Abstract
The directional transport of the plant hormone auxin depends on transcellular gradients of auxin-efflux carriers that continuously cycle between plasma membrane and intracellular compartments. This cycling has been proposed to depend on actin filaments. However, the role of actin for the polarity of auxin transport has been disputed. To get insight into this question, actin bundling was induced by overexpression of the actin-binding domain of talin in tobacco BY-2 cells and in rice plants. This bundling can be reverted by addition of auxins, which allows to address the role of actin organization on the flux of auxin. In both systems, the reversion of a normal actin configuration can be restored by addition of exogenous auxins and this fully restores the respective auxin-dependent functions. These findings lead to a model of a self-referring regulatory circuit between polar auxin transport and actin organization. To further dissect the actin-auxin oscillator, we used photoactivated release of caged auxin in tobacco cells to demonstrate that auxin gradients can be manipulated at a subcellular level.
Why Plant Cells Continuously have to Ask for the Right Direction
When plants began to settle on terrestrial habitats, they had to develop flexible and simultaneously robust mechanical lattices to overcome the constraints of mechanical load that was not any longer compensated by buoyancy. The invention of load-bearing modules consisting of central vasculature, surrounded by parenchymatic tissue and a water-tight epidermis with controllable stomata, the so called telomes, was a key achievement in the success story of land plants.Citation1 Asymmetric branching of the originally dichotomous telomes, their planation with subsequent fusion, or their spherical fusion and subsequent reduction, generated all organs found in the recent true land plants. These transitions are well documented by fossile records, leading to the conclusion that the Bauplan of land plants can be explained by patterning of vasculature as core element of the telomic building blocks. p ]The central regulator for patterning is the plant hormone auxin.Citation2 Auxin-dependent patterning is linked to a directional flow of auxin, a cell-to-cell process described by a modified chemiosmotic model.Citation3 This means that patterning is driven by cell polarity. The self-amplification of cell polarity by a polar auxin flow has been linked with directional intracellular traffic that contains positive feedback loops in combination with lateral inhibition resulting in an ordered pattern.Citation4 This mechanism has been elegantly demonstrated for the venation of developing leavesCitation5 or the patterning of meristems,Citation6 and ultimately dependends on the directionality of auxin transport. In the meantime, several plant-specific pinformed (PIN) proteins have been identified as candidates for auxin-efflux facilitator proteins,Citation7 and despite a long debate on the actual function of these proteins, the most recent results show that they are in fact rate-limiting for auxin efflux.Citation8
The polar localization of the PIN-proteins is not static, but corresponds to a dynamic equilibrium between endocytotic uptake into endosomal compartments and exocytotic targeting to the plasma membrane. This was concluded from experiments with Brefeldin A (BFA)Citation9,Citation10 that caused a redistribution of the PIN1 protein into intracellular compartments. This so called PIN-cycling is controlled by small GTPases, the ADP-ribosylation factors (ARF) and their associated guanine nucleotide exchange factorsCitation11 that are the targets of BFA. A mutation of one of these guanine nucleotide exchange factors causes the gnom mutant phenotype, where PIN1 becomes trapped in endosomal compartments. However, the link between ARFs and PIN has been challenged by experiments, where the internalization of PIN2 was blocked by tyrphostin, an inhibitor of clathrin-mediated endocytosis.Citation12
What does Actin Do in Auxin Transport-Does it?
