Abstract
In order to investigate the effects of root hypoxia (1-2 % oxygen) on the nitrogen (N) metabolism of tomato plants (Solanum lycopersicum L. cv. Micro-Tom), a range of N compounds and N-assimilating enzymes were performed on roots and leaves of plants submitted to root hypoxia at the second leaf stage for three weeks. Obtained results showed that root hypoxia led to a significant decrease in dry weight (DW) production and nitrate content in roots and leaves. Conversely, shoot to root DW ratio and nitrite content were significantly increased. Contrary to that in leaves, glutamine synthetase activity was significantly enhanced in roots. The activities of nitrate and nitrite reductase were enhanced in roots as well as leaves. The higher increase in the NH4+ content and in the protease activities in roots and leaves of hypoxically treated plants coincide with a greater decrease in soluble protein contents. Taken together, these results suggest that root hypoxia leaded to higher protein degradation. The hypoxia-induced increase in the aminating glutamate dehydrogenase activity may be considered as an alternative N assimilation pathway involved in detoxifying the NH4+, accumulated under hypoxic conditions. With respect to hypoxic stress, the distinct sensitivity of the enzymes involved in N assimilation is discussed.
Introduction
Plants grow in a dynamic environment, which frequently imposes constraints on growth and development. Among the adverse environmental factors commonly encountered by land plants, flooding is one of the most significant abiotic stresses.Citation1 Flooding of the soil can have a tremendous impact on the growth and survival of plants, and thereby on agricultural as well as natural ecosystems. In the last decades considerable progress has been made in our understanding of the mechanisms that enable certain plant species and cultivars to withstand periods with excess soil water or even complete submergence. Much of the research has been carried out with crop plant species, such as Oryza sativa, Zea mays, Trifolium subterraneum and Medicago sativa,Citation2–Citation5 but wild species originating from wetland habitats have been also used, mostly for comparative studies.Citation6,Citation7
With transient flooding or irrigation followed by slow drainage, or in natural wetlands, plant roots can become oxygen deficient because of slow transfer of dissolved oxygen in the water-filled pore space of the soil. The decrease in ambient oxygen may not be, itself or alone, the perceived signal but could trigger metabolic responses, which then initiate the signalling cascade.Citation8 The immediate consequence of oxygen depletion is the reduction in respiration, thus diminishing ATP generation, and leading to a decrease in the ATP/ADP ratio and the adenylate energy charge.Citation9 Changes in ATP level itself could conceivably serve as signal for subsequent adaptive responses. Low ATP levels lead to an energy crisis to which plants can respond by increasing glycolytic flux (Pasteur Effect) and switching to alcoholic fermentation,Citation3,Citation10 with a variety of electron acceptors such as pyruvate and acetaldehyde replacing oxygen. This metabolic adaptation helps maintain ATP production and NAD+ regeneration.Citation11 In addition, to cope with the reduction in oxygen supply, plants have developed a number of morphological adaptations that enable them to survive transient periods of complete or partial subme.12,Citation13 Escape from hypoxia involves shoot elongation, development of aerenchyma and adventitious root formation.Citation14,Citation15
Most studies on biochemical alterations in plants due to hypoxic stress have been focused on sugar metabolism.Citation16,Citation17 However, data regarding hypoxia effects on N metabolism steps have recently become available.Citation18,Citation19 The physiological roles of exogenous nitrate (NO3−) and nitrate reductase (NR) under the conditions of plant hypoxia and anoxia attracted the attention of researchers since NO3− is widely applied as a N fertilizer, in particular on wetland soils abundant in many countries.Citation20,Citation21 The role of NO3− reduction in oxygen deficiency tolerance is the subject of many controverses. Some investigations could not verify any effect of NO3− and NR on the energy metabolism in oxygen-deficient roots.Citation22,Citation23 However, other investigations have shown that NO3− supply to anaerobic root tissues led to an improved redox state (NADH/NAD+ ratio) and a higher adenylate energy charge,Citation24,Citation25 through the consumption of the reducing power (NADH) generated by the glycolysis and by the NO3− reduction to nitrite (NO2−). In these tissues, NR activity often increases, either through increased gene expression,Citation26 or post-translational activation.Citation27 These observations raised the hypothesis that NR could be a key enzyme to understand the beneficial impact of NO3− on plant survival under anoxia.
