Abstract
Alternative splicing of pre-mRNAs greatly contributes to diversity in products generated from a single gene. However, the underlying regulatory mechanisms are poorly understood. In the present study, we describe evolutionarily conserved intra-intronic and inter-intronic RNA secondary structures. Mutation experiments revealed that intra-intronic RNA secondary structure causes steric hindrance to enforce mutual splicing exclusivity, while inter-intronic RNA pairing largely functions through a looped-out mechanism. Moreover, mutually exclusive splicing may be regulated by RNA pairing competition between intra- and inter-introns. Importantly, the resulting dynamic RNA architecture largely controls mutually exclusive splicing, although cis-acting regulatory sequences may fine-tune this process. Our results emphasize the importance of dynamic RNA architecture in alternative splicing.
Introduction
Alternative splicing is an important mechanism for the expansion of proteomic and functional diversity.Citation1,Citation2Ever-increasing new alternative transcripts have been detected because of high-throughput sequencing advances.Citation3 In humans, up to 95% of primary transcripts are estimated to undergo alternative splicing.Citation4,Citation5 Mutually exclusive splicing is a strictly regulated form of alternative splicing, where the splicing machinery must choose only one out of two or more candidate exons.Citation6 Several trans-acting factors and multiple cis-elements have been demonstrated to regulate the mutually exclusive exons in cultured insect and mammalian cells.Citation7-Citation16 Several mechanisms have been proposed to direct mutually exclusive splicing, including steric interference,Citation17 spliceosome incompatibility,Citation18 nonsense-mediated decay,Citation6 and competing RNA secondary structures.Citation19-Citation22 However, the underlying regulatory mechanisms in large part remain poorly understood.
The 14–3-3 proteins have been implicated in a number of different signal transduction processes in many cell types.Citation23 The leonardo gene encodes the Drosophila (D) homolog of the zeta isoform of the 14–3-3 protein family,Citation24 which is preferentially expressed in adult mushroom bodies, centers of insect learning and memory.Citation25,Citation26 Three mutually exclusive exons are present in the 14–3-3ζ genes from D. melanogaster and Apis mellifera, while only two copies exist in Bombyx mori and Tribolium castaneum.Citation22 Transgenic rescue analyses have indicated that these splice variants are required for Drosophila embryonic viability.Citation25,Citation26 Recently, we experimentally confirmed competing RNA secondary structures that direct mutually exclusive splicing of 14–3-3 ζ pre-mRNA. A combinatorial mechanism of these inter-intronic RNA pairings was revealed that synergistically ensures selection of only one of multiple exons, through a combination of approximation-activation and looped-out repression in competition by RNA pairing.Citation22
In this study, we reveal physical competition between intra- and inter-intronic RNA pairings. Combining Lepidoptera-wide analyses of alternative splicing patterns with mutational experiments, we found a correlation between the dynamic competition for intra- and inter-intronic RNA pairings and the ratio of exon 5a/5b. Intra-intronic RNA pairing caused steric hindrance between splice sites to ensure mutually exclusive splicing, while inter-intronic RNA pairing functioned by looping-out of exon 5b. Thus, the highly structured pre-mRNA within the exon cluster possibly represents an additional way of regulating mutually exclusive splicing.
Results
Splicing of exons 5a to 5b is blocked by an RNA hairpin
Disrupting and compensatory mutations previously demonstrated that mutually exclusive splicing of exon 5 was regulated by inter-intronic IE1-to-IEa RNA pairing in the silkworm.Citation22 Interestingly, a strong RT-PCR (reverse transcription polymerase chain reaction) product larger than that containing only a single exon 5 variant was detected when using the IE1 mutation construct but not in the wild-type construct (). Further cloning and sequencing of these products revealed that they are derived from RNAs containing exon 5a and exon 5b variants spliced together. Unexpectedly, we failed to detect this abundant RT-PCR product containing exon 5a and exon 5b variants spliced together in the IE1 deletion construct (). Nevertheless, further sequencing and exon-specific restriction analysis indicated that exon 5a was markedly reduced in the IE1 deletion compared with wildtype, although deleting IE1 had little effect on exon 5 inclusion (). These results are reminiscent of the steric hindrance mechanism in which the intron separating two alternative exons is too small to be efficiently spliced in mammals and in Drosophila.Citation27,Citation28 This raised the possibility that the intron between exon 5a and exon 5b is too small to allow splicing of the IE1 deletion construct.
