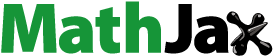
Abstract
Plants respond to almost any kind of external stimulus with transients in their cytoplasmic free calcium concentration ([Ca2+]c). A huge variety of kinetics recorded by optical techniques has been reported in the past. This variety has been credited the specificity needed to explain how information about incoming stimuli is evaluated by the organism and turned into the right physiological responses which provide advantages for survival and reproduction. A physiological response often takes place away from the site of stimulation. This requires cell-to-cell communication. Hence, responding cells are not necessarily directly stimulated but rather receive an indirect stimulus via cell-to-cell communication. It appears unlikely that the '[Ca2+]c signature' in the primarily stimulated cell is conveyed over long distances via cell-to-cell communication from the 'receptor cells' to the 'effector cells'. Here, a novel aspect is highlighted to explain the variety of [Ca2+] kinetics seen by integrating methods of [Ca2+]c recording. Plants can generally be seen as cellular automata with specific morphology and capable for cell-to-cell communication. Just a few rules are needed to demonstrate how waves of [Ca2+]c-increases percolate through the organism and thereby deliver a broad variety of 'signatures'. Modeling intercellular signaling may be a possible way to find explanations for different kinds of signal transmission, signal amplification, wave formation, oscillations and stimulus-response coupling. The basic examples presented here show that care has to be taken when interpreting cellular '[Ca2+]c signatures' recorded by optical techniques which integrate over a big number of cells or even whole plants.
Introduction
In the past three decades the cytoplasmic free calcium [Ca2+]c in plants has been credited with central roles in ion transport, signal perception, signal transduction and physiological response.Citation1,Citation2,Citation3 Changes in cytoplasmic free calcium ([Ca2+]c) occur with any external stimulus, be it biotic or abiotic. It has been hypothesized many times that amplitude and duration of the cellular [Ca2+] signal and its subcellular location may determine what specific subset of calcium dependent proteins (i.e., the ‘calcium receptors’) are responding (i.e., activated or de-activated) on cellular level. “However, the focus on intracelluar mechanisms might have led […] away from physiology”.Citation4 Therefore, a glance at the whole, namely intercellular signalling, may be worthwhile.
Any physiological ‘whole plant’-responses like changes in growth and development, organ movement, metabolic shifts or pathogen defence will need some sort of cell-to-cell communication and systemic spread of signals. This communication is coupled with directed transport of ions,Citation5,Citation6 or phytohormones,Citation7–Citation9 with hydraulic signalsCitation10 or with the release and spread of reactive oxygen species (ROS) or nitrogen monoxide (NO).Citation11 The best studied, the most effective and fastest cell-to-cell communication in plants is electrical signalling, in vascular plants, via vascular tissue.Citation12–Citation14 This in turn is always tightly coupled with transients in [Ca2+]c. Action potentials (APs) and other fast membrane potential transients are accompanied by changes in [Ca2+]c due to the abundance of depolarisation-activated cation channels.
Electrical signals on cellular level can spread over tissues, organs and the whole organism and thereby transport information and elicit responses at locations far away from the point of initial stimulation. Most prominent examples are Mimosa pudica and the venus fly trap Dionaea muscipula.Citation15,Citation16 All plants can propagate and spread signals via cell-to-cell communication. In particular electrical signalling has been observed in many diverse plant species from macroalgae like Chara to vascular plants like Arabidopsis, Tobacco and maize and even shrubs and trees like willow and poplar.Citation12,Citation13,Citation17,Citation18
Different optical techniques were developed to measure and image [Ca2+]c in plants.Citation19 On cellular level fluorescence techniques using synthetic Ca2+ dyes like Indo or FuraCitation20,Citation21 or recombinant indicators like pericams or cameleonsCitation22 are most frequently used. Luminescence techniques using recombinant photoproteins like aequorin and obelin are used to measure [Ca2+]c more globally, on whole organ or whole plant level.Citation23 The latter technique has been used to also demonstrate the migration (percolation) of the [Ca2+]c-signal through organs.Citation24,Citation25 All these optical techniques showed that each stimulus elicits a specific [Ca2+]c response (e.g., reference Citation26).