Ammonium (NH4+) originated from direct absorption, NR activity, photorespiration, dinitrogen fixation or deamination of nitrogenous compounds, is assimilated by the glutamine synthetase (GS) and the glutamate synthase (GOGAT). Under special conditions, glutamate dehydrogenase (GDH) is able to generate glutamate.Citation28 Despite its abundance in plant tissues, the precise function of GDH remains obscure. In physiological conditions, the essential function of this enzyme is the deamination of glutamate to provide carbon skeletons and reducing power for plant cells.Citation29 The aminating activity of GDH is specially stimulated in certain stress conditions: with senescence,Citation30 NH4+ excess,Citation31,Citation32 dark and atmospheric CO2 excess.Citation33 Generally, these conditions lead to a perturbation of the activities of enzymes involved in NH4+ assimilation, such as inhibition of GS,Citation34 and GOGAT.Citation35 However, the effects of root hypoxia on the activities of these enzymes are scarce in the literature.
In a previous study, we observed that root hypoxia affected not only the transport of nitrate from roots to shoots, but also its reduction to NO2− in clover plants.Citation32 The effects of root hypoxia on N metabolism are highly complicated, and vary depending on plant species and experimental conditions.Citation25 The aim of this study was to explore the effects of root hypoxia on N metabolism steps in tomato. We studied the behavior of the enzymes of nitrate and NH4+ assimilation in tomato leaves and roots under prolonged root hypoxia.
Results
Plant development and biomass allocation.
Root hypoxia was applied, in the present study, at the second leaf stage. At this moment, the initial root and shoot dry weights were 0.03 ± 0.01 g and 0.13 ± 0.03 g, respectively. The changes in the root and shoot dry weights (DW) and in shoot/root ratio were investigated after a three week period of hypoxic treatment. Root hypoxia decreased root and leaf DW production by 34 and 18%, respectively. Shoot to root DW ratio increased by 26% in hypoxically treated (HT) relative to control aerated (NT) plants (). This indicates that, under these growth conditions, the dry matter was more allocated to the aerial part. Root and leaf hydrations were decreased by 23 and 18%, respectively, in HT compared to NT plants. Concomitantly, leaf chlorophyll content was decreased by about 30%, whereas no obvious difference was observed in total leaf area ().
Nitrate, nitrite, ammonium and soluble protein contents in roots and leaves.
Roots and leaves were analyzed for their NO3−, NO2−, NH4+ and soluble protein contents (). Our results showed that, as for RondelloCitation19 and Rio GrandeCitation32 varieties, root hypoxia decreased root and leaf NO3− contents. These decreases were about 70 and 50%, respectively (). NO2− and NH4+ contents were eight times higher in roots of HT compared to those of NT plants (). Concomitantly, leaf NO2− and NH4+ contents were at least 4 fold higher (). Soluble protein was significantly decreased by root hypoxia both in roots and in leaves ().
Effects of root hypoxia on the N-metabolizing enzymes.
In the control tomato plants, we found that leaf NR activity was equal to that measured in roots, whereas under hypoxic conditions NR activity was 5 times higher in roots than in leaves. Root and leaf NR activities were 7- and 2-fold higher in HT compared to NT plants, respectively ().
In the aerated plants, NiR activity was two times higher in roots than in leaves. Under hypoxic treatment, root and leaf NiR activities were 2- and 3- fold higher in HT compared to NT plants, respectively. In the both conditions, root and leaf NiR activities were much higher than NR activities (). This ensures a rapid nitrite metabolism, protecting cells from NO2− toxicity. The NiR to NR ratio was highly decreased in the roots after a 3 week period of hypoxic treatment, whereas in the leaves it was significantly increased ().
GS activity was significantly inhibited in the leaves and slightly enhanced in the roots of HT compared to NT plants (). GDH activity was significantly enhanced by root hypoxia in roots as well as in leaves ().
Proteolytic activitiy was significantly enhanced by root hypoxia treatment in the leaves and roots (). This is in accordance with the decrease in soluble protein content (), and the increase in NH4+ accumulation ().
Discussion
The primary effect of soil flooding is to slow down the oxygen transfer to the roots. This limits their aerobic respiration and dramatically depresses their metabolism.Citation49 In the present study, the N metabolism was investigated in tomato plants after a three week period of hypoxic treatment.
Our results showed that root hypoxia led to some typical effects commonly observed in tomatoCitation36,Citation41 and in other plant species.Citation3,Citation4,Citation50,Citation51 These included a decrease in root hydration and DW production (). The decrease in DW production was suggested to be directly related to changes in net carbon assimilation that are attributed to stomatal and non-stomatal limitations of photosynthesis.Citation50
The consequences of oxygen deficiency were not restricted to roots. The effects of hypoxia also extended to aerial organs. Although all seedlings survived three weeks of flooding, some symptoms of hypoxic stress were observed. These included a slight chlorosis of the older leaves, a slowing of leaf initiation (data not shown) and decreases in leaf hydration and chlorophyll content ().
