Abstract
We investigated the potential of salicylic acid (SA) in alleviating the adverse effects of heat stress on photosynthesis in wheat (Triticum aestivum L.) cv WH 711. Activity of ribulose 1,5-bisphosphate carboxylase (Rubisco), photosynthetic-nitrogen use efficiency (NUE), and net photosynthesis decreased in plants subjected to heat stress (40°C for 6 h), but proline metabolism increased. SA treatment (0.5 mM) alleviated heat stress by increasing proline production through the increase in γ-glutamyl kinase (GK) and decrease in proline oxidase (PROX) activity, resulting in promotion of osmotic potential and water potential necessary for maintaining photosynthetic activity. Together with this, SA treatment restricted the ethylene formation in heat-stressed plants to optimal range by inhibiting activity of 1-aminocyclopropane carboxylic acid (ACC) synthase (ACS). This resulted in improved proline metabolism, N assimilation and photosynthesis. The results suggest that SA interacts with proline metabolism and ethylene formation to alleviate the adverse effects of heat stress on photosynthesis in wheat.
Introduction
Heat stress has been recognized as a serious threat to crop production worldwide.Citation1 Intergovernmental panel on climatic change (IPCC) reported an expected global mean temperature increase of 0.3°C per decadeCitation2 and may reach to approximately 1 and 3°C above the present value by 2025 and 2100, respectively. Heat stress induces generation of excessive reactive oxygen species (ROS) resulting in cellular injuries and even collapse of cellular organization.Citation3
The inhibition of photosynthesis by heat stress has been attributed to the impairment of structural organization and decrease of photosystem II (PSII) activity.Citation4 Heat stress damages the oxygen-evolving complex of PSII and impairs electron transfer within the PSII reaction centers.Citation5 Also, the photosynthetic inhibition in plants under heat stress results from the inhibition of the activation of ribulose 1,5-bisphosphate carboxylase (Rubisco),Citation6 Rubisco activase activity,Citation7 and the Calvin cycle reactions together with the decrease in stomatal conductanceCitation8 and intercellular CO2 concentration.Citation9 Xu and ZhouCitation10 have shown that inhibition of photosynthesis under heat stress was associated with reduced nitrogen (N) assimilation and decreased availability of N to photosynthetic apparatus, which adversely affects Rubisco protein and activity.
The accumulation of proline is one of the adaptive mechanisms that plants operate for survival under stress.Citation11,Citation12 It regulates abiotic stress by detoxifying excess ROS, cellular osmotic adjustment, protection of biological membranes, and stabilization of enzymes/proteins.Citation11 Under environmental stress, proline acts as an antioxidant by utilizing NADPH for the reduction of glutamate to P5C and further from P5C to proline and generates NADP+, which is otherwise prevented due to reduced rate of the Calvin cycle resulting in the production of singlet oxygen and accumulation of ROS.Citation13 This generated NADP+ is used as an electron acceptor and for the restoration of the Calvin cycle under stress conditions.Citation14 In plants, proline biosynthesis from glutamate appears to be the predominant pathway under stress conditions.Citation15 Proline metabolism involves enzymes, γ-glutamyl kinase (GK), and γ-glutamyl phosphate reductase, regarded as enzyme complex P5C synthetase. The enzyme GK is considered as the key enzyme in proline metabolism. Moreover, proline oxidase (PROX) also influences proline accumulation as it degrades proline and provides glutamate to enter the pathway. The tolerance of plants and protection of photosynthesis against heat stress could be enhanced by adopting strategies to increase proline metabolism and investment of greater N to photosynthesis by increasing photosynthetic-nitrogen use efficiency (NUE).
Salicylic acid (SA), a common phenolic compound functions as a plant growth regulator and promotes photosynthesis under heat stress by influencing various physiological processes and biochemical reactions.Citation16,Citation17 Studies have shown that SA alleviated temperature,Citation9 salinity,Citation17,Citation18 and drought stress.Citation19 SA applied through rooting medium improved photosynthetic capacity of spring wheatCitation20 and foliar spray of SA alleviated decreases in photosynthesis under salt stress by increasing N assimilation and antioxidant metabolism in mungbean.Citation17,Citation21 SA may also influence photosynthesis by interacting with ethylene signaling. The inhibition of ethylene synthesis under heat stress by SA may result in increasing plants’ response to ethylene and promoting proline metabolism, photosynthetic-NUE, and photosynthesis.
The objective of the research was to assess the effect of SA applied exogenously in protection of photosynthesis of wheat (Triticum aestivum L. cv WH 711) plants subjected to heat stress. The study was undertaken to test the hypothesis that SA protects photosynthesis through increase in proline metabolism, photosynthetic-NUE, and modulating ethylene formation under heat stress.
Results
SA induces γ-glutamyl kinase and inhibits proline oxidase for proline accumulation under heat stress
In order to assess the role of proline in heat tolerance, we studied proline metabolizing enzymes GK and PROX under no stress and heat stress and studied how much they were induced by 0.5 mM SA treatment. Proline accumulation increased significantly on application of SA as well as with heat stress treatment. Heat stress induced proline biosynthesis and increased proline content by 84.7% in comparison to control. Proline accumulation under heat stress was further increased with SA application. Maximum proline accumulation of 168.6% resulted from 0.5 mM SA under heat stress compared with control. Application of 0.5 mM SA to no-stress plants increased proline accumulation by 76.5% in comparison to control ().
Figure 1. Proline content, γ-glutamyl kinase activity, and proline oxidase activity in wheat (Triticum aestivum L.) cv WH 711 at 30 DAS. Plants were grown with/without heat stress and treated with foliar 0.5 mM SA at 15 DAS. Data are presented as treatments mean ± SE (n = 4). Data followed by same letter are not significantly different by LSD test at p < 0.05. FW; fresh weight.
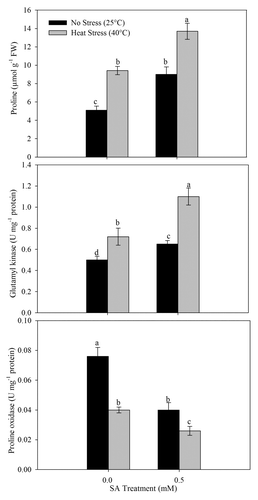
Activity of GK increased in heat-stressed plants and also with SA plus heat stress treatments. Application of 0.5 mM SA increased 120.0% GK activity of heat-stressed plants compared with control (). On the other hand, activity of PROX reduced in no-stress and heat-stressed plants with SA treatment. Application of 0.5 mM SA reduced PROX activity by 65.8% in stressed plants, while a reduction of 47.4% occurred in heat-stressed plants compared with control ().
SA reduces heat stress-induced oxidative stress
For determining the influence of SA in alleviating heat stress-induced oxidative stress, we analyzed the contents of TBARS and H2O2 and measured chlorophyll fluorescence parameters after application of SA. Application of SA proved effective in lowering oxidative stress under heat stress. The content of TBARS and H2O2 were reduced by 28.9% and 50.9% with 0.5 mM SA treatment under heat stress compared with control ().