The big diversity of [Ca2+]c responses recorded on cellular and on whole-plant level led to the so-called ‘signature hypothesis’.Citation27–Citation29 This hypothesis has been questionedCitation3,Citation30 and despite well-founded doubts it is still conveyed.Citation31
Here, another aspect is highlighted to explain the broad variety and complexity of [Ca2+]c kinetics seen in intact higher plants when measured by means of integrating optical techniques:
For simplicity reasons just a few general assumptions (rules) are made to model plants as conglomerations of cells with a competence for cell-to-cell communication. Each cell can respond to an external stimulus, giving a typical [Ca2+]c signal and can hand down this signal to neighboring cells, leading to signal percolation and spreading throughout the organism. This delivers a possible answer to the question: How does local and specific stimulation determine the overall [Ca2+]c response recorded from a whole plant?
Assumptions
#1. The kinetic of [Ca2+]c in a single cell after stimulation or excitation is well defined.
#2. A cell is either in an excited state or not (binary state behavior).
#3. A cell switches into an excited state when it is stimulated or when one of its neighboring cells has switched into an excited state.
#4. A cell is not excitable (i.e., in a refractory period) as long as it is still excited.
Notes
Assumption #1 means that a single-cell [Ca2+]c kinetic elicited by a certain stimulus can be described by a sum of exponentials (example EquationEq. 1, ). Thereby, the kinetic is quantified by a minimum of five independent free parameters (Ca0, a1, a2, τE, τR).
Assumption #2 means that an excited state is defined by the cytoplasmic calcium concentration which exceeds a certain (excitation-) level, E-level = [Ca2+]E. Cells with [Ca2+]c below [Ca2+]E are called ‘not excited’.
Assumption #3 means that a stimulus received from a cell is transmitted to its neighboring cells. The speed of this transmission depends on the kinetics of the [Ca2+]c increase i.e., the time constant τE (see EquationEq. 1) and on [Ca2+]E ().
Assumption #4 means that a cell is not excitable as long as its [Ca2+]c level is still not below [Ca2+]E. This determines a refractory period (mainly given by τR) which is needed to form a wave of excitation.
Cellular excitation and the single-cell calcium response.
A stimulated cell may undergo a rapid change in membrane potential which is sometimes called “action potential” (AP) or “transient potential” (TP). This transient is accompanied by a [Ca2+]c spike with a fast rise and a slow recovery of [Ca2+]c to its previous level. The kinetics of the AP and of the [Ca2+]c changes however are not necessarily identical (Thiel et al. 1997).
In a first approach a [Ca2+]c spike is described by two exponentials (EquationEq. 1). One of which (τE) determines the rise in [Ca2+]c and the other (τR) its recovery back to steady state ():
Equation 1.
Results
Cell-to-cell communication and signal percolation.
The two-cell system. A cellular excitation can be transmitted to neighboring cells (assumption #3). With a simple two cell system this means that a [Ca2+]c signal elicited by a stimulus in one cell is received by the other one. This event is shown in . When the [Ca2+]c in the stimulated cell (black trace) exceeds a certain level [Ca2+]E (i.e., ≈400 nM; red line) then the neighboring cell switches into the excited state as well (blue trace). The time Δt (i.e., ≈5) between the stimulus of the first cell (at t = 50) and the response in the second cell is defined by [Ca2+]E, the amplitude of the [Ca2+]c spike and the time constant τE (EquationEq. 1). The observed [Ca2+]c transient of the whole system then is the mean of the two individual [Ca2+]c traces. The velocity V of signal transmission from cell to cell (wavefront) through the tissue is then given by Δt and the dimension (r) of the cell (EquationEq. 2
):
Equation 2.
The four-cell system. shows the responses in the 4-cell system when a border cell is stimulated. The overall signal received ( bold trace) does not differ very much from that of a single cell ( gray trace). The main difference is in the raising part of the curve. The whole system seems to response more slowly.
The sixteen-cell system. The model can be expanded to a system with an increased number of individual cells. As a next step a 16-cell system is chosen as this is still easy to study. Here, the fact becomes obvious that the shape of the system and the location of the stimulus determine the overall signal. gives the compact version (4 × 4 cell system) with excitation in the middle of the system. , in contrast, shows the system where the exciting stimulus is transmitted like through a chain of dominos. The overall response of the 16-cell system in the ‘domino-configuration’ ( green trace) is remarkably different from of a single cell response (gray trace).
From here on it is easy to imagine that finally with increasing cell numbers a stimulus will cause independent [Ca2+]c-waves propagating from the location of stimulation onwards through the whole plant.
The 225-cell system. Consequently, a much bigger number of cells allows to study responses from systems with different configurations:
The compact configuration. A configuration like that shown in with 225 cells (i.e., a compact 15 × 15-square) would lead to a linear chain reaction (, green trace).