As NO3− is a key molecule to N metabolism, supporting the synthesis of plant N compounds, we investigated the effect of root hypoxia on NO3− reduction in tomato leaves and roots. It has been suggested that in higher plants, nitrate assimilation can take place in roots as well as in leaves, with the contribution of each organ being dependent on the species, on the external NO3− concentration and on the rate of NO3− assimilation in the aerial part of the plant.Citation52,Citation53 Our results showed that, under hypoxic conditions, more than 80% of NO3− reduction occurred in roots (). This result is in disagreement with the higher biomass production obtained in the leaves relative to the roots () and may be related to a sufficient availability of reducing power in this organ.Citation54
It is now well established that NO3− is the primary signal for the induction of its own transport system, as well as for the enzymes implicated in NO3− assimilation.Citation55 Previous works demonstrated that exogenous NO3− supplied to the medium of anaerobic excised rice roots increased the adenylic energy charge and lowered the catabolic reducing charge.Citation24 Both factors could contribute to root survival and suggest a possible role of NO3− reduction in oxygen deficiency tolerance. Moreover, in most plant tissues other than the rice coleoptile, NO3− reduction under flooded conditions is restricted to the conversion of NO3− to NO2−56 Our results showed that root hypoxia led to an activation or induction, of NiR in addition to the activation of NR (), which is observed in hypoxic,Citation57 as well as anoxic roots.Citation25,Citation58 Such an increase in NiR activity can be considered as a direct consequence of the increase in NR activity (). In fact, we can attribute the NiR activity enhancement to a higher availability of NO2− (). In addition, our results showed that under normoxic as well as hypoxic conditions, NiR activity was always much higher than NR activity (). This imbalance assures that toxic levels of NO2− are not reached in the cytosol. Ogawa et al.Citation59 confirmed the hypothesis of a co-regulation between NR and NiR, which is indispensable to prevent nitrite toxicity and to avoid the waste of electrons by NR and NiR activities.
In a previous study,Citation32 we showed that the increase in NiR activity under root hypoxia was restricted to roots and that no effects were observed on leaf NH4+ content and NiR activity in the Rio Grande variety. By contrast, leaf NH4+ content () and NiR activity () were significantly increased by root hypoxia, in the present study. The differences between results obtained for Micro-Tom and Rio Grande varieties may be related only to the plant material used (growth type: determinate versus indeterminate variety), since the experimental conditions were almost the same in the two studies.
Our results on NH4+-assimilating enzymes showed that, under hypoxic conditions, GS activity was inhibited in the leaves but enhanced in the roots (). The decrease in the leaf GS activity could derive from a low accessibility of glutamate for the GS enzyme. This is consistent with the fact that, in tomato, abiotic stresses lead to a high deviation of glutamate to the organic osmoticum synthesis.Citation60 Proline, putrescine and betaine derive from glutamate and they could restrict glutamate availability for GS activity. In the roots, GS activity increased as an attempt to overcome the GS activity decrease in the leaves. Similar results were found in tomato under cadmium stress. The GS activity decrease in leaves was due to a lower content of the chloroplastic GS isoform (GS2) protein and transcripts, whereas the increase in GS activity in roots was related to the induction of the cytosolic GS isoform (GS1) protein and transcripts.Citation61
The significant increase in NH4+ content in roots and leaves of HT relative to NT plants () was concomitant to a significant increase in the GDH activities (). Excessive NH4+ was shown to induce the aminating GDH pathway.Citation31,Citation62 In our case, the induction by root hypoxia of the aminating GDH pathway suggests that GDH may be involved in vivo in the NH4+ detoxification and in the replenishment of glutamate pool, which is highly required to produce protective metabolites (proline, phytochelatines, etc.).
The higher increase in the NH4+ content () and in the protease activity () in roots and leaves of HT plants coincide with a greater decrease in soluble protein content (). These results suggest that root hypoxia leaded to higher protein degradation. Such a process was observed in maize root tips,43,Citation63 and in tomato fruitsCitation64 and rootsCitation65 under sugar starvation or extended darkness.