Figure 2. Content of TBARS and H2O2 in wheat (Triticum aestivum L.) cv WH 711 at 30 DAS. Plants were grown with/without heat stress and treated with foliar 0.5 mM SA at 15 DAS. Data are presented as treatments mean ± SE (n = 4). Data followed by same letter are not significantly different by LSD test at p < 0.05. FW; fresh weight, TBARS; thiobarbituric acid reactive substances.
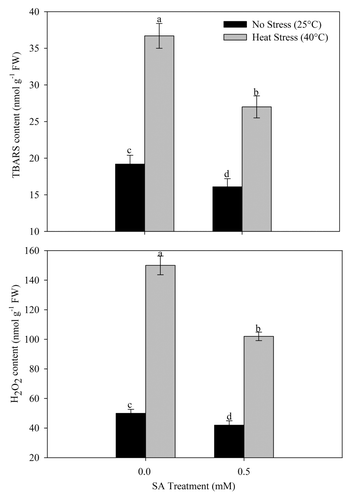
Measurement of chlorophyll fluorescence was under taken in the terms of initial chlorophyll fluorescence (Fo), maximal fluorescence (Fm), variable fluorescence (Fv), and quantum yield efficiency of photosystem II (Fv/Fm). Application of SA proved most effective in lowering oxidative stress under heat stress and increased quantum yield efficiency of photosystem II compared with heat-stressed plants ().
Table 1. Initial chlorophyll fluorescence (F0), maximal fluorescence (Fm), variable fluorescence (Fv), and quantum yield efficiency of photosystem II (Fv/Fm) of wheat (Triticum aestivum) cv WH 711 at 30 d after sowing (DAS). Plants were grown with/without heat stress and treated with foliar 0.5 mM SA. Data are presented as treatments mean ± SE (n = 4)
SA increases photosynthetic-NUE and N metabolism under heat stress
The application of 0.5 mM SA increased NR activity, N content, and photosynthetic-NUE of plants grown under no stress and heat stress over the control. In contrast, heat stress inhibited N content, NR activity, and photosynthetic-NUE by 33.3%, 23.8%, and 44.2%, respectively, compared with the control. The follow-up treatment of heat stress plants with 0.5 mM SA reduced the adverse effects of heat stress and the decrease in N content, NR activity, and photosynthetic-NUE was limited to 7.4%, 3.2%, and 6.7%, respectively, compared with the control ().
Figure 3. Leaf N content, NR activity, and photosynthetic-NUE in wheat (Triticum aestivum L.) cv WH 711 at 30 DAS. Plants were grown with/without heat stress and treated with foliar 0.5 mM SA at 15 DAS. Data are presented as treatments mean ± SE (n = 4). Data followed by same letter are not significantly different by LSD test at p < 0.05. FW; fresh weight, DW; dry weight, NUE; nitrogen use efficiency.
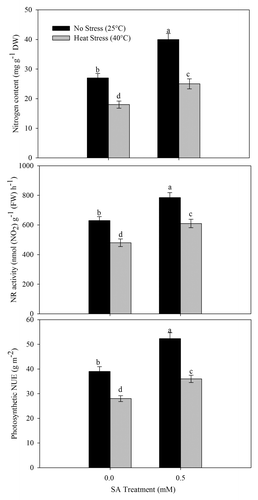
Influence of SA on leaf osmotic potential and water potential
The treatment of 0.5 mM SA alone resulted in increase of osmotic potential by 57.6% and water potential by 44.6% compared with the control (). The plants subjected to heat stress showed reduction of 32.1% in osmotic potential and 44.2% in water potential compared with the control (). Application of SA on heat-stressed plants lowered the reduction in osmotic potential and water potential caused by heat stress.
Figure 4. Leaf osmotic potential and water potential in wheat (Triticum aestivum L.) cv WH 711 at 30 DAS. Plants were grown with/without heat stress and treated with foliar 0.5 mM SA at 15 DAS. Data are presented as treatments mean ± SE (n = 4). Data followed by same letter are not significantly different by LSD test at p < 0.05.
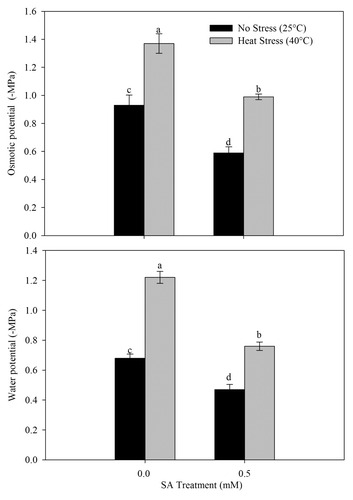
SA alleviates heat stress effects on photosynthesis, SPAD chlorophyll, rubisco activity, and water-use efficiency
Application of 0.5 mM SA to no-stress or heat-stressed plants increased photosynthetic characteristics significantly. Heat stress significantly reduced net photosynthesis, stomatal conductance, and intercellular CO2 concentration by 52.2%, 16.3%, and 41.0% compared with the control. However, SA treatment to heat-stressed plants reduced the negative effects on photosynthetic characteristics, and SA application limited the decreases in the above characteristics to 7.2%, 5.5% and 4.6%, respectively, compared with the control ().
Figure 5. Net photosynthesis, stomatal conductance, and intercellular CO2 concentration in wheat (Triticum aestivum L.) cv WH 711 at 30 DAS. Plants were grown with/without heat stress and treated with foliar 0.5 mM SA at 15 DAS. Data are presented as treatments mean ± SE (n = 4). Data followed by same letter are not significantly different by LSD test at p < 0.05.
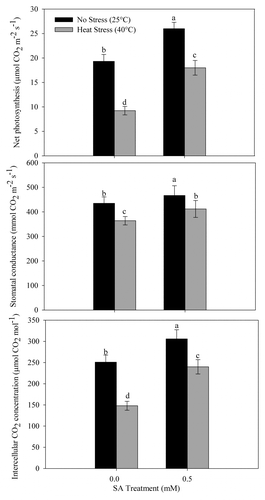
SA at 0.5 mM increased chlorophyll, Rubisco activity, and water-use efficiency (WUE) in no-stress plants. However, the decreases in these characteristics in response to heat stress were reduced by 0.5 mM SA. Heat stress reduced chlorophyll content, Rubisco activity, and WUE by 41.4%, 54.2%, and 42.8%, respectively, compared with the control. Application of 0.5 mM SA reduced the loss of chlorophyll, Rubisco activity, and WUE and showed maximum protection from heat stress. The decrease in chlorophyll content, Rubisco activity, and WUE due to heat stress treatment was restricted to 13.8%, 16.7%, and 9.2%, respectively, under heat stress compared with the control ().