Inhibitors of actin assembly suppress the effect of BFA on PIN1 internalization, cause a rapid internalization of PIN3,Citation13 and disturb the polar localization of PIN1,Citation10 and, with even higher efficiency, of the auxin-efflux carrier AUX1.Citation14 On the other hand, treatment of zygotes of the brown alga Fucus by phytotropins impaired both the organization of actin filaments, and the induction of developmental polarity by light or gravity.Citation15 In addition, the cycling of PIN-proteins between plasma membrane and intracellular compartments is regulated by auxin itself.Citation16 These observations have led to models that link the polar localization of the PIN-proteins to actin-dependent transport.Citation17,Citation18 However, this presumed link has been recently questioned by experiments, where PIN1 and PIN2 maintained their polar localization, although actin filaments had been eliminated by the artificial auxin 2,4-dichlorophenoxy-acetic acid (2,4-D), or the phytotropin naphthylphthalamic acid (NPA).Citation19
On the other hand, the phytotropin tri-iodobenzoic acid (TIBA) induced actin bundling not only in plants, but also in mammalian and yeast cells, i.e., in cells that are not to be expected to utilize auxin as signaling compound.Citation20 This was interpreted as supportive evidence for a role of actin filaments in polar auxin transport. Interestingly, the phytotropin action of TIBA along with N-ethyl-maleimide was originally discovered when the role of SH-rich proteins for auxin transport was investigated.Citation21 Both compounds had been classically used as inhibitors of sulfhydryl groups and N-ethylmaleimide was widely used as efficient blocker of actomyosin activity, for instance in the context of cytoplasmic streaming.Citation22 Thus, the observation that TIBA inhibits auxin flux by bundling actinCitation20 is congruent with the classical use of SH-blockers to inhibit functions of actomyosin.
This collection of partially contradicting observations illustrates that, although actin seems to play a role for the polarity of auxin fluxes; this issue is, first, not simple and, second, far from being understood.
Auxin Alters the Organization of Actin
During the past decade we have been analyzing the response of actin filaments during auxin-dependent cell growth in Graminean coleoptiles. To understand the role of actin in signal-dependent growth, we initially analyzed phytochrome-triggered cell elongation in maize coleoptilesCitation23 and could define two populations of actin filaments (F-actin) that were functionally different. In cells that underwent rapid elongation, F-actin was organized into fine strands that became bundled in response to conditions that inhibited growth. This transition was rapid and preceded the changes in growth rate. Moreover, this response was confined to the epidermis, i.e., to the target tissue for the signal control of growth.Citation24
We later succeeded to separate the two actin populations biochemically due to differences in sedimentability.Citation25 Whereas the fine actin filaments correlated with a cytosolic fraction of actin, actin became progressively trapped on the endomembrane system and partitioned into the microsomal fraction when bundling induced by light (perceived by phytochrome), by fluctuations of auxin content or by Brefeldin A. This bundling of actin was accompanied by a shift of the dose-response of auxin-dependent cell elongation towards higher concentrations and thus to a reduced auxin-sensivity. This led to a model, where auxin signaling triggered the reorganization of F-actin bundles into finer filaments that more efficiently transported auxin-signaling/transport components towards the cell pole. This debundling response of actin in response to auxin could later be demonstrated in intact rice coleoptiles in vivo using the actin binding domain of mouse talin in fusion with the yellow fluorescent protein (YFP) first upon transient,Citation26 later after stable expression.Citation27
The Auxin-Actin Circuit in Patterned Cell Division
In addition to cell expansion, auxin can induce cell division through a G-protein dependent pathway.Citation28 This pathway is preferentially triggered by 2,4-dichlorophenoxy-acetic acid (2,4-D). In contrast, cell expansion is preferentially triggered by 1-naphthalene acetic acid (NAA) using a pathway that is independent of G-proteins.Citation29 When the effect of auxin on root growth in Arabidopsis thaliana was assessed separately for the contributions of cell division and cell elongation,Citation19 the natural auxin indole-acetic acid (IAA), along with NAA and TIBA were found to inhibit cell elongation with little effect on filamentous actin. In contrast, 2,4-D and NPA impaired cell proliferation and eliminated actin filaments. This indicates that auxins regulate cell expansion and cell division through two distinct pathways that not only differ with respect to the role of G-proteins, but also with respect to the role of actin filaments. To what extent the auxin effect on cell division involves corresponding redistributions of PIN-proteins remains to be elucidated. A part of the controversy on this aspect (see above) might arise from the fact that the root is a very complex system consisting of different tissue layers that differ with respect to molecular machinery, auxin sensitivity, and cytoskeletal organization. Moreover, the frequency of cycling cells, even in a rapidly growing root, is relatively modest.