In conclusion, this study demonstrates that, in tomato plants, root hypoxia (1) decreased root and leaf NO3− content and enhanced NR activity in the two organs. This process could be an adaptation of plant roots to transient hypoxic conditions.Citation66 (2) leaded to protein degradation via the induction of the protease activity. (3) accelerated N compounds synthesis and accumulation via the stimulation of NiR, GS and GDH activities. The hypoxia-induced increase in the aminating GDH activity may be considered as an alternative N assimilation pathway involved in the NH4+ detoxification and glutamate synthesis. However, the other pathways of glutamate synthesis cannot be excluded. More work at the molecular level will improve our understanding of nitrogen enzyme expressions and activities under hypoxic stress.
Material and Methods
Plant material and growth conditions.
Tomato (Solanum lycopersicum L. cv. Micro-Tom) seeds were germinated on filter paper moistened with distilled water for 1 week at 23°C in the dark, and then grown hydroponically in a growth chamber (16 h light at 23°C/8 h dark at 18°C with an irradiance of 350 µmolm−2s−1 and 75–80% relative humidity). Each seedling was placed in a 25 ml vermiculite plug on a polystyrene tray floating on the nutrient solution, with 6 plants per 10 l tank. The nutrient solution, pH 5.8, contained macronutrients: 3 mM KNO3, 1 mM Ca(NO3)2, 2 mM KH2PO4, 0.5 mM MgSO4, 32.9 µM EDTA-Fe, and micronutrients: 30 µM H3BO4, 5 µM MnSO4, 1 µM CuSO4, 1 µM ZnSO4, 1 µM (NH4)6Mo7O24. Hypoxic treatment was applied at the second leaf stage (one week after transplanting) for three weeks by stopping air bubbling. Oxygen shortage was imposed progressively by root oxygen consumption as in Horchani et al.Citation36,Citation37 except that before weekly medium renewal, the new nutrient solution was flushed with nitrogen down to about 2% O2 in order to maintain a low oxygen pressure without re-oxygenation of the roots. Control plants were continuously bubbled with air.
Vegetative growth analysis.
Three weeks after root hypoxia application (at the sixth leaf stage), plants were harvested and divided into roots and shoots. Roots were washed in distilled water. Fresh weights (FW) were immediately determined for roots and shoots. Dry weights (DW) were obtained by weighing the plant material after drying at 80°C until a constant mass was reached. DW production was estimated as the difference between the DWf obtained at the end of the experiment and the DW0 measured just before root hypoxia application. Leaf area (LA) and chlorophyll content were measured as in Horchani et al.Citation37
Nitrate, nitrite, ammonium and soluble protein assay.
For NO3−, NO2− and protein determination, leaf and root samples of three-week-old plants were ground thoroughly with a pestle and mortar in 500 mM Tris-HCl pH 7.5, and centrifuged at 20,000 g for 15 min. NO3− and NO2− were assayed in plant tissues using the salicylic acid-sulfuric acid method,Citation38 and the Griess reagent method,Citation39 respectively. Total soluble proteins were assayed according to BradfordCitation40 using γ-globulin as a standard. NH4+ was extracted using 6% (w/v) TCA as in Horchani et al.Citation41 and assayed spectrophotometrically in sample extracts using the phenol-hypochlorite method.Citation42
Enzyme assays.
Nitrate and nitrite reductase. Tissue samples were ground at 4°C using an extraction buffer containing 25 mM Tris-HCl pH 8.5, 1 mM EDTA, 20 µM FAD, 1 mM dithiothreitol (DTT), 1% (w/v) bovine serum albumin (BSA), 10 mM cystein, 20 µM E64 and 2 mM phenyl-methyl-sulphonyl-fluoride (PMSF). The crude extracts were centrifuged at 20,000 g for 15 min and the supernatants were assayed for nitrate and nitrite reductase activities.
Nitrate reductase activity assay was adopted from Miranda et al.Citation39 The reaction medium (1 ml) contained 0.2 M Hepes pH 7.0, 15 mM KNO3 and 250 µM NADH. Reaction was initiated by NO3− addition, run at 28°C and then stopped after 30 min by adding 10 µl ADH (180 units/ml) and 10 µl acetaldehyde (4% v/v). The coloured reaction was produced by adding 480 µl of nitrite reagent (240 µl of 1% (w/v) sulphanilamide in 1 N HCl, plus 240 µl of 0.01% (w/v) N-1-naphtylenediamine dihydrochloride in water) and incubating for 30 min at room temperature. The absorbance was read at 540 nm. Nitrite reductase (NiR) activity was assayed by following NO2− consumption from the assay mixture using the dithionite-methyl viologen method.43 The reaction medium (1 ml) consisted of 20 mM potassium phosphate (pH 7.3), 1 mM NaNO2, 40 µM methyl viologen and the sample to be assayed. The reaction was started by addition of 10 µl of 100 mM sodium dithionite in 200 mM sodium bicarbonate and run at 28°C. Samples were maintained under anaerobiosis during the incubation. After 30 min, 20 µl aliquot fractions were sampled, diluted in 480 µl H2O, and shaken energetically for 30 s. A 500 µl aliquot of the NO2− reagentCitation39 was then added, and the absorbance measured at 540 nm after 30 min. Assay blanks contained enzymic extract plus reagents except dithionite.