Figure 6. SPAD chlorophyll, rubisco activity and water use efficiency of wheat (Triticum aestivum L.) cv WH 711 at 30 DAS. Plants were grown with/without heat stress and treated with foliar 0.5 mM SA at 15 DAS. Data are presented as treatments mean ± SE (n = 4). Data followed by same letter are not significantly different by LSD test at p < 0.05.
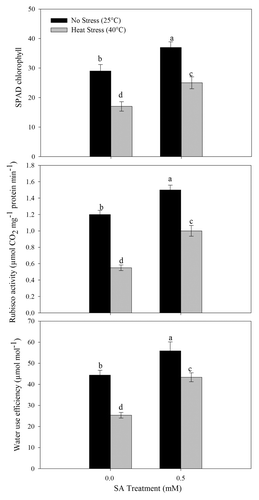
SA inhibits ACS activity and ethylene formation
Plants subjected to heat stress enhanced ACS activity by about 2 times and ethylene by about 1.5 times compared with the control. Treatment of SA inhibited ACS activity and ethylene formation in plants grown under no stress and heat stress conditions. SA treatment given to heat-stressed plants lowered ethylene formation through the decrease in ACS activity by 34.3% compared with the control ().
Figure 7. Leaf ACS activity and ethylene in wheat (Triticum aestivum L.) cv WH 711 at 30 DAS. Plants were grown with/without heat stress and treated with foliar 0.5 mM SA at 15 DAS. Data are presented as treatments mean ± SE (n = 4). Data followed by same letter are not significantly different by LSD test at p < 0.05. ACS; 1-aminocyclopropane carboxylic acid synthase.
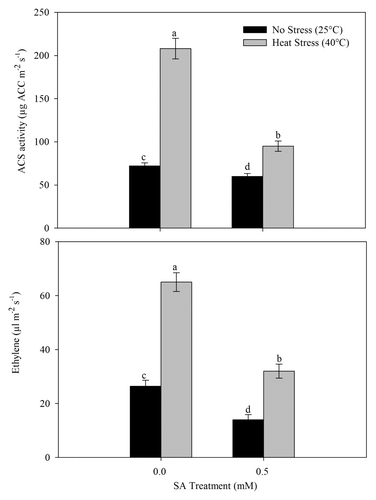
Discussion
Phytohormones play central role in modifying the plants’ ability to adapt under changing environments by mediating several physiological processes, including induction of osmolyte (proline) synthesis, utilization of nutrients, and increasing source strength. The role of SA in regulation of physiological process and plant development has been reported.Citation17,Citation21-Citation23 The reported research was undertaken to gain insight into the SA-induced mechanisms for protection of photosynthesis against heat stress. In this study, it was observed that SA application promoted photosynthesis under no stress condition, and alleviated the adverse effect of heat stress. The protection of photosynthesis under heat stress was associated with the integrated physiological and biochemical mechanisms induced by 0.5 mM SA treatment, such as increased synthesis of proline and N assimilation and suppression of stress ethylene formation. The increased proline accumulation after SA application resulted from induced γ-glutamyl kinase activity and inhibited proline oxidase activity. SA application has been shown to influence photosynthetic capacity of crop plants in a dose-dependent manner. Lower concentration of SA improves photosynthetic characteristics,Citation17,Citation20,Citation21 while higher SA concentration has been found to inhibit photosynthesis by inhibiting the synthesis of large and small subunits of Rubisco,Citation22 disintegration of plastid structure,Citation23 and increased accumulation of NADPH by limiting the Calvin cycle.Citation24
It has been reported that γ-glutamyl kinase and proline oxidase play important roles in controlling the level of proline and environmental stress in plants.Citation12,Citation25 Study on salt stress has shown that increased γ-glutamyl kinase activity and reduced proline oxidase activity by SA treatment leads to increased tolerance of green gramCitation26 and lentil.Citation27 In the present study, the SA-induced accumulation of proline protected photosynthetic machinery from heat stress injury both by increasing water potential through increased osmotic potential and by reducing oxidative stress induced by heat stress. The oxidative stress, measured as content of H2O2 and TBARS, and chlorophyll fluorescence in heat-stressed plants was found reduced by SA treatment. Proline has been shown to reduce oxidative stress by acting as potential oxygen radical scavenger in plants.Citation28-Citation30 The observed correlation between SA-induced changes in net photosynthesis and SA-induced changes in proline under heat stress suggests that SA application protects photosynthesis through alleviating the adverse effects of heat stress on proline accumulation (). Rasheed et al.Citation33 have shown increased heat stress tolerance in sugarcane by increased proline accumulation and restricting H2O2 generation. In contrast, Lv et al.Citation34 have shown higher ion leakage, higher ROS, and malondialdehyde levels with the increased activity of the Pro/P5C cycle and proline accumulation after the recovery of plants from heat treatment in Arabidopsis seedlings. Moreover, increase in water potential by SA treatment of heat-stressed plants helped in stabilization of Rubisco protein and the increased stomatal conductance facilitated higher rate of CO2 diffusion into intercellular spaces leading to increased photosynthesis. Baghizadeh and HajmohammadrezaeiCitation32 have shown that plants overcome the water deficiency effects via accumulation of compatible osmolytes that were influenced by SA. The favorable effect of SA in increasing photosynthesis through the increase in stomatal conductance may also be attributed to the SA-inhibited ABA content in guard cells. Rai et al.Citation31 have shown that SA reverses the ABA-mediated stomatal closure.
Figure 8. Relationship between SA-induced changes in net photosynthesis (Pn) and SA-induced changes in proline content under heat stress in wheat (Triticum aestivum L.) cv WH 711. The percent increase in values from 0.5 mM SA plus heat stress over heat stress plants (n = 4). ** significant at p < 0.01.
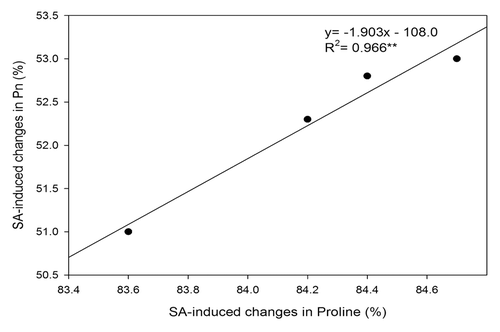
In addition, protection of photosynthesis from heat stress by 0.5 mM SA also resulted from the increased N assimilation (activity of nitrate reductase and N content) and higher allocation of N to Rubisco protein (photosynthetic-NUE). Photosynthetic-NUE is an important tool to improve the inherent variation in photosynthetic capacity, which increases if more leaf N is allocated to Rubisco. The increased N assimilation in plants receiving SA provides N backbone for chlorophyll and proline synthesis. The increased SPAD values in heat-stressed plants after SA application reflect higher chlorophyll synthesis. SPAD values have a significant association with photosynthesis and leaf N content.Citation35 Recently, Albert et al.Citation36 have suggested that proline plays a significant role in leaf N remobilization and in N-use efficiency in oilseed rape under water limited conditions. Palma et al.Citation18 have shown the contribution of SA treatment in preventing N fixation inhibition and improvement in salinity tolerance in Medicago sativa.