As simple model to study cellular aspects of cell division, the tobacco cell line Bright Yellow 2 (BY-2) has been very useful over many years. In this system, the role of different phytohormones has been studied in detail,Citation30 and the possibility to synchronize BY-2,Citation31 provided a powerful approach to detect fluctuations of hormone levels during cell division.Citation32 Moreover, we had observed that cell divisions within individual cell files are partially synchronized, leading to a much higher frequency of cell files with even cell numbers as compared to files with uneven cell numbers.Citation33,Citation34 This synchrony of cell divisions could be inhibited by low concentrations of NPA. Although it has been known for a while that auxin is necessary for the progress of the cell cycle, and thus can be used to synchronize the cell cycle in plant cell cultures,Citation35 this was the first time that auxin was shown to coordinate the divisions of adjacent cells.
Using the auxin-dependent synchrony, we addressed the role of actin in auxin-signaling using patterning as sensitive trait to monitor changes of polar auxin fluxes.Citation34 If actin is part of an auxin-driven feedback loop, it should be possible to manipulate auxin-dependent patterning through manipulation of actin. To test this prediction, we used a genetic approach, where we expressed the actin-binding domain of mouse talin in fusion with YFP. Mouse talin has been proposed to compete with endogenous actin-depolymerization factors for binding sites on actin such that the actin filaments are progressively trapped in a bundled configuration.Citation36 In fact, a BY-2 cell line overexpressing the actin-binding domain of mouse talin in fusion with YFP exhibited constitutively bundled actin filaments. As expected, the synchrony of cell division was impaired in this line, but could be restored by addition of transportable auxins such as IAA and NAA (but not by 2,4-D) along with a normal organization of actin. A:thus not only responds to changes in the cellular content of auxin, but actively participates in the establishment of the polarity that drives auxin transport.
In the search for factors that regulate organization and polarity of actin filaments, we cloned the tobacco actin-related protein 3, a component of the actin-nucleation complex, as a bonafide marker for sites of actin nucleation.Citation37 By biolistic transient transformation of tobacco cells we could, for the first time, visualize ARP3 in living plant cells. Using dual fluorescence visualization of actin (by a GFP fusion of the actin-binding site of fimbrin) and RFP-ARP3 we were able to confirm that ARP3 decorates actin filaments in vivo. When actin filaments were transiently eliminated (either by treatment with cytochalasin D or be cold treatment) and then allowed to recover, ARP3 marked the sites, from which the new filaments emanated. Thus, this marker allowed to followed the behavior of actin-nucleation sites through patterned cell division and to observe that the apical cells of a file behaved differently from the cells in the center of a cell file.
The apical cells are distinct by their characteristic morphology (conical shape, distinct polarity) from the isodiametric cells in the center of a file. ARP3 accumulated in the apex of these apical cells, whereas PIN1 was concentrated at the proximal cross wall. Upon disintegration of the file into single cells, this gradient of ARP3 persisted, whereas PIN1 was redistributed uniformly over the plasma membrane. The apical cells divided in the mode of a stem cell, where the terminal daughter cell inherited the features of an apical cell, whereas the proximal daughter cell was isodiametric and developed the characteristics of a central cell. Interestingly, during the asymmetric divisions of apical cells, the ARP3-gradient was maintained in the terminal daughter cell, but it was lost in the proximal daughter cell. However, when the cell files extended by additional divisions, eventually a new ARP3 gradient (culminating in the distal pole, i.e., opposite to the gradient in the apical cell) in the terminal cell opposed to the original apex. Upon disintegration, these basal cells behave in the same way as the apical cells. The fate of the central cells of a file differs from that of the two terminal cells. In the central cells, ARP3 is distributed uniformly, and upon disintegration these cells divide symmetrically producing first bicellular, and then in a second round of synchronous divisions, quadricellular files. During those later stages ARP3 is redistributed in the terminal cells of the file such that it accumulates in the distal cell poles. Thus, although the final result resembles that in a cell file originating from a terminal progenitor cell, the pathway differs. In the developmental pathway of a terminal progenitor, the ARP3 gradient is present a priori, during the development of a central progenitor, the ARP3 gradient is generated a posteriori from an ancestral state that is symmetric.