Glutamine synthetase. Glutamine synthetase (GS) was extracted in a medium containing 0.1 M Tricine (pH 8.0), 20 mM MgSO4, 10 µM chymostatin and 1 mM EDTA. The supernatant of the crude extract was used for enzymic measurement. GS was determined according to a modified version of the method of O'Neal and Joy.Citation44 The assay mixture (1 ml) consisted of 0.1 M Tricine (pH 7.8), 4 mM MgSO4, 0.2 mM EDTA, 80 mM glutamate, 6 mM NH2OH, 8 mM ATP and enzyme extract. The reaction was started by addition of NH2OH and terminated, after 10 min at 37°C, with 1 ml FeC13 reagent. Extracts were then centrifuged and the absorbance of the γ-glutamyl hydroxamate (GHM) formed was measured at 540 nm. Assay blanks contained all the reagents except ATP.
Glutamate dehydrogenase. Glutamate dehydrogenase (GDH) extraction was performed according to the method described by Magalhaes and Huber.Citation45 Frozen samples were homogenized in a cold mortar and pestle with 100 mM Tris-HCl (pH 7.5), 14 mM β-mercaptoethanol and 1% (w/v) polyvinyl polypyrrolidone (PVP). The extract was centrifuged at 12,000 g for 15 min at 4°C. GDH activity was determined in the aminating direction by following the absorption change at 340 nm by NADH oxidation.Citation46
Protease.
Protease activity was measured by the method of Weckenmann and Martin,Citation47 using azocasein as substrate. Absorbance of the released-azo-dye- was measured at 340 nm and one unit of activity was defined as the activity producing an increase of 0.01 units of absorbance during 1 h incubation.Citation48
Statistics.
Statistical data analysis was made using the Student's t-test. The results are given as means (±SD) of at least six replicates per treatment. The significance of differences between the control and the treatment mean values was determined at the significance level of p < 0.05. Experiments were replicated two to three times.
Figures and Tables
Figure 1 Nitrate (A), nitrite (B), ammonium (C) and soluble protein (D) contents in root and leaf tissues of aerated (□) and hypoxically treated (■) tomato plants for three weeks. Values are the mean of six replicates ± SD. *The significance of differences between the control and the treatment mean values was determined by the Student's t-test at the significance level of p < 0.05.
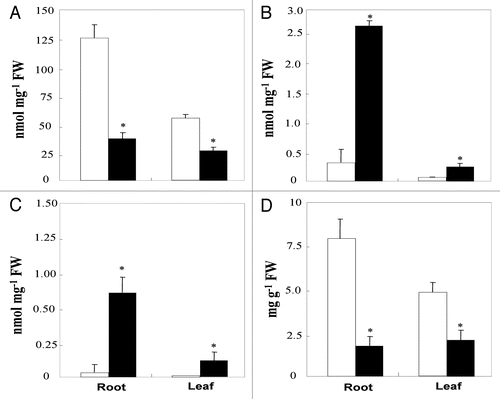
Figure 2 Glutamine synthetase (A), glutamate dehydrogenase (B) and protease (C) activities in roots and leaves of aerated (□) and hypoxically treated (■) tomato plants for three weeks. Values are the mean of six replicates ± S.D. *The significance of differences between the control and the treatment mean values was determined by the Student's t-test at the significance level of p < 0.05.
