
- Lovicu FJ, Overbeek PA. Overlapping effects of different members of the FGF family on lens fiber differentiation in transgenic mice. Development 1988; 125:3365 - 3377; PMID: 9693140
- Robinson ML. An essential role for FGF receptor signaling in lens development. Semin Cell Dev Biol 2006; 17:726 - 440; PMID: 17116415; http://dx.doi.org/10.1016/j.semcdb.2006.10.002
- Zhao H, Yang T, Madakashira BP, Thiels CA, Bechtle CA, Garcia CM, et al. Fibroblast growth factor receptor signaling is essential for lens fiber cell differentiation. Dev Biol 2008; 318:276 - 288; PMID: 18455718; http://dx.doi.org/10.1016/j.ydbio.2008.03.028
- Schulz MW, Chamberlain CG, de Iongh RU, McAvoy JW. Acidic and basic FGF in ocular media and lens: implications for lens polarity and growth patterns. Development 1993; 118:117 - 126; PMID: 7690700
- Gao C, Chen YG. Dishevelled: The hub of Wnt signaling. Cell Signal 2010; 22:717 - 727; PMID: 20006983; http://dx.doi.org/10.1016/j.cellsig.2009.11.021
- Wu J, Mlodzik M. A quest for the mechanism regulating global planar cell polarity of tissues. Trends Cell Biol 2009; 19:295 - 305; PMID: 19560358; http://dx.doi.org/10.1016/j.tcb.2009.04.003
- Goodrich LV, Strutt D. Principles of planar polarity in animal development. Development 2011; 138:1877 - 1892; PMID: 21521735; http://dx.doi.org/10.1242/dev.054080
- Qian D, Jones C, Rzadzinska A, Mark S, Zhang X, Steel KP, et al. Wnt5a functions in planar cell polarity regulation in mice. Dev Biol 2007; 306:121 - 133; PMID: 17433286; http://dx.doi.org/10.1016/j.ydbio.2007.03.011
- Gao B, Song H, Bishop K, Elliot G, Garrett L, English MA, et al. Wnt signaling gradients establish planar cell polarity by inducing Vangl2 phosphorylation through Ror2. Dev Cell 2011; 20:163 - 176; PMID: 21316585; http://dx.doi.org/10.1016/j.devcel.2011.01.001
- Mii Y, Taira M. Secreted Frizzled-related proteins enhance the diffusion of Wnt ligands and expand their signalling range. Development 2009; 136:4083 - 4088; PMID: 19906850; http://dx.doi.org/10.1242/dev.032524
- Stump RJ, Ang S, Chen Y, Von Bahr T, Lovicu FJ, Pinson K, et al. A role for Wnt/beta-catenin signaling in lens epithelial differentiation. Dev Biol 2003; 259:48 - 61; PMID: 12812787; http://dx.doi.org/10.1016/S0012-1606(03)00179-9
- Lyu J, Joo CK. Wnt signaling enhances FGF2-triggered lens fiber cell differentiation. Development 2004; 131:1813 - 1824; PMID: 15084465; http://dx.doi.org/10.1242/dev.01060
- Ang SJ, Stump RJ, Lovicu FJ, McAvoy JW. Spatial and temporal expression of Wnt and Dickkopf genes during murine lens development. Gene Expr Patterns 2004; 4:289 - 295; PMID: 15053977; http://dx.doi.org/10.1016/j.modgep.2003.11.002
- Chen Y, Stump RJ, Lovicu FJ, McAvoy JW. Expression of Frizzleds and secreted frizzled-related proteins (Sfrps) during mammalian lens development. Int J Dev Biol 2004; 48:867 - 877; PMID: 15558478; http://dx.doi.org/10.1387/ijdb.041882yc
- Chen Y, Stump RJ, Lovicu FJ, McAvoy JW. A role for Wnt/planar cell polarity signaling during lens fiber cell differentiation?. Semin Cell Dev Biol 2006; 17:712 - 725; PMID: 17210263; http://dx.doi.org/10.1016/j.semcdb.2006.11.005
- Miller LA, Smith AN, Taketo MM, Lang RA. Optic cup and facial patterning defects in ocular ectoderm beta-catenin gain-of-function mice. BMC Dev Biol 2006; 6:14; PMID: 16539717; http://dx.doi.org/10.1186/1471-213X-6-14
- Kreslova J, Machon O, Ruzickova J, Lachova J, Wawrousek EF, Kemler R, et al. Abnormal lens morphogenesis and ectopic lens formation in the absence of beta-catenin function. Genesis 2007; 45:157 - 168; PMID: 17410548; http://dx.doi.org/10.1002/dvg.20277
- Liu H, Thurig S, Mohamed O, Dufort D, Wallace VA. Mapping canonical Wnt signaling in the developing and adult retina. Invest Ophthalmol Vis Sci 2006; 47:5088 - 5097; PMID: 17065530; http://dx.doi.org/10.1167/iovs.06-0403
- Liu H, Mohamed O, Dufort D, Wallace VA. Characterization of Wnt signaling components and activation of the Wnt canonical pathway in the murine retina. Dev Dyn 2003; 227:323 - 334; PMID: 12815618; http://dx.doi.org/10.1002/dvdy.10315
- Smith AN, Miller LA, Song N, Taketo MM, Lang RA. The duality of beta-catenin function: a requirement in lens morphogenesis and signaling suppression of lens fate in periocular ectoderm. Dev Biol 2005; 285:477 - 489; PMID: 16102745; http://dx.doi.org/10.1016/j.ydbio.2005.07.019