The ‘domino’-configuration. If we have 225 cells in the domino configuration analogous to then a wave-front of excited states would propagate through the system after the first cell has been stimulated. In the overall signal ( and blue trace) this would result in a constantly elevated [Ca2+]c level as long as the wave is still propagating through the system. Now, this response gives no clue anymore on what happens on cellular level (compare blue trace with gray trace in ).
The avalanche configuration. In an avalanche configuration it is assumed that every excited cell stimulates two neighboring cells. Thus the number of excited cells is exponentially increasing (avalanche reaction). Even here (, red trace), the system response is clearly different from that when all cells are responding simultaneously (gray trace).
225 cells in the ‘young-seedling’ configuration. An avalanche configuration as mentioned above would require a fractal morphology. This is realized in only few plant species such as ferns (e.g., male fern Dryopteris filix-mas). Thus, this example remains more academic.
However, the 225 cells in can be arranged in a pattern which is similar to that of a seedling (). Here four different events are demonstrated () just to see what happens when the seedling is stimulated in four different ways:
All cells of the left cotyledon are stimulated simultaneously ()
‘Epidermal’ cells of both cotyledons are stimulated simultaneously ()
Two meristematic cells are stimulated ()
The root tip only is stimulated ()
The different kinetics resulting from the different events are shown in .
When the root tip is excited, the overall signal starts to climb up the root resulting in a domino effect (compare at 100 < t < 150 with blue trace), then, in the hypocotyl, an avalanche effect occurs (compare at 150 < t < 250 with red trace) amplifying the signal and resulting in an additional bump at t = 220 before the signal spreads into the coteledons and ceases (at t > 300). So, even biphasic responses—not seen in the single-cell signal ()—occur. Thus, the recorded overall signal is dependent on the configuration of the system and on the site of initial stimulation.
Discussion
Based on the assumption that (electrical) cell-to-cell communication is accompanied by a cell-to-cell percolation of [Ca2+]c signals and vice versa, a basic model has been developed here that mimics the overall [Ca2+]c response in whole plants such as recorded by a luminometer from luminescent aequorin-expressing plants or by brightfield fluorescence ratios from whole plants or organs expressing calcium-specific probes. On the one hand, the above model shows that the whole-system-response does not differ significantly from the single-cell-response (–) when
the system is small (few cells only),
the system is compact (no tendrills, no long twigs, no long roots),
many cells are excited simultaneously,
the ‘percolation-resistance’ (i.e., [Ca2+]E) is low, and thus the percolation velocity is high compared to the [Ca2+]c-kinetics in a single cell response.
On the other hand, however, the overall signal is very different from the single-cell signal, when
there is a big number of cells, and
the morphology is elongated, branched or dendritic,
few cells only are excited (the stimulus—such as wounding—is local),
the percolation resistance ([Ca2+]E) is high.
Thus, it becomes obvious that in some cases a kinetic analysis of a whole-system-signal will not allow conclusions on the response (the ‘signature’) in a single cell. And vice versa: the single cell response does not provide clues about the whole system's response. If the primary stimulus defines the signature of the cellular [Ca2+]c signal then this signature does not necessarily appear in the neigbouring ‘secondary’ cells which receive the message via cell-to-cell communication. In fact, several parameters, like shape/morphology of the plant, the location of the primary stimulus or the primary receptor, and the kind of cell-to-cell interaction have to be considered, before specific conclusions can be drawn.
Here only two-dimensional objects have been modelled for convenience. But, it is easy to deduce from the above examples that the kinetics of a recorded overall [Ca2+]c signal become even more complicated when
the system is a three-dimensional object,
several cells at different ends of the system are excited,
different stimuli impact on the system,
endogenous [Ca2+]c elevations appear due to developmental, diurnal or general metabolic changes,
there are obstacles and high ‘percolation resistances’ in the system such as electrically isolated cells, cells lacking plasmodesmata, Caspary-strips, dead cells or air-filled intercellular spaces, so that signal percolation has to make a detour, or stands still,
there is tissue with long prosenchymatic cells—such as vascular strands—specialized for accelerated signal transmission.
In particular the latter points (5 and 6) show that in complex biological systems like plants, signal events may form their own routes depending on the underlying signal transmission mode.