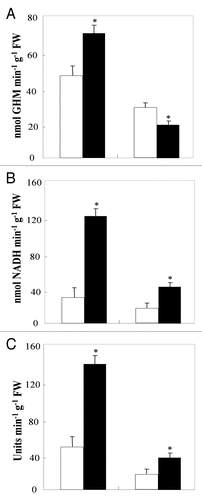
Table 1 Root and shoot dry weights (DW), shoot/root ratio, root and leaf water contents, leaf area and leaf chlorophyll content of tomato plants grown in aerated (NT) and hypoxic (HT) nutrient solution
Table 2 Changes in nitrate reductase (NR) and nitrite reductase (NiR) activities, and NiR/NR ratio in roots and leaves of aerated (NT) and hypoxically treated (HT) tomato plants for three weeks
References
- Blom CWPM, Voeseneck LACJ. Flooding: the survival strategies of plants. Trends Ecol Evol 1996; 11:290 - 295
- Vartapetian BB, Jackson MB. Plant adaptations to anaerobic stress. Ann Bot 1997; 79:3 - 20
- Aschi-Smiti S, Bizid E, Hamza M. Effet de l'hydromorphie sur la croissance de 4 variétés de trèfle (Trifolium subterraneum L.). Agronomie 2002; 23:97 - 104
- Aschi-Smiti S, Chaibi W, Brouquisse R, Ricard B, Saglio P. Assessment of enzyme induction and aerenchyma formation as mechanisms for flooding tolerance in Trifolium subterraneum ‘Park’. Ann Bot 2003; 91:195 - 204
- Irving LJ, Sheng YB, Woolley D, Matthew C. Physiological effects of waterlogging on two lucerne varieties grown under glasshouse conditions. J Agron Crop Sci 2007; 193:345 - 356
- Justin SHFW, Armstrong W. The anatomical characteristics of roots and plant response to soil flooding. New Phytol 1987; 106:465 - 495
- Visser EJW, Blom CWPM, Voesenek LACJ. Flooding-induced adventitious rooting in Rumex: morphology and development in an ecological perspective. Acta Bot Neerl 1996; 45:17 - 28
- Ricard B, Aschi-Smiti S, Gharbi I, Brouquisse R. Huang B. Cellular and molecular mechanisms of plant tolerance to waterlogging. In Plant-Environment Interactions 2006; Boca Raton/London/New York Taylor and Francis 177 - 208
- Gharbi I, Ricard B, Rolin D, Maucourt M, Andrieu MH, Bizid E, et al. Effect of hexokinase activity on tomato root metabolism during prolonged hypoxia. Plant Cell Environ 2007; 30:508 - 517
- Ricard B, Couee I, Raymond P, Saglio P, Saint-Ges V, Pradet A. Plant metabolism under hypoxia and anoxia. Plant Physiol Biochem 1994; 32:1 - 10
- Dennis ES, Dolferus R, Ellis M, Rahman M, Wu Y, Hoeren FU, et al. Molecular strategies for improving waterlogging tolerance in plants. J Exp Bot 2000; 51:89 - 97
- Bailey-Serres J, Voesenek LACJ. Flooding stress: acclimations and genetic diversity. Annu Rev Plant Biol 2008; 59:313 - 339
- Voesenek LA, Colmer TD, Pierik R, Millenaar FF, Peeters AJ. How plants cope with complete submergence. New Phytol 2006; 170:213 - 226
- Perata P, Voesenek LA. Submergence tolerance in rice requires Sub1A, an ethylene-response-factor-like gene. Trends Plant Sci 2007; 12:43 - 46
- Alonso AP, Vigeolas H, Raymond P, Rolin D, Dieuaide-Noubhani M. A new substrate cycle in plants. Evidence for a high glucose phosphate-to-glucose turnover from in vivo steady-state and pulse labeling experiments with [13C] glucose and [14C] glucose. Plant Physiol 2005; 138:2220 - 2232
- Alonso AP, Raymond P, Rolin D, Dieuaide-Noubhani M. Substrate cycles in the central metabolism of maize root tips under hypoxia. Phytochem 2007; 68:2222 - 2231
- Allegre A, Silvestre J, Morard P, Kallerhoff J, Pinelli E. Nitrate reductase regulation in tomato roots by exogenous nitrate: a possible role in tolerance to long-term root anoxia. J Exp Bot 2004; 55:2625 - 2634
- Morard P, Lacoste L, Silvestre J. Effect of oxygen deficiency on mineral nutrition of excised tomato roots. J Plant Nutr 2004; 27:1532 - 1537
- Heeb A, Lundegardh B, Ericson T, Savage GP. Effects of nitrate, ammonium and organic-nitrogen-based fertilizers on growth and yield of tomatoes. J Plant Nutr Soil Sci 2005; 168:123 - 129
- Vartapetian BB. Plant anaerobic stress as a novel trend in ecological physiology, biochemistry and molecular biology: further development of the problem. Russ J Plant Physiol 2006; 53:711 - 738
- Lee RB. The release of nitrite from barley roots in response to metabolic inhibitors, uncoupling agents and anoxia. J Exp Bot 1979; 30:119 - 133