Moreover, SA treatment antagonistically interacted with ethylene synthesis by inhibiting ACS activity and ethylene formation in heat-stressed plants. The inhibition of photosynthetic functions by stress ethylene induced by heat stress was alleviated by 0.5 mM SA application. Thus, ethylene formation in heat-stressed plants was restricted to the optimal range after application of 0.5 mM SA. The optimal ethylene formation resulting from SA application under heat stress elicited its effects on photosynthetic functions (PSII and Rubisco activity) via its influence on N assimilation, photosynthetic-NUE, and proline synthesis.
We have shown earlier that ethylene increases photosynthesisCitation37 via increase in NUE and allocation of N to Rubisco, and also through increase in stomatal conductance in mustard.Citation38-Citation41 In contrast, Leblanc et al.Citation42 have shown that N uptake and nitrate transporter BnNrt2.1 transcript levels were markedly reduced in the presence of ethylene promoter in Brassica napus, but were increased in the presence of ethylene inhibitor. Li et al.Citation43 have observed inhibition of ACS expression in the presence of SA in Lycopersicon esculentum. Study of Alvarez et al.Citation44 also suggested that salt stress tolerance could be induced by higher level of proline accumulation and low level of ethylene in sunflower. There are reports available that show increased photosynthesis by SA treatment under cadmium,Citation45 water,Citation46 heat,Citation9 and salt stress.Citation17,Citation21 However, the study of interaction between SA and ethylene in the regulation of photosynthesis by regulating proline production and photosynthetic-NUE in wheat under heat stress has not been reported. The present study showed that SA treatment alleviated the heat stress induced decrease in photosynthesis and maintained higher PSII function and Rubisco activity, and it accelerated the recovery of photosynthesis mainly through its effects on ethylene formation. There is probably some SA-induced ethylene signaling response chain that integrated synthesis of proline and N assimilation and protected photosynthesis under heat stress. A schematic representation is shown in to connect various responses, such as proline synthesis, nitrogen metabolism, ethylene production, and photosynthesis after SA application under heat stress.
Figure 9. Schematic representation showing SA application results in protected photosynthesis under heat stress in wheat involving proline accumulation, water balance, N metabolism, and ethylene formation. ACS, 1-aminocyclopropane carboxylic acid synthase; NUE, nitrogen use efficiency; ROS, reactive oxygen species; SA, salicylic acid (dotted lines represent areas need to be investigated).
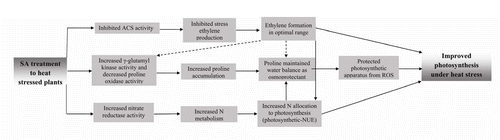
Conclusively, it may be said that 0.5 mM SA alleviated the adverse effects of heat stress on photosynthesis by mechanism of induced proline accumulation and interaction with ethylene. The increase in proline production following SA treatment under heat stress increased osmotic potential enabling the plants for higher water intake resulting in positive influence on stomatal aperture and photosynthetic machinery leading to higher efficiency of PS II and increased Rubisco activity that cumulatively resulted in increased photosynthesis under heat stress. Moreover, SA application inhibited stress ethylene formation under heat stress and optimal ethylene resulting after SA treatment favored proline production and influenced photosynthesis positively via its effect on photosynthetic machinery. The information of the interaction between SA and ethylene in eliciting responses could be exploited in future for providing tolerance to wheat crop against heat stress by modulating these plant hormones. The major regulatory points of SA and ethylene signaling pathway components can be targeted for manipulation to improve the response of crop to heat stress.
Materials and Methods
Plant material and growth conditions
Wheat (Triticum aestivum L.) cv WH 711 seeds were surface sterilized with 0.1% HgCl2 followed by repeated washings with deionized water, and were sown in 300 mL plastic pots filled with acid-washed sand. The pots were kept in the environmental growth chamber (Khera KI-261, Delhi) with day/night temperatures at 25/18 ± 3°C, 12 h photoperiod (PAR 300 µmol m−2 s−1), and relative humidity of 65 ± 5%. Two plants per pot were maintained and were saturated alternate days with 300 mL of full strength Hoagland’s nutrient solution. In the experimentation, 1 set of plants was maintained at 25°C (no stress) while another set of plants was subjected to 40°C for 6 h daily for 15 d and were then allowed to recover at 25°C (heat stress) and grown for the experimental period. SA was dissolved in absolute ethanol then added drop wise to water (ethanol/water: 1/1000 v/v) and was applied at 15 d after sowing (DAS) on the foliage of plants at the concentrations of 0.5 mM on plants subjected to no stress or heat stress with a hand sprayer. The control plants were sprayed with ethanol/water: 1/1000 v/v. A surfactant teepol (0.5%) was added with the control and SA treatments solution. The volume of the spray was 25 mL per pot. The concentration of SA was selected based on our earlier findings.Citation17,Citation21 The experiment followed a randomized complete block design and the number of replicates for each treatment was 4 (n = 4). Measurements were done at 30 DAS and care was taken to select the same age of leaves for the determinations.
Estimation of proline
Proline content was determined spectrophotometrically by adopting the ninhydrin method of Bates et al.Citation47 Fresh leaf samples (300 mg) were homogenized in 3 mL of 3% sulphosalicylic acid. The homogenate filtrate was reacted with 1 mL each of acid ninhydrin and glacial acetic acid for 1h in a test tube placed in a water bath at 100°C. The mixture was extracted with toluene and the absorbance was measured on a spectrophotometer (UV–vis L164, Elico, Hyderabad, India) at 520 nm using L-proline as a standard.
Assay of γ-glutamyl kinase activity and proline oxidase activity
To determine the activity of GK and PROX, enzyme extract was prepared by homogenizing 500 mg leaf sample in 0.1 M Tris-HCl buffer, pH 7.5, at 4°C. The homogenate was centrifuged at 30,000 × g for 30 min and pellet was collected and used as extract for assay of GK and PROX. For GK enzyme activity extract was kept in a freezer at –20°C.
Activity of GK (EC 2.7.2.11) was assayed by the method of Hayzer and LeisingerCitation48 with slight modification. The frozen sample was suspended in 10 mL of 0.1 M Tris–HCl buffer containing 1 mM 1, 4- dithiothreitol (DTT) to rupture the cell and centrifuged at 30,000 × g for 30 min. The assay mixture contained 50 mM L-glutamate, 10 mM ATP, 20 mM MgCl2, 100 mM hydroxylamine HCl and 50 mM Tris–HCl, pH 7.0 with 200 µL of desalted extract in a final volume of 500 µL. The reaction was started by the addition of enzyme extract. After 30 min of incubation at 37°C, the reaction was stopped by the addition of 1.0 mL FeCl3.3H2O (2.5%, w/v) and trichloroacetic acid (6%, w/v) in 2.5 M HCl. Protein was precipitated and removed by centrifugation at 12,000 × g (4°C) and absorbance was recorded at 540 nm. Activity of γ -glutamyl kinase was expressed in U mg−1 protein. One Unit (U) of enzyme activity is defined as µg of γ -glutamyl hydroxamate min−1 mg−1 protein.