These findings indicate that actin nucleation is upstream of developmental polarity and the polar distribution of PIN1. Alternatively, ARP3 and PIN1 might be distributed in parallel by an unknown event that is expressed in response to cell polarity.
Interestingly, the asymmetric divisions of terminal cells follow a constant geometric proportionality, whereby the ratio between mother cell and the respective larger daughter cell remains constant. In other words: the division obeys the principle of the ‘golden section’. However, this ratio can be shifted by NPA, by addition of transportable auxin, or by 2,3-butanedione monoxime, an inhibitor of myosin motor function, indicating that the plane of cell division is regulated by actomyosin and auxin (Maisch and Nick P, unpublished results).
The Auxin-Actin Circuit in Cell Elongation
The suppression of auxin-dependent division synchrony by talininduced bundling of actin and it rescue by polarly transportable auxins suggested a causal link between actin organization and auxin transport. However, the readout, synchrony of cell division, was indirect—the argument would be more straightforward, if the transport of auxin could be measured directly rather than being monitored by a transport-dependent developmental process. To clarify this issue, we decided to test the relationship between actin organization and auxin transport in a system, where both parameters are amenable to observation and manipulation. We therefore returned to the classical system for the physiology of auxin transport, the Graminean coleoptile. To manipulate actin organization, we used again the approach to expressed the actinbinding protein mouse talin in fusion with the yellow fluorescent protein.Citation27 By utilizing different, independent transgenic lines, we were able to obtain variable degrees of F-actin bundling depending on the expression level of mouse talin. Whereas a moderate expression of talin left the configuration and dynamics of actin basically untouched, we observed bundling of actin filaments in lines that overexpressed talin strongly. In these strong expressor lines the characteristic alterations of actin organization and dynamics were accompanied by alterations of actin-dependent growth responses such as gravitropic bending or auxin-induced cell elongation. The sensitivity of these physiological responses to latrunculin B (that acts by sequestering monomeric actin and thus eliminates actin filaments due to their innate turnover), was reduced in the strong expressor lines, and both the speed, by which the wave of labelled auxin moved through the coleoptile segments was reduced as well as the steady-state velocity of transport in the strong talin-YFP overexpressors.
These observations linked the amplitude of talin overexpression with the physiological effect of actin bundling. When actin-bundling was induced in the wild type by phalloidin (mimicking the situation in the overexpressor line), this reduced actin dynamicity and auxin transport as well. These correlations demonstrated that a debundled configuration of actin is necessary for efficient responses to auxin including elongation growth, gravitropic bending and polar transport of auxin. Similar to the mT-BY2 cell line, addition of exogenous IAA or NAA (but 2,4-D) could restore both a debundled actin configuration and efficient longitudinal auxin transport in the strong talin expressors and with the same time course. Moreover, gravitropic curvature as physiological response dependent on polar auxin transport was recovered as well. Thus, the phenotype of the strong talin expressors was completely rescued by debundling of actin in response to exogenous IAA. Therefore, in these lines, a debundled configuration of actin is sufficient for efficient responses to auxin.
Thus, F-actin debundling was found to be both necessary and sufficient for auxin-induced stimulation of auxin transport, it followed the same time course, and it could be triggered by IAA and NAA, but not by 2,4-D. The most straightforward model to explain these findings is a causal relationship between auxin-induced debundling of F-actin and auxin-induced stimulation of auxin transport. By inducing a dissociation of F-actin bundles into finer strands, auxin might release auxin-efflux carriers such as the PIN proteins from sequestration in intracellular compartments such that these carriers can be delivered to their site of action at the plasma membrane ().