The models presented here are no ‘cellular automata’ by definition [for exact definition see WolframCitation32 and his website (www.stephenwolfram.com/publications/books/ca-reprint/)] since the systems discussed here are very limited in their number of cells. However, ‘cellular automata’ in a broader sense may be favourable tools for modelling complexity, self organisation and even nonlinear dynamics.Citation33–Citation35
The examples presented here are not based on assumptions about the time scale and the molecular mechanisms underlying cell-to-cell communication. Therefore, they are open for further refinements in order to simulate observed events and concrete mechanisms.
Material and Methods
The models have been developed as Excel spreadsheets using Micosoft-Excel 2002. The Excel files can be obtained from the author on request. (www.zbm.uni-kiel.de/agplieth/).
Conclusions and Perspectives
The signature of a global [Ca2+]c signal is dependent on, and distorted by so many parameters that care has to be taken when interpreting signals recorded by techniques which integrate over a number of cells or even whole plants.
Modelling intercellular signalling may be a possible way to find explanations for different kinds of signal transmission, signal amplification, wave formation and oscillations. Any attempt of ‘modelling complexity’ can lead to novel insights and the ability to decide whether the cellular response or the systemic (percolated) response is decisive for the final physiological response under study.
Abbreviations
[Ca2+]c | = | cytoplasmic free calcium concentration |
ROS | = | reactive oxygen species |
NO | = | nitrogen monoxide |
Figures and Tables
Figure 1 A single cell response after stimulation. The cell was stimulated at t = 50. A timescale of 1: = 0.1 sec gives a realistic estimate of the real kinetic in a living plant. The parameters according to EquationEq. 1 for this [Ca2+]c-spike are: .
![Figure 1 A single cell response after stimulation. The cell was stimulated at t = 50. A timescale of 1: = 0.1 sec gives a realistic estimate of the real kinetic in a living plant. The parameters according to EquationEq. 1[Ca2+]c(t)=[Ca2+]c0+a1⋅(1−exp(−tτE))+a2⋅(1−exp(−tτR)) for this [Ca2+]c-spike are: Table 1.](/cms/asset/ed9c48af-ec68-4876-a392-7dcf7bf9618c/kpsb_a_10910717_f0001.gif)
Figure 2 The signal percolation in a two cell system. (A) The response in the second cell (blue trace) occurs when the [Ca2+]c in the stimulated cell exceeds half maximum (a level of [Ca2+]E = 400 nM). This results in a delay time of Δt = 5. (B) The [Ca2+]c traces of both cells overlap in time. It is obvious that the sum (=overall signal of the two-cell system) does not differ significantly from the single cell response.
![Figure 2 The signal percolation in a two cell system. (A) The response in the second cell (blue trace) occurs when the [Ca2+]c in the stimulated cell exceeds half maximum (a level of [Ca2+]E = 400 nM). This results in a delay time of Δt = 5. (B) The [Ca2+]c traces of both cells overlap in time. It is obvious that the sum (=overall signal of the two-cell system) does not differ significantly from the single cell response.](/cms/asset/ab749350-416d-4098-9c00-7591ab8ec6f1/kpsb_a_10910717_f0002.gif)
Figure 3 A presentation of the four cell system. (A) In a four cell system the configuration can be either a running of the signal from one end through the whole system (left side in A) or from the middle to the borders (right hand side in A). (B) The single responses from the individual cells. (C) The sum of all (bold line) gives the observed signal. It is slightly different from that of a single cell given as gray trace for comparison.
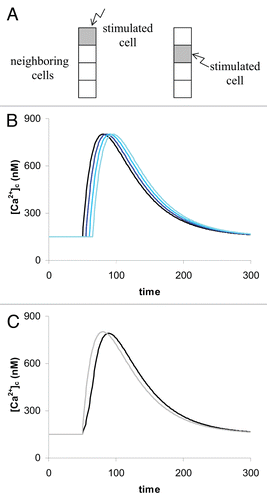
Figure 4 The 16-cells system. (A) The compact system with stimulation in the middle. (B) The elongated system (domino configuration) with stimulation at one end. (C) [Ca2+]c-traces of all 16 cells in the domino configuration. (D) The over-all responses of the whole system in the ‘domino configuration’ (green) and in the compact configuration (pink). This trace is almost the same as in the 4-cell system as shown in . For comparison the response of a single cell is shown (gray line). This is identical with a whole systems response when all cells are simultaneously stimulated.