PROX activity was determined adopting the method of Huang and CavalieriCitation49 with slight modification. The pellet was mixed with 1 mL Tricine, KOH buffer (pH 7.5) containing 6 M sucrose. This extract was used for the enzyme assay. The assay mixture contained 1.2 mL of 50 mM Tris–HCl buffer (pH 8.5), 1.2 mL of 5 mM MgCl2, 0.1 mL of 0.5 mM NADP, 0.1 mL of 1 mM KCN, 0.1 mL of 1 mM phenazine methosulfate (PSM), 0.1 mL of 0.06 mM 2, 6 dichlorophenol indophenols (DCPIP) and 0.1 mL of 0.1 M proline in a final volume of 3 mL. The increase in absorbance was recorded at 600 nm at 25°C using proline to initiate the reaction. Proline oxidase activity was expressed in U mg−1 protein. One Unit (U) of enzyme activity is defined as mM DCPIP reduced min−1 mg−1 protein.
Determination of lipid peroxidation, H2O2 content, and chlorophyll fluorescence
The level of lipid peroxidation in leaves was determined by estimating the content of thiobarbituric acid reactive substances (TBARS) as described by Dhindsa et al.Citation50 Fresh leaf tissues (500 mg) were ground in 0.25% 2-thiobarbituric acid in 10% trichloroacetic acid using mortar and pestle. After heating at 95°C for 30 min, the mixture was quickly cooled on ice bath and centrifuged at 10,000 × g for 10 min. The absorbance of the supernatant was read at 532 nm and corrected for non-specific turbidity by subtracting the absorbance of the same at 600 nm. The content of TBARS was calculated using the extinction coefficient (155 mM−1cm−1).
The content of H2O2 was determined following the method of Okuda et al.Citation51 Fresh leaf tissues (50 mg) were ground in ice cold 200 mM perchloric acid. After centrifugation at 1200 × g for 10 min, perchloric acid of the supernatant was neutralized with 4 M KOH. The insoluble potassium perchlorate was eliminated by centrifugation at 500 × g for 3 min. The reaction was started by the addition of peroxidase and the increase in absorbance was recorded at 590 nm for 3 min.
Fully expanded leaf of wheat plants were allowed to adapt under dark conditions for 30 min before chlorophyll fluorescence measurements using chlorophyll fluorometer (OS-30p, USA). Initial fluorescence (Fo) was measured with a measuring beam at a light intensity of 250 μmol m−2 s−1 for 2 s. Maximum fluorescence (Fm) was obtained by measuring chlorophyll fluorescence during a saturating light (5000 μmol m−2 s−1). Maximal PSII photochemical efficiency (Fv/Fm) and variable fluorescence (FV) were also recorded. The variable fluorescence (Fv) in PSII was calculated as Fm–Fo according to Maxwell and Johnson.Citation52
Determination of nitrate reductase activity, leaf N content, and photosynthetic-NUE
Activity of NR (EC 1.7.99.4) in leaves was measured by preparing the enzyme extract using the method of Kuo et al.Citation53 Leaf tissue (1.0 g) was frozen in liquid N2, ground to a powder with a chilled mortar and pestle, and then stored at −80°C. The powder was thawed for 10 min at 4°C and was homogenized in a blender in 250 mM Tris–HCl buffer, pH 8.5, containing 10 mM cysteine, 1 mM EDTA, 20 M FAD, 1 mM DTT, and 10% (v/v) glycerol. The homogenate was centrifuged at 10,000 × g for 30 min at 4°C. NR activity was assayed as the rate of nitrite production at 28°C adopting the procedure of Nakagawa et al.Citation54 The assay mixture contained 10 mM KNO3, 0.065 M HEPES (pH 7.0), 0.5 mM NADH in 0.04 mM phosphate buffer (pH 7.2), and enzyme in a final volume of 1.5 mL. The reaction was initiated by adding NADH. After 15 min the reaction was terminated by adding 1 mL of 1 N HCl solution containing 1% sulfanilamide followed by the addition of 1 mL of 0.02% aqueous N-1-napthylethylene-di-amine-dihydrochloride (NED). The absorbance was read at 540 nm after 10 min.
Leaf N content was determined in acid-peroxide digested material using the method of LindnerCitation55 Photosynthetic-NUE was calculated by the ratio of photosynthesis rate to N content per unit leaf area. Leaf area was measured using leaf area meter (LA 211, Systronic, New Delhi, India).
Determination of leaf water potential and osmotic potential
Leaf water potential was measured on second leaf from top (fully expanded youngest leaf) of the plant by using water potential system (Psypro, WESCOR, USA). The leaf used for water potential measurement was frozen in liquid nitrogen in sealed polythene bags, which was thawed and cell sap was extracted with the help of a disposable syringe. The extracted sap was used for the determination of osmotic potential using a vapor pressure osmometer (5520, WESCOR, USA).
Determination of photosynthetic parameters
Net photosynthesis, stomatal conductance, and intercellular CO2 concentration were measured in fully expanded uppermost leaves of plants in each treatment using infrared gas analyzer (CI-340, Photosynthesis System, Bio-Science, USA). The measurements were done between 11.00 and 12.00 h at light saturating intensity and at 370 ± 5 µmol mol−1 atmospheric CO2 concentrations. Chlorophyll was measured with the help of SPAD chlorophyll meter (SPAD 502 DL PLUS, Spectrum Technologies, USA).
Rubisco activity (EC 4.1.1.39) was determined spectrophotometrically by adopting the method of UsudaCitation56 by monitoring NADH oxidation at 30°C at 340 nm during the conversion of 3- phosphoglycerate to glycerol 3-phosphate after the addition of enzyme extract to the assay medium. For enzyme extraction, leaf tissue (1.0 g) was homogenized using a chilled mortar and pestle with ice-cold extraction buffer containing 0.25 M Tris–HCl (pH 7.8), 0.05 M MgCl2, 0.0025 M EDTA and 37.5 mg DTT. The homogenate was centrifuged at 4°C at 10,000 × g for 10 min. The resulting supernatant was used to assay the enzyme. The reaction mixture contained 100 mM Tris–HCl (pH 8.0), 40 mM NaHCO3, 10 mM MgCl2, 0.2 mM NADH, 4 mM ATP, 5 mM DTT, 1 U of glyceraldehydes 3-phosphodehydrogenase and 1 U of 3-phosphoglycerate kinase and 0.2 mM ribulose 1,5-bisphosphate (RuBP). Protein was estimated according to BradfordCitation57 using bovine serum albumin as standard. Water-use efficiency was calculated as the ratio of photosynthesis to stomatal conductance to avoid effects of small differences in vapor pressure between measurements.Citation58
Measurement of ACS activity and ethylene evolution
The activity of ACS (EC 4.4.1.14) was measured by adopting the methods of Avni et al.Citation59 and Woeste et al.Citation60 Leaf tissue (5.0 g) was ground in 100 mM HEPES buffer (pH 8.0) containing 4 mM dithiothreitol (DTT), 2.5 mM pyridoxal phosphate, and 25% polyvinylpyrrolidone (PVP). The homogenized preparation was centrifuged at 12,000 × g for 15 min. One mL of the supernatant was placed in a 30 mL tube and 0.1 mL of 5 mM S-adenosyl methionine (AdoMet) was added and incubated for 2 h at 22°C. The ACC formed was determined by its conversion to ethylene by the addition of 0.1 mL of 20 mM HgCl2 followed by the addition of 0.1 mL of a 1:1 mixture of saturated NaOH/NaCl and placed on ice for 10 min. In the control set, AdoMet was not added.