- Saglio P, Drew MC, Pradet A. Metabolic acclimatation to anoxia induced by low (2–4 KPa partial pressure) oxygen pretreatment (hypoxia) in root tips of Zea mays. Plant Physiol 1988; 8:61 - 66
- Reggiani R, Brambilla I, Bertani A. Effect of exogenous nitrate on anaerobic metabolism in excised rice roots—Nitrate reduction and pyridine nucleotide pools. J Exp Bot 1985; 36:1193 - 1199
- Stoimenova M, Hansch R, Mendel R, Gimmler H, Kaiser WM. The role of nitrate reduction in the anoxic metabolism of roots. I. Characterization of root morphology and normoxic metabolism of wild type tobacco and a transformant lacking root nitrate reductase. Plant Soil 2003; 253:145 - 153
- Mattana M, Brambilla I, Bertani A, Reggiani R. Expression of nitrogen assimilating enzymes in germinating rice under anoxia. Plant Physiol Biochem 1996; 34:653 - 657
- Botrel A, Kaiser WM. Nitrate reductase activation state in barley roots in relation to the energy and carbohydrate status. Planta 1997; 201:496 - 501
- Srivastava HS, Singh RP. Role and regulation of L-glutamate dehydrogenase activity in higher plants. Phytochem 1987; 26:597 - 610
- Robinson SA, Slade AP, Fox GG, Phillips R, Ratcliffe RG, Stewart GR. The role of glutamate dehydrogenase in nitrogen metabolism. Plant Physiol 1991; 95:509 - 516
- Loulakakis KA, Roubelakis-Angelakis KA, Kanellis AK. Regulation of glutamate dehydrogenase and glutamate synthase in avocado fruit during development and ripening. Plant Physiol 1996; 76:536 - 540
- Osuji GO, Madu WC. Ammonium ion-dependant isomerisation of glutamate dehydrogenase in relation to glutamate synthesis in maize. Phytochem 1995; 39:495 - 503
- Horchani F, Aschi-Smiti S, Brouquisse R. Involvement of nitrate reduction in the tolerance of tomato plants to prolonged root hypoxia. Acta Physiol. Plant 2010; 32:1113 - 1123; http://dx.doi.org/10.1007/s11738-010-0503-0
- Refouvelet E, Daguin F. Polymorphic glutamate dehydrogenase in lilac vitroplants as revealed by combined preparative IEF and native PAGE: effect of ammonium deprivation, darkness and atmospheric CO2 enrichment upon isomerization. Physiol Plant 1999; 105:199 - 206
- Kamachi K, Yamaya T, Mae T, Ojima K. A role for glutamine synthetase in the remobilization of leaf nitrogen during natural senescence in rice leaves. Plant Physiol 1991; 96:411 - 417
- Hetch U, Oelmiller R, Schmidt R, Mohr H. Action of light, nitrate and ammonium on the level NADH and ferredoxin dependent glutamate synthase in the cotyledons of mustard seedlings. Planta 1988; 175:130 - 138
- Horchani F, Aloui A, Brouquisse R, Aschi-Smiti S. Physiological responses of tomato plants (Solanum lycopersicum) as affected by root hypoxia. J Agron Crop Sci 2008; 194:297 - 303
- Horchani F, Khayati H, Raymond P, Brouquisse R, Aschi-Smiti S. Contrasted effects of prolonged root hypoxia on tomato (Solanum lycopersicum) roots and fruits metabolism. J Agron Crop Sci 2009; 195:313 - 318
- Cataldo DA, Haroon M, Schrader LE, Youngs VL. Rapid colorimetric determination of nitrate in plant tissue by nitration of salicylic acid. Commun Soil Sci Plant Analys 1975; 6:71 - 80
- Miranda KM, Espey MG, Wink DA. A rapid, simple spectrophotometric method for simultaneous detection of nitrate and nitrite. Nitric Oxide 2001; 5:62 - 71
- Bradford MM. A rapid and sensitive method for the quantification of microgram quantities of proteins utilizing the principle of protein-dye binding. Anal Biochem 1976; 72:248 - 254
- Horchani F, Gallusci P, Baldet P, Cabasson C, Maucourt M, Rolin D, et al. Prolonged root hypoxia induces ammonium accumulation and decreases the nutritional quality of tomato fruits. J Plant Physiol 2008; 165:1352 - 1359
- Brouquisse R, James F, Raymond P, Pradet A. Study of glucose starvation in excised maize root tips. Plant Physiol 1991; 96:619 - 626
- O'Neal D, Joy KW. Glutamine synthetase of pea leaves. I. Purification stabilization and pH optima. Arch Biochem Biophys 1973; 159:113 - 122