![Figure 4 The 16-cells system. (A) The compact system with stimulation in the middle. (B) The elongated system (domino configuration) with stimulation at one end. (C) [Ca2+]c-traces of all 16 cells in the domino configuration. (D) The over-all responses of the whole system in the ‘domino configuration’ (green) and in the compact configuration (pink). This trace is almost the same as in the 4-cell system as shown in Figure 3C. For comparison the response of a single cell is shown (gray line). This is identical with a whole systems response when all cells are simultaneously stimulated.](/cms/asset/bb5c6e72-434c-4a76-a093-6c8e12e44212/kpsb_a_10910717_f0004.gif)
Figure 5 The 225 cells system. (A) The compact 225 cell configuration (15 × 15 cells). (B) The responses of a 225-cell system in the ‘domino’ configuration (blue), in the compact configuration (green), in the ‘avalanche’-configuration (red). The gray trace represents the response when all cells are responding simultaneously (dotted line) and is identical with the response of a single cell. [Ca2+]E was here set to 310 nM which results in a delay time of Δt = 3. All other model parameters are the same as in . The inset is a close-up and represents the modelled data between t = 40 and 240.
![Figure 5 The 225 cells system. (A) The compact 225 cell configuration (15 × 15 cells). (B) The responses of a 225-cell system in the ‘domino’ configuration (blue), in the compact configuration (green), in the ‘avalanche’-configuration (red). The gray trace represents the response when all cells are responding simultaneously (dotted line) and is identical with the response of a single cell. [Ca2+]E was here set to 310 nM which results in a delay time of Δt = 3. All other model parameters are the same as in Figure 1. The inset is a close-up and represents the modelled data between t = 40 and 240.](/cms/asset/696a1040-d9ed-42be-8b10-4480f469f85c/kpsb_a_10910717_f0005.gif)
Figure 6 Dependence of the overall [Ca2+]c kinetic on the location of the primary stimulus and on the number of primarily stimulated cells in the ‘young-seedling configuration’. (A) All cells of the left cotyledon are stimulated simultaneously; (B) The surface cells of both cotyledons are stimulated; (C) Two meristematic cells are stimulated; (D) The root tip only is stimulated. (E) Dependent on the site of stimulation and the number of primarily stimulated cells, very different [Ca2+]c kinetics are obtained from the whole system.
![Figure 6 Dependence of the overall [Ca2+]c kinetic on the location of the primary stimulus and on the number of primarily stimulated cells in the ‘young-seedling configuration’. (A) All cells of the left cotyledon are stimulated simultaneously; (B) The surface cells of both cotyledons are stimulated; (C) Two meristematic cells are stimulated; (D) The root tip only is stimulated. (E) Dependent on the site of stimulation and the number of primarily stimulated cells, very different [Ca2+]c kinetics are obtained from the whole system.](/cms/asset/9a23ffe7-e0e0-41e4-914f-bf79c81973e9/kpsb_a_10910717_f0006.gif)
Table 1 Model parameters used according to EquationEquation 1 for calculating curves in to
Acknowledgements
I thank Hartmut Kaiser (Botanical Institute, University Kiel) for critically reading the manuscript. The author received financial support from the DFG and the federal state of Schleswig-Holstein.
References
- Sanders D, Pelloux J, Brownlee C, Harper JF. Calcium at the crossroads of signaling. Plant Cell 2002; 14:401 - 417
- White PJ, Broadley MR. Calcium in plants. Ann Bot 2003; 92:487 - 511
- Plieth C. Calcium: Just another regulator in the machinery of life?. Ann Bot 2005; 96:1 - 8
- Røttingen J, JG I. Ruled by waves? Intracellular and intercellular calcium signalling. Acta Physiologica Scandinavica 2000; 169:203 - 219
- Sibaoka T. Transmission of action potential in Biophytum. Botanical Magazine Tokyo 1973; 86:51
- Tabata T, Sibaoka T. Conduction velocity and blockage of action potential in Chara internodal cells. Plant Cell Physiol 1987; 28:1187 - 1194