The evolution of ethylene was measured by cutting 0.5 g of leaf material into small pieces that were placed into 30 mL tubes containing moist paper to minimize evaporation from the tissue and were stoppered with secure rubber caps and placed in light for 2 h under the same condition used for plant growth. An earlier experiment showed that 2 h incubation time was adequate for ethylene detection without the interference of wound-induced ethylene. A 1 mL gas sample from the tubes was withdrawn with a hypodermic syringe and assayed on a gas chromatograph (Nucon 5700, New Delhi, India) equipped with a 1.8 min Porapack N (80–100 mesh) column, a flame ionization detector, and data station. Nitrogen was used as carrier gas. The flow rates of nitrogen, hydrogen, and oxygen were 30, 30, and 300 mL min−1, respectively. The detector was set at 150°C. Ethylene was identified based on the retention time and quantified by comparison with peaks from standard ethylene concentration.
Statistical analysis
Data were statistically analyzed using analysis of variance (ANOVA) by SPSS statistics (ver. 17.0), and presented as treatment mean ± SE (n = 4). Least significant difference (LSD) was calculated for the significant data at p < 0.05. Bars showing the same letter are not significantly different by LSD test at p < 0.05. Linear regression was performed for the determination of SA-induced changes in net photosynthesis and SA-induced changes in proline content under heat stress.
Abbreviations: | ||
ABA | = | abscisic acid |
ACS | = | 1-aminocyclopropane carboxylic acid synthase |
DAS | = | days after sowing |
GK | = | γ-glutamyl kinase |
IPCC | = | intergovernmental panel on climatic change |
N | = | nitrogen |
NADPH | = | nicotinamide adenine dinucleotide phosphate (reduced) |
NADP+ | = | nicotinamide adenine dinucleotide phosphate |
NED | = | N-1-napthylethylene-di-amine-dihydrochloride |
NR | = | nitrate reducatse |
PROX | = | proline oxidase |
PSII | = | photosystem II |
NUE | = | nitrogen use efficiency |
ROS | = | reactive oxygen species |
Rubisco | = | ribulose 1 |
5-bisphosphate carboxylase | = | RuBP |
ribulose 1 | = | 5-bisphosphate |
SA | = | Salicylic acid |
TBARS | = | thiobarbituric acid reactive substances |
WUE | = | water-use efficiency |
Acknowledgments
Authors are thankful to the University Grants Commission, Council of Scientific and Industrial Research and Department of Biotechnology, New Delhi for providing financial assistance for the research in the laboratory of N.A. Khan.
Disclosure of Potential Conflicts of Interest
No potential conflicts of interest were disclosed.
References
- Khan MIR, Asgher M, Khan NA. Rising temperature in the changing environment: A serious threat to plants. Climate Change Environ Sustain 2013; 1:25 - 36; http://dx.doi.org/10.5958/j.2320-6411.1.1.004
- Jones PD, New M, Parker DE, Martin S, Rigor IG. Surface air temperature and its changes over the past 150 years. Rev Geophys 1999; 37:173 - 99; http://dx.doi.org/10.1029/1999RG900002
- Schöffl F, Prandl R, Reindl A. Molecular responses to heat stress. In: Shinozaki K, Yamaguchi-Shinozaki K, eds. Molecular responses to cold, drought, heat and salt stress in higher plants. Austin, TX: R.G. Landes Co, 1999: 81-98
- Lichtenthaler HK, Langsdorf G, Lenk S, Buschamann C. Chlorophyll fluorescence imaging of photosynthetic activity with the flesh lamp fluorescence imaging system. Photosynthetica 2005; 43:355 - 69; http://dx.doi.org/10.1007/s11099-005-0060-8
- Kouril R, Lazár D, Ilík PP, Skotnica J, Krchnák P, Naus J. Krch a’k P. High temperature induced chlorophyll fluorescence rise in plants at 40–50 °C: experimental and theoretical approach. Photosynth Res 2004; 81:49 - 66; http://dx.doi.org/10.1023/B:PRES.0000028391.70533.eb; PMID: 16328847
- Crafts-Brandner SJ, Salvucci ME. Rubisco activase constrains the photosynthetic potential of leaves at high temperature and CO2. Proc Natl Acad Sci U S A 2000; 97:13430 - 5; http://dx.doi.org/10.1073/pnas.230451497; PMID: 11069297
- Feller U, Crafts-Brandner SJ, Salvucci ME. Moderately high temperatures inhibit ribulose-1,5-bisphosphate carboxylase/oxygenase (Rubisco) activase-mediated activation of Rubisco. Plant Physiol 1998; 116:539 - 46; http://dx.doi.org/10.1104/pp.116.2.539; PMID: 9490757
- Monson RK, Stidham MA, Williams GJ, Edwards GE, Uribe EG. Temperature Dependence of Photosynthesis in Agropyron smithii Rydb.: I. FACTORS AFFECTING NET CO(2) UPTAKE IN INTACT LEAVES AND CONTRIBUTION FROM RIBULOSE-1,5-BISPHOSPHATE CARBOXYLASE MEASURED IN VIVO AND IN VITRO. Plant Physiol 1982; 69:921 - 8; http://dx.doi.org/10.1104/pp.69.4.921; PMID: 16662320
- Wang LJ, Fan L, Loescher W, Duan W, Liu GJ, Cheng JS, Luo HB, Li SH. Salicylic acid alleviates decreases in photosynthesis under heat stress and accelerates recovery in grapevine leaves. BMC Plant Biol 2010; 10:34; http://dx.doi.org/10.1186/1471-2229-10-34; PMID: 20178597
- Xu ZZ, Zhou GS. Nitrogen metabolism and photosynthesis in Leymus chinensis in response to long-term soil drought. J Plant Growth Regul 2006; 25:252 - 66; http://dx.doi.org/10.1007/s00344-006-0043-4
- Hare PD, Cress WA, van Staden J. Dissecting the roles of compatible osmolyte accumulation during stress. Plant Cell Environ 1998; 21:535 - 53; http://dx.doi.org/10.1046/j.1365-3040.1998.00309.x
- Kavi Kishor PB, Sangam S, Amruth RN, Sri Laxmi P, Naidu KR, Rao KRSS, Reddy KJSR, Theriappan P, Sreenivasulu N. Regulation of proline biosynthesis, degradation, uptake and transport in higher plants: its implications in plant growth and abiotic stress tolerance. Curr Sci 2005; 88:424 - 38