- Magalhaes JR, Huber DM. Response of ammonium assimilation enzymes to nitrogen form treatments in different plant species. J Plant Nutr 1991; 14:175 - 185
- Loyala-Vergas VM, de Jimenez ES. Differential role of glutamate dehydrogenase in nitrogen metabolism of maize tissues. Plant Physiol 1984; 76:536 - 540
- Weckenmann D, Martin P. Endopeptidase activity and nitrogen mobilisation in senescing leaves of Nicotiana rustica in light and dark. Physiol Plant 1984; 60:333 - 340
- Debouba M, Gouia H, Suzuki A, Ghorbel MH. NaCl stress effects on enzymes involved in nitrogen assimilation pathway in tomato “Lycopersicon esculentum” seedlings. J Plant Physiol 2006; 163:1247 - 1258
- Mommer L, Pederson O, Visser EJW. Acclimation of a terrestrial plant to submergence facilitates gas exchange under water. Plant Cell Environ 2004; 27:1281 - 1287
- Pezeshki SR. Wetland plant responses to soil flooding. Environ Exp Bot 2001; 46:299 - 312
- Henshaw TL, Gilbert RA, Scholberg JMS, Sinclair TR. Soya bean (Glycine max L. Merr.) genotype response to early-season flooding: II. Aboveground growth and biomass. J Agron Crop Sci 2007; 193:189 - 197
- Gojon A, Bussi C, Grignon C, Salsac L. Distribution of NO3− reduction between roots and shoots of peach tree seedlings as affected by NO3− uptake rate. Physiol Plant 1991; 82:502 - 512
- Peuke AD, Glaab J, Kaiser WM, Jeschlke WD. The uptake and flow of C and N and ions between roots and shoots in Ricinus communis L. IV. Flow and metabolism of inorganic nitrogen and malate depending on nitrogen nutrient and salt treatment. J Exp Bot 1996; 47:377 - 385
- Lillo C. Amancio S, Stulen I. Light regulation of nitrate uptake, assimilation and metabolism. Plant ecophysiology. Nitrogen acquisition and assimilation in higher plants 2004; Dordrecht Kluwer Academic Press Publisher 149 - 184
- Crawford NM. Nitrate: nutrient and signal for plant growth. Plant Cell 1995; 7:859 - 868
- Lee RB. Inorganic nitrogen metabolism in barley root under poorly aerated conditions. J Exp Bot 1978; 29:693 - 708
- Drew MC. Oxygen deficiency and root metabolism: injury and acclimation under hypoxia and anoxia. Annu Rev Plant Physiol Plant Mol Biol 1997; 48:223 - 250
- Garcia-Novo F, Crawford RMM. Soil aeration, nitrate reduction and flooding tolerance in higher plants. New Phytol 1973; 72:1031 - 1039
- Ogawa K, Soutome R, Hiroyama K, Hagio T, Ida S, Nakagawa H. Co-regulation of nitrate reductase and nitrite reductase in cultured spinach cells. J Plant Physiol 2000; 157:299 - 306
- Santa-Cruz A, Acosta M, Rus A, Bolarin MC. Short-term salt tolerance mechanisms in differentially salt tolerant tomato species. Plant Physiol Biochem 1999; 37:65 - 71
- Chaffei C, Pageau K, Suzuki A, Gouia H, Ghorbel MH, Masclaux-Daubresse C. Cadmium toxicity induced changes in nitrogen management in Lycopersicon esculentum leading to a metabolic safeguard through an amino acid storage strategy. Plant Cell Physiol 2004; 45:1681 - 1693
- Boussama N, Ouariti O, Ghorbel MH. Changes in growth and nitrogen assimilation in barley seedlings under cadmium stress. J Plant Nutr 1999; 22:731 - 752
- Brouquisse R, Gaudillere JP, Raymond P. Induction of a carbon-starvation-related proteolysis in whole maize plants submitted to light/dark cycles and to extended darkness. Plant Physiol 1998; 117:1281 - 1291
- Baldet P, Devaux C, Chevalier C, Brouquisse R, Just D, Raymond P. Contrasted responses to carbohydrate limitation in tomato fruit at two stages of development. Plant Cell Environ 2002; 25:1639 - 1649
- Devaux C, Baldet P, Joubès J, Dieuaide-Noubhani M, Just D, Chevalier C, et al. Physiological, biochemical and molecular analysis of sugar-starvation responses in tomato roots. J Exp Bot 2003; 54:1143 - 1151
- Morard P, Lacoste L, Silvestre J. The effect of oxygen deficiency on the uptake of water and mineral nutrients by tomato plants in soilless culture. J Plant Nutr 2000; 23:1063 - 1078