- Haberer G, Kieber JJ. Cytokinins. New Insights into a Classic Phytohormone 2002; 354 - 362
- Baluska F, Barlow PW, Baskin TI, Chen R, Feldman L, Forde BG, et al. What is apical and what is basal in plant root development?. Trends Plant Sci 2005; 10:409 - 411
- Shabala S, Pang J, Zhou M, Shabala L, Cuin TA, Nick P, Wegner LH. Electrical signalling and cytokinins mediate effects of light and root cutting on ion uptake in intact plants. Plant Cell Environ 2009; 32:194 - 207
- Christmann A, Weiler EW, Steudle E, Grill E. A hydraulic signal in root-to-shoot signalling of water shortage. Plant J 2007; 52:167 - 174
- Baluska F, Volkmann D, Menzel D. Plant synapses: actin-based domains for cell-to-cell communication. Trends Plant Sci 2005; 10:106 - 111
- Grams TE, Lautner S, Felle HH, Matyssek R, Fromm J. Heat-induced electrical signals affect cytoplasmic and apoplastic pH as well as photosynthesis during propagation through the maize leaf. Plant Cell Environ 2009; 32:319 - 326
- Fromm J, Lautner S. Electrical signals and their physiological significance in plants. Plant Cell Environ 2007; 30:249 - 257
- Yan X, Wang Z, Huang L, Wang C, Hou R, Xu Z, Qiao X. Research progress on electrical signals in higher plants. Prog Nat Sci 2009; 19:531 - 541
- Forterre Y, Skotheim JM, Dumais J, Mahadevan L. How the Venus flytrap snaps. Nature 2005; 433:421 - 425
- Hodick D, Sievers A. On the mechanism of trap closure of Venus flytrap (Dionaea muscipula Ellis). Planta 1989; 179:32 - 42
- Fromm J, Spanswick R. Characteristics of action potential in willow (Salix viminalis L). J Exp Bot 1993; 44:1119 - 1125
- Fromm J, Bauer T. Action potentials in maize sieve tubes change phloem translocation. J Exp Bot 1994; 45:463 - 469
- Fricker MD, Parsons A, Tlalka M, Blancaflor E, Gilroy S, Meyer A, Plieth C. Fluorescent probes for living plant cells. Plant Cell Biology: A Practical Approach—2nd Ed 2001; 35 - 84
- McAinsh MR, Brownlee C, Hetherington AM. Visualizing changes in cytosolic-free Ca2+ during the response of stomatal guard cells to abscisic acid. Plant Cell 1992; 4:1113 - 1122
- Plieth C, Sattelmacher B, Hansen UP, Thiel G. The action potential in Chara: Ca2+ release from internal stores visualized by Mn2+-induced quenching of furadextran. Plant J 1998; 13:167 - 175
- Allen GJ, Kwak JM, Chu SP, Llopis J, Tsien RY, Harper JF, Schroeder JI. Cameleon calcium indicator reports cytoplasmic calcium dynamics in Arabidopsis guard cells. Plant J 1999; 19:735 - 747
- Plieth C. Salinas J, Sanchez-Serrano JJ. Aequorin as a reporter gene. “Arabidopsis Protocols” 2007; 323:2nd Edition Humana Press 307 - 327
- Knight MR, Read ND, Campbell AK, Trewavas AJ. Imaging calcium dynamics in living plants using semisynthetic recombinant aequorins. J Cell Biol 1993; 121:83 - 90
- Campbell AK, Trewavas AJ, Knight MR. Calcium imaging shows differential sensitivity to cooling and communication in luminous transgenic plants. Cell Calcium 1996; 19:211 - 218
- Plieth C. Plant calcium signaling and monitoring—pros and cons and recent experimental approaches. Protoplasma 2001; 218:1 - 23
- McAinsh MR, Brownlee C, Hetherington AM. Calcium ions as secondary messengers in guard cell signal transduction. Physiologia Plantarum 1997; 100:16 - 29
- McAinsh MR, Hetherington AM. Encoding specificity in Ca2+ signalling systems. Trends Plant Sci 1998; 3:32 - 36
- Ng C-Y, McAinsh MR. Encoding specificity in plant calcium signalling: hot-spotting the ups and downs and waves. Ann Bot 2003; 92:477 - 485
- Scrase-Field S, Knight MR. Calcium: just a chemical switch?. Curr Opin Plant Biol 2003; 6:500 - 506
- McAinsh MR, Pittman JK. Shaping the calcium signature. New Phytol 2009; 181:275 - 294
- Wolfram S. Cellular Automata and Complexity: Collected Papers 1994;
- Hütt M-T, Lüttge U. Nonlinear Dynamics as a Tool for Modelling in Plant Physiology 2002; 281 - 297
- Markus M, Hess B. Isotropic cellular automaton for modelling excitable media. Nature 1990; 347:56 - 58
- Miramontes O, Sole RV, Goodwin BC. Collective behaviour of random-activated mobile cellular automata. Physica D 1993; 63:145 - 160