- Chaves MM, Flexas J, Pinheiro C. Photosynthesis under drought and salt stress: regulation mechanisms from whole plant to cell. Ann Bot 2009; 103:551 - 60; http://dx.doi.org/10.1093/aob/mcn125; PMID: 18662937
- Hare PD, Cress WA. Metabolic implications of stress-induced proline accumulation in plants. Plant Growth Regul 1997; 21:79 - 102; http://dx.doi.org/10.1023/A:1005703923347
- Delauney AJ, Verma DPS. Proline biosynthesis and osmoregulation in plants. Plant J 1993; 4:215 - 23; http://dx.doi.org/10.1046/j.1365-313X.1993.04020215.x
- Raskin I. Role of Salicylic Acid in Plants. Annu Rev Plant Physiol Plant Mol Biol 1992; 43:439 - 63; http://dx.doi.org/10.1146/annurev.pp.43.060192.002255
- Nazar R, Iqbal N, Syeed S, Khan NA. Salicylic acid alleviates decreases in photosynthesis under salt stress by enhancing nitrogen and sulfur assimilation and antioxidant metabolism differentially in two mungbean cultivars. J Plant Physiol 2011; 168:807 - 15; http://dx.doi.org/10.1016/j.jplph.2010.11.001; PMID: 21112120
- Palma F, López-Gómez M, Tejera NA, Lluch C. Salicylic acid improves the salinity tolerance of Medicago sativa in symbiosis with Sinorhizobium meliloti by preventing nitrogen fixation inhibition. Plant Sci 2013; 208:75 - 82; http://dx.doi.org/10.1016/j.plantsci.2013.03.015; PMID: 23683932
- Yazdanpanah S, Baghizadeh A, Abbassi F. The interaction between drought stress and salicylic and ascorbic acids on some biochemical characteristics of Satureja hortensis.. Afr J Agr Res 2011; 6:798 - 807
- Arfan M, Athar HR, Ashraf M. Does exogenous application of salicylic acid through the rooting medium modulate growth and photosynthetic capacity in two differently adapted spring wheat cultivars under salt stress?. J Plant Physiol 2007; 164:685 - 94; http://dx.doi.org/10.1016/j.jplph.2006.05.010; PMID: 16884826
- Khan NA, Syeed S, Masood A, Nazar R, Iqbal N. Application of salicylic acid increases contents of nutrients and antioxidative metabolism in mungbean and alleviates adverse effects of salinity stress. Int J Plant Bio 2010; http://dx.doi.org/10.4081/pb.2010.e1
- Pancheva TV, Popova LP. Effect of salicylic acid on the synthesis of ribulose-1,5- biphosphate carboxylase/oxygenase in barley leaves. J Plant Physiol 1998; 152:381 - 6; http://dx.doi.org/10.1016/S0176-1617(98)80251-4
- Uzunova AN, Popova LP. Effect of salicylic acid on leaf anatomy and chloroplast ultrastructure of barley plants. Photosynthetica 2000; 38:243 - 50; http://dx.doi.org/10.1023/A:1007226116925
- Janda K, Hideg E, Szalai G, Kovács L, Janda T. Salicylic acid may indirectly influence the photosynthetic electron transport. J Plant Physiol 2012; 169:971 - 8; http://dx.doi.org/10.1016/j.jplph.2012.02.020; PMID: 22579359
- Abdul Jaleel C, Gopi R, Sankar B, Manivannan P. Kishore kumar A, Sridharan R, Panneerselvam R. Studies on germination, seedling vigour, lipid peroxidation and proline metabolism in Catharanthus roseus seedlings under salt stress. S Afr J Bot 2007; 73:190 - 5; http://dx.doi.org/10.1016/j.sajb.2006.11.001
- Misra N, Gupta AK. Effect of salt stress on proline metabolism in two high yielding genotypes of green gram. Plant Sci 2005; 169:331 - 9; http://dx.doi.org/10.1016/j.plantsci.2005.02.013
- Misra N, Saxena P. Effect of salicylic acid on proline metabolism in lentil grown under salinity stress. Plant Sci 2009; 177:181 - 9; http://dx.doi.org/10.1016/j.plantsci.2009.05.007
- Hamilton EW 3rd, Heckathorn SA. Mitochondrial adaptations to NaCl. Complex I is protected by anti-oxidants and small heat shock proteins, whereas complex II is protected by proline and betaine. Plant Physiol 2001; 126:1266 - 74; http://dx.doi.org/10.1104/pp.126.3.1266; PMID: 11457977
- Wahid A, Gelani S, Ashraf M, Foolad MR. Heat tolerance in plants: an overview. Environ Exp Bot 2007; 61:199 - 223; http://dx.doi.org/10.1016/j.envexpbot.2007.05.011
- Smirnoff N, Cumbes QJ. Hydroxyl radical scavenging activity of compatible solutes. Phytochemistry 1989; 28:1057 - 60; http://dx.doi.org/10.1016/0031-9422(89)80182-7
- Rasheed R, Wahid A, Farooq M, Hussain I, Basra SMA. Role of proline and glycinebetaine pretreatments in improving heat tolerance of sprouting sugarcane (Saccharum sp.) buds. Plant Growth Regul 2011; 65:35 - 45; http://dx.doi.org/10.1007/s10725-011-9572-3
- Lv WT, Lin B, Zhang M, Hua XJ. Proline accumulation is inhibitory to Arabidopsis seedlings during heat stress. Plant Physiol 2011; 156:1921 - 33; http://dx.doi.org/10.1104/pp.111.175810; PMID: 21670222
- Baghizadeh A, Hajmohammadrezaei M. Effect of drought stress and its interaction with ascorbate and salicylic acid on okra (Hibiscus esculents L.) germination and seedling growth. J Physiol Biochem 2011; 7:55 - 65
- Rai VK, Sharma SS, Sharma S. Reversal of ABA-induced stomatal closure by phenolic compounds. J Exp Bot 1986; 37:129 - 34; http://dx.doi.org/10.1093/jxb/37.1.129
- Araus JL, Bort J, Ceccarelli S, Grando S. Relationship between leaf structure and carbon isotope discrimination in field grown barley. Plant Physiol Biochem 1997; 35:533 - 41
- Albert B, Le Cahérec F, Niogret MF, Faes P, Avice JC, Leport L, Bouchereau A. Nitrogen availability impacts oilseed rape (Brassica napus L.) plant water status and proline production efficiency under water-limited conditions. Planta 2012; 236:659 - 76; http://dx.doi.org/10.1007/s00425-012-1636-8; PMID: 22526495