The implications of this model are to be explored, but already at this stage it can be used to derive characteristic properties of basipetal auxin transport. For instance, the model predicts that the transport of IAA should oscillate. Auxin will induce fine F-actin strands that will partition auxin-efflux carriers more efficiently to the plasma membrane, sucht that the intracellular auxin concentration will decrease. This decrease will cause F-actin bundling and, as a consequence, efflux carriers will be sequestered in intracellular compartments, culminating in a reduced efflux such that auxin received from the adjacent cells will accumulate and trigger a new cycle. The frequency of these oscillations should depend on the dynamics of actin reorganisation (around 20 min), and the speed of PIN cycling (in the range of 5–10 min, 10) and is expected to be in the range of 25–30 min. In fact, such oscillations with a period of 25 min have been actually observed during classical experiments on basipetal auxin transport in coleoptiles.Citation38
We therefore arrive at a model of a self-referring regulatory circuit between polar auxin transport and actin organization, where auxin promotes its own transport by shaping actin filaments. This circuit seems to contribute to the self-amplification of auxin transport that is a central element in current models of auxin-dependent patterning.
Outlook: Manipulation of Intracellular Auxin Gradients with Caged Auxin
To probe the actin-auxin oscillator further, it is necessary to control auxin gradients at higher spatial resolution, desirably even across a single cell. To achieve this goal we used an approach based on caged auxin that can be released by localized irradiation in single cells or even parts of a cell.Citation39 Caged compounds are conventionally based on 2-nitrobenzyl-esters as caging group. However, the ester-bond was found to be enzymatically hydrolyzed in plant cells such that auxin was released prior to photolysis producing high unspecific background activities. Therefore, an esterase-resistant caging group was designed based on molecular modeling of the active sites in the responsible esterases, and this eliminated background release in the dark as probed by treatment of Arabidopis roots that had been transformed with the auxin-responsive DR5-promotor driving GUS. Moreover, root elongation as rapid and characteristic auxin-response could be manipulated by irradiation demonstrating physiological activity of the uncaged auxin.
In the next step, we examined, whether auxin gradients can be manipulated at the level of individual cells by this approach. For this purpose, we used tobacco BY-2 cells expressing GFP as reporter driven by the DR5 promoter. To demonstrate release of caged auxin, bicellular files were selected, where the fluorescence intensity of the reporter was equal in both cells. Then, IAA was released from its caged precursor in the tip of one cell by microirradiation with UV-light and fluorescence intensity followed over time. The fluorescence intensity in the cytoplasm of the irradiated cell increased continuously, whereas the cytoplasmic fluorescence in the adjacent cell did not change.
To test, whether this auxin gradient could trigger gradients of auxin-dependent physiological responses, we again used auxin-induced debundling of actin as reporter using again the BY-2 line overexpressing YFP-talin.Citation34 Caged IAA was loaded into the transgenic cells, and one cell of the bicellular file was irradiated with a microbeam of UV light. F-actin bundles reorganized into fine F-actin strands in the irradiated cell, but not in the neighbouring cell demonstrating that the physiological response to auxin truly reported the cell-to-cell gradient of auxin release. Thus, for the first time in a physiological context, these modified caged auxins open the exciting possibility of studying polar auxin transport on the cellular level. In the next step, this tool will be used to impose specific transcellular gradients of the patterning signal and even to probe actin organization in the context of intracellular gradients of auxin.
Abbreviations
2,4-D, 2,4 | = | dichlorophenoxy-acetic acid |
ARF, ADP | = | ribosylation factors |
ARP3 | = | actin-related protein 3 |
AUX1 | = | auxin influx carrier |
BFA | = | brefeldin A |
NPA | = | naphthylphthalamic acid |
PIN | = | pin-formed auxin-efflux facilitators |
TIBA | = | tri-iodobenzoic acid |
YFP | = | yellow fluorescent protein |
Figures and Tables
Figure 1 Model of the actin-auxin oscillator. In the absence of auxin, actin is organized in bundles that do not support the localization of auxin-efflux carriers in the plasma membrane, such that these carriers are clustered inside the cell. Upon addition of IAA or NAA (but not 2,4-D that is perceived by a different receptor), these bundles detach into fine actin strands that efficiently support the polar localization of the auxin-efflux carriers. The altered auxin efflux will transiently reduce the intracellular concentration of auxin (which will be later compensated by auxin drainage from neighboring cells) such that actin returns to a more bundled configuration. This will again affect the localization and thus the activity of efflux-carriers such that intracellular auxin concentration increases again. Since the cycling of transporters is a rapid process, the frequency of the oscillator is mainly determined by the velocity of actin reorganization (in the range of around 20 min).