- Wansleeben C, Meijlink F. The planar cell polarity pathway in vertebrate development. Dev Dyn 2011; 240:616 - 626; PMID: 21305650; http://dx.doi.org/10.1002/dvdy.22564
- Lawrence PA, Struhl G, Casal J. Planar cell polarity: one or two pathways?. Nat Rev Genet 2007; 8:555 - 563; PMID: 17563758; http://dx.doi.org/10.1038/nrg2125
- Seifert JR, Mlodzik M. Frizzled/PCP signaling: a conserved mechanism regulating cell polarity and directed motility. Nat Rev Genet 2007; 8:126 - 138; PMID: 17230199; http://dx.doi.org/10.1038/nrg2042
- Strutt D. The planar polarity pathway. Curr Biol 2008; 18:898 - 902; PMID: 18957230; http://dx.doi.org/10.1016/j.cub.2008.07.055
- Wang Y, Nathans J. Tissue/planar cell polarity in vertebrates: new insights and new questions. Development 2007; 134:647 - 658; PMID: 17259302; http://dx.doi.org/10.1242/dev.02772
- Corbit KC, Shyer AE, Dowdle WE, Gaulden J, Singla V, Chen MH, et al. Kif3a constrains beta-catenin-dependent Wnt signaling through dual ciliary and non-ciliary mechanisms. Nat Cell Biol 2008; 10:70 - 76; PMID: 18084282; http://dx.doi.org/10.1038/ncb1670
- Jones C, Chen P. Primary cilia in planar cell polarity regulation of the inner ear. Curr Top Dev Biol 2008; 85:197 - 224; PMID: 19147007; http://dx.doi.org/10.1016/S0070-2153(08)00808-9
- Sugiyama Y, McAvoy JW. Chen P, Turksen K. Analysis of PCP defects in mammalian eye lens. Planar Cell Polarity Signalling: Methods and Protocols New York Humana Press In Press
- Axelrod JD. Basal bodies, kinocilia and planar cell polarity. Nat Genet 2008; 40:10 - 11; PMID: 18163128; http://dx.doi.org/10.1038/ng0108-10
- Park TJ, Haigo SL, Wallingford JB. Ciliogenesis defects in embryos lacking inturned or fuzzy function are associated with failure of planar cell polarity and Hedgehog signaling. Nat Genet 2006; 38:303 - 311; PMID: 16493421; http://dx.doi.org/10.1038/ng1753
- Ross AJ, May-Simera H, Eichers ER, Kai M, Hill J, Jagger DJ, et al. Disruption of Bardet-Biedl syndrome ciliary proteins perturbs planar cell polarity in vertebrates. Nat Genet 2005; 37:1135 - 1140; PMID: 16170314; http://dx.doi.org/10.1038/ng1644
- Simons M, Walz G. Polycystic kidney disease: cell division without a c(l)ue?. Kidney Int 2006; 70:854 - 864; PMID: 16816842; http://dx.doi.org/10.1038/sj.ki.5001534
- Singla V, Reiter JF. The primary cilium as the cell's antenna: signaling at a sensory organelle. Science 2006; 313:629 - 633; PMID: 16888132; http://dx.doi.org/10.1126/science.1124534
- Bovolenta P, Esteve P, Ruiz JM, Cisneros E, Lopez-Rios J. Beyond Wnt inhibition: new functions of secreted Frizzled-related proteins in development and disease. J Cell Sci 2008; 121:737 - 746; PMID: 18322270; http://dx.doi.org/10.1242/jcs.026096
- Kawano Y, Kypta R. Secreted antagonists of the Wnt signalling pathway. J Cell Sci 2003; 116:2627 - 2634; PMID: 12775774; http://dx.doi.org/10.1242/jcs.00623
- Wang Y, Badea T, Nathans J. Order from disorder: Self-organization in mammalian hair patterning. Proc Natl Acad Sci USA 2006; 103:19800 - 19805; PMID: 17172440; http://dx.doi.org/10.1073/pnas.0609712104
- Wang Y, Nathans J. Tissue/planar cell polarity in vertebrates: new insights and new questions. Development 2007; 134:647 - 658; PMID: 17259302; http://dx.doi.org/10.1242/dev.02772
- Grove M, Demyanenko G, Echarri A, Zipfel PA, Quiroz ME, Rodriguiz RM, et al. ABI2-deficient mice exhibit defective cell migration, aberrant dendritic spine morphogenesis and deficits in learning and memory. Mol Cell Biol 2004; 24:10905 - 10922; PMID: 15572692; http://dx.doi.org/10.1128/MCB.24.24.10905-22.2004
- Rivera C, Yamben IF, Shatadal S, Waldof M, Robinson ML, Griep AE. Cell-autonomous requirements for Dlg-1 for lens epithelial cell structure and fiber cell morphogenesis. Dev Dyn 2009; 238:2292 - 2308; PMID: 19623611; http://dx.doi.org/10.1002/dvdy.22036
- Bellaïche Y, Radovic A, Woods DF, Hough CD, Parmentier ML, O'Kane CJ, et al. The Partner of Inscuteable/Discs-large complex is required to establish planar polarity during asymmetric cell division in Drosophila. Cell 2001; 106:355 - 366; PMID: 11509184; http://dx.doi.org/10.1016/S0092-8674(01)00444-5
- Findl O, Buehl W, Bauer P, Sycha T. Interventions for preventing posterior capsule opacification. Cochrane Database Syst Rev 2010; 2:3738; PMID: 20166069