- Khan NA. An evaluation of the effects of exogenous ethephon, an ethylene releasing compound, on photosynthesis of mustard (Brassica juncea) cultivars that differ in photosynthetic capacity. BMC Plant Biol 2004; 4:21; http://dx.doi.org/10.1186/1471-2229-4-21; PMID: 15625009
- Pierik R, Tholen D, Poorter H, Visser EJW, Voesenek LACJ. The Janus face of ethylene: growth inhibition and stimulation. Trends Plant Sci 2006; 11:176 - 83; http://dx.doi.org/10.1016/j.tplants.2006.02.006; PMID: 16531097
- Iqbal N, Khan NA, Nazar R, da Silva JAT. Ethylene-stimulated photosynthesis results from increased nitrogen and sulfur assimilation in mustard types that differ in photosynthetic capacity. Environ Exp Bot 2012; 78:84 - 90; http://dx.doi.org/10.1016/j.envexpbot.2011.12.025
- Khan NA, Mir MR, Nazar R, Singh S. The application of ethephon (an ethylene releaser) increases growth, photosynthesis and nitrogen accumulation in mustard (Brassica juncea L.) under high nitrogen levels. Plant Biol (Stuttg) 2008; 10:534 - 8; http://dx.doi.org/10.1111/j.1438-8677.2008.00054.x; PMID: 18761492
- Iqbal N, Nazar R, Syeed S, Masood A, Khan NA. Exogenously-sourced ethylene increases stomatal conductance, photosynthesis, and growth under optimal and deficient nitrogen fertilization in mustard. J Exp Bot 2011; 62:4955 - 63; http://dx.doi.org/10.1093/jxb/err204; PMID: 21705383
- Leblanc A, Renault H, Lecourt J, Etienne P, Deleu C, Le Deunff E. Elongation changes of exploratory and root hair systems induced by aminocyclopropane carboxylic acid and aminoethoxyvinylglycine affect nitrate uptake and BnNrt2.1 and BnNrt1.1 transporter gene expression in oilseed rape. Plant Physiol 2008; 146:1928 - 40; http://dx.doi.org/10.1104/pp.107.109363; PMID: 18287493
- Li N, Parsons BL, Liu DR, Mattoo AK. Accumulation of wound-inducible ACC synthase transcript in tomato fruit is inhibited by salicylic acid and polyamines. Plant Mol Biol 1992; 18:477 - 87; http://dx.doi.org/10.1007/BF00040664; PMID: 1371404
- Alvarez I, Tomaro ML, Benavides MP. Changes in polyamines, proline and ethylene in sunflower calluses treated with NaCl. Plant Cell Tissue Organ Cult 2003; 74:51 - 9; http://dx.doi.org/10.1023/A:1023302012208
- Popova L, Maslenkova L, Yordanova R, Krantev A, Szalai G, Janda T. Salicylic acid protects photosynthesis against cadmium toxicity in pea plants. Gen Appl Plant Physiol 2008; 34:133 - 48
- Singh B, Usha K. Salicylic acid induced physiological and biochemical changes in wheat seedlings under water stress. Plant Growth Regul 2003; 39:137 - 41; http://dx.doi.org/10.1023/A:1022556103536
- Bates LE, Waldren RP, Teare ID. Rapid determination of free proline for water stress studies. Plant Soil 1973; 39:205 - 7; http://dx.doi.org/10.1007/BF00018060
- Hayzer DJ, Leisinger TH. The gene-enzyme relationships of proline biosynthesis in Escherichia coli.. J Gen Microbiol 1980; 118:287 - 93; PMID: 6255065
- Huang AHC, Cavalieri AJ. Proline oxidase and water stress induced proline accumulation in spinach leaves. Plant Physiol 1979; 63:531 - 5; http://dx.doi.org/10.1104/pp.63.3.531; PMID: 16660761
- Dhindsa RH, Plumb-Dhindsa P, Thorpe TA. Leaf senescence correlated with increased level of membrane permeability, lipid peroxidation and decreased level of SOD and CAT. J Exp Bot 1981; 32:93 - 101; http://dx.doi.org/10.1093/jxb/32.1.93
- Okuda T, Matsuda Y, Yamanaka A, Sagisaka S. Abrupt increase in the level of hydrogen peroxide in leaves of winter wheat is caused by cold treatment. Plant Physiol 1991; 97:1265 - 7; http://dx.doi.org/10.1104/pp.97.3.1265; PMID: 16668520
- Maxwell K, Johnson GN. Chlorophyll fluorescence--a practical guide. J Exp Bot 2000; 51:659 - 68; http://dx.doi.org/10.1093/jexbot/51.345.659; PMID: 10938857
- Kuo TM, Warner RL, Kleinhofs A. In vitro stability of nitrate reductase from barley leaves. Phytochemistry 1982; 21:531 - 3; http://dx.doi.org/10.1016/0031-9422(82)83134-8
- Nakagawa H, Poulle M, Oaks A. Characterization of Nitrate Reductase from Corn Leaves (Zea mays cv W64A x W182E): Two Molecular Forms of the Enzyme. Plant Physiol 1984; 75:285 - 9; http://dx.doi.org/10.1104/pp.75.2.285; PMID: 16663612
- Lindner RC. Rapid analytical methods for some of the more common inorganic constituents of plant tissues. Plant Physiol 1944; 19:76 - 89; http://dx.doi.org/10.1104/pp.19.1.76; PMID: 16653905
- Usuda H. The activation state of ribulose 1,5-bisphosphate carboxylase in maize leaves in dark and light. Plant Cell Physiol 1985; 26:1455 - 63
- Bradford MM. A rapid and sensitive method for the quantitation of microgram quantities of protein utilizing the principle of protein-dye binding. Anal Biochem 1976; 72:248 - 54; http://dx.doi.org/10.1016/0003-2697(76)90527-3; PMID: 942051
- Von Cammerer S, Farquhar GD. Some relationship between the biochemistry of photosynthesis and the gas exchange of leaves. Planta 1981; 153:376 - 87; http://dx.doi.org/10.1007/BF00384257
- Avni A, Bailey BA, Mattoo AK, Anderson JD. Induction of ethylene biosynthesis in Nicotiana tabacum by a Trichoderma viride xylanase is correlated to the accumulation of 1-aminocyclopropane-1-carboxylic acid (ACC) synthase and ACC oxidase transcripts. Plant Physiol 1994; 106:1049 - 55; http://dx.doi.org/10.1104/pp.106.3.1049; PMID: 7824643
- Woeste KE, Ye C, Kieber JJ. Two Arabidopsis mutants that overproduce ethylene are affected in the post-transcriptional regulation of 1-aminocyclopropane-1-carboxylic synthase. Plant Physiol 1999; 30:81 - 6