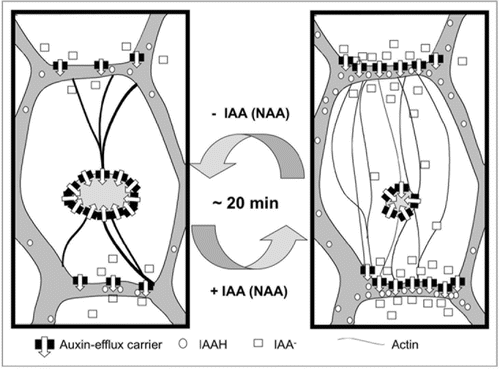
References
- Zimmermann W. Die Telomtheorie 2000; Stuttgart Gustav Fischer
- Nick P. Baluška F, Mancuso S. Auxin and the Communication between Plant Cells. Signaling in Plants 2009; Heidelberg Springer 1 - 27
- Lomax TL, Muday GK, Rubery PH. Davies PJ. Auxin transport. Plant Hormones: Physiology, Biochemistry and Molecular Biology 1995; Dordrecht Kluwer 509 - 530
- Nick P. Noise yields Order—Auxin, actin and polar patterning. Plant Biol 2006; 8:360 - 370
- Sachs T. Integrating cellular and organismic aspects of vascular differentiation. Plant Cell Physiol 2000; 41:649 - 656
- Reinhard D, Mandel T, Kuhlemeier C. Auxin regulates the initiation and radial position of plant lateral organs. Plant Cell 2000; 12:507 - 518
- Chen JG. Dual auxin signalling pathways control cell elongation and division. J Plant Growth Regul 2000; 20:255 - 264
- Petrášek J, Mravec J, Bouchard R, Blakeslee JJ, Abas M, Seifertová D, et al. PIN proteins perform a rate-limiting function in cellular auxin efflux. Science 2006; 312:914 - 918
- Steinmann T, Geldner N, Grebe M, Mangold S, Jackson CL, Paris S, et al. Coordinated polar localization of auxin efflux carrier PIN1 by GNOMARF GEF. Science 1999; 286:316 - 318
- Geldner N, Friml J, Stierhof YD, Jürgens G, Palme K. Auxin transport inhibitors block PIN1 cycling and vesicle trafficking. Nature 2001; 413:425 - 428
- Geldner N, Anders N, Wolters H, Keicher J, Kronberger W, Müller P, et al. The Arabidopsis GNOM ARF-GEF mediates endosomal recycling, auxin transport and auxin-dependent plant growth. Cell 2003; 112:219 - 230
- Dhonukshe P, Aniento F, Hwang I, Robinson DG, Mravec J, Stierhof YD, et al. Clathrin-mediated constitutive endocytosis of PIN auxin efflux carriers in Arabidopsis. Current Biol 2007; 17:520 - 527
- Friml J, Wišniewska J, Benková E, Mendgen K, Palme K. Lateral relocation of auxin efflux regulator PIN3 mediates tropism in Arabidopsis. Nature 2002; 415:06 - 09
- Kleine-Vehn J, Dhonukshe P, Swarup R, Bennett M, Friml J. Subcellular trafficking of the Arabidopsis auxin influx carrier AUX1 uses a novel pathway distinct from PIN1. Plant Cell 2006; 18:3171 - 3181
- Sun H, Basu S, Brady SR, Luciano RL, Muday GK. Interactions between auxin transport and actin cytoskeleton in developmental polarity of Fucus distichus embryos in response to light and gravity. Plant Physiol 2004; 135:266 - 278
- Paciorek T, Zažimalová E, Ruthardt N, Petrášek J, Stierhof YD, Kleine-Vehn J, et al. Auxin inhibits endocytosis and promotes its own efflux from cells. Nature 2005; 435:1251 - 1256
- Blakeslee JJ, Peer WA, Murphy AS. Auxin transport. Curr Opin Plant Biol 2005; 8:494 - 500
- Muday GK, Murphy AS. An emerging model of auxin transport regulation. Plant Cell 2002; 14:293 - 299
- Rahman A, Bannigan A, Sulaman W, Pechter P, Blancaflor EB, Baskin TI. Auxin, actin and growth of the Arabidopsis thaliana primary root. Plant J 2007; 50:514 - 528
- Dhonukshe P, Grigoriev I, Fischer R, Tominaga M, Robinson DG, Hašek J, et al. Auxin transport inhibitors impair vesicle motility and actin cytoskeleton dynamics in diverse eukaryotes. Proc Natl Acad Sci USA 2008; 105:4489 - 4494
- Leopold AC. Ruhland W. The transport of auxin. Encyclopedia of Plant Physiology 1961; 14:Berlin Springer 671 - 682
- Nagai R, Rebhun LI. Cytoplasmic microfilaments in streaming Nitella cells. J Ultrastruct Res 1966; 14:571 - 589
- Waller F, Nick P. Response of actin microfilaments during phytochrome-controlled growth of maize seedlings. Protoplasma 1997; 200:154 - 162
- Kutschera U, Bergfeld R, Schopfer P. Cooperation of epidermal and inner tissues in auxin-mediated growth of maize coleoptiles. Planta 1987; 170:168 - 180
- Waller F, Riemann M, Nick P. A role for actin-driven secretion in auxin-induced growth. Protoplasma 2002; 219:72 - 81
- Holweg C, Süßlin C, Nick P. Capturing in-vivo dynamics of the actin cytoskeleton. Plant Cell Physiol 2004; 45:855 - 863
- Nick P, Han M, An G. Auxin stimulates its own transport by actin reorganization. Plant Physiology 2009; 151:155 - 167
- Ullah H, Chen JG, Temple B, Boyes DC, Alonso JM, Keith RD, et al. The β-subunit of the Arabidopsis G protein negatively regulates auxin-induced cell division and affects multiple developmental processes. Plant Cell 2003; 15:393 - 409
- Campanoni P, Nick P. Auxin-dependent cell division and cell elongation: NAA and 2,4-D activate different pathways. Plant Physiol 2005; 137:939 - 948
- Nagata T, Nemoto Y, Hasezava S. Tobacco BY-2 cell line as the “Hela” cell in the cell biology of higher plants. Int Rev Cytol 1992; 132:1 - 30
- Nagata T, Kumagai F. Plant cell biology through the window of the highly synchronized tobacco BY-2 cell line. Methods Cell Sci 1999; 21:123 - 127
- Redig P, Shaul O, Inze D, Van Montagu M, Van Onckelen H. Levels of endogenous cytokinins, indole-3-acetic acid and abscisic acid during the cell cycle of synchronized tobacco BY-2 cells. FEBS Letts 1996; 391:175 - 180
- Campanoni P, Blasius B, Nick P. Auxin transport synchronizes the pattern of cell division in a tobacco cell line. Plant Physiol 2003; 133:1251 - 1260
- Maisch J, Nick P. Actin is involved in auxin-dependent patterning. Plant Physiol 2007; 143:1695 - 1704
- Stals H, Inzé D. When plant cells decide to divide. Trends Plant Sci 2001; 6:359 - 364
- Ketelaar T, Anthony RG, Hussey PJ. Green fluorescent protein-mTalin causes defects in actin organization and cell expansion in Arabidopsis and inhibits actin depolymerizing factor’s actin depolymerizing activity in vitro. Plant Physiol 2004; 136:3990 - 3998
- Maisch J, Fišerová J, Fischer L, Nick P. Actin-related protein 3 labels actin-nucleating sites in tobacco BY-2 cells. J Exp Bot 2009; 60:603 - 614
- Hertel R, Flory R. Auxin movement in corn coleoptiles. Planta 1968; 82:123 - 144
- Kusaka N, Maisch J, Nick P, Hayashi KI, Nozaki H. Manipulation of intercellular auxin in a single cell by light with esterase-resistant caged auxins. ChemBioChem 2009; 10:2195 - 2202