Figure 1. Splicing of exons 5a to 5b is induced by an IE1 mutation, but not an IE1 deletion in B. mori 14–3-3ζ pre-mRNA. (A) Genomic organization of the B. mori (Bmo) 14–3-3ζ gene. Constitutive exons (in black boxes), alternative exons (in blue boxes), conserved intronic elements IE1 and IE2 (yellow), and the intron (line) are shown. Docking sites (marked by saddle shapes) are reverse-complementary to an upstream selector sequence (marked by hearts). (B) Effects of mutations on exon 5a and exon 5b inclusion. (C) Effects of mutations on exon 5a and exon 5b selection. The specific RT-PCR band containing exon 5a or exon 5b was purified for digestion using an exon-specific restriction enzyme. (D). Schematic diagrams of the mutant constructs that test for splicing. A series of constructs were made, with IE1 replaced by inserts of 6, 13, 18, 24, 31, and 35 nt. (E). Effects of inserts on exon 5 splicing. (F). Schematic diagrams of the minigene constructs used to test the importance of the RNA hairpin by increasing the loop sizes. (G). Effect of the enlarged loops on exon 5 selection. Data are expressed as the percentage of the mean ± standard deviation from three independent experiments.
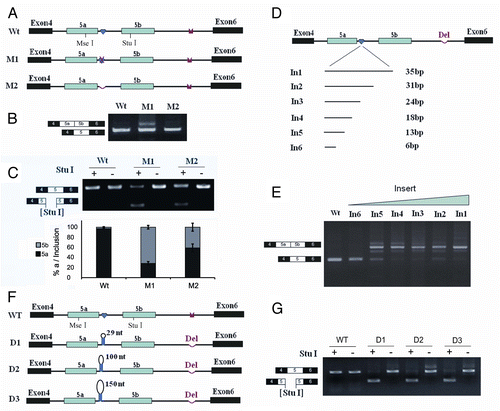
Given that IE1 functions in a sequence-independent manner in mutually exclusive splicing, we hypothesized that RNA secondary structures were formed within the IE1 sequences. Thus, the “effective” distance of introns, if smaller than a lower limit, would prevent exon 5a and exon 5b from being spliced together. To confirm this, we inserted unrelated heterogeneous sequences instead of IE1 sequences to increase the intron size, which we predicted would allow splicing. As expected, abundant RT-PCR products were detected containing exon 5a and exon 5b variants spliced together (). These results demonstrate that the inserts allow splicing of exon 5a to exon 5b.
To determine the minimal spacing required to allow splicing, a series of mutants was then constructed, with the size of the inserts varying between 3 and 6 nucleotides (nt) (). Transfection experiments with these constructs indicated that an insert of 6 nt was inadequate for splicing (). For this construct, the only band detected was the band containing exon 5a or exon 5b. For constructs with inserts of 13 nt or more, both expected splicing products (one exon 5 or both) were observed (). This result indicates that the minimum intron size to allow splicing is around 65 nt. These data are close to the minimal distance of approximately 60 nt previously determined in Drosophila.Citation28 To further determine whether the RNA hairpin acts sterically to interfere with splice sites for exon 5 selection, we made a series of insertions into the loop (). These experiments revealed that increasing the loop size from 29 nt to 100 or 150 nt does not result in a significant increase in RNAs containing exon 5a and exon 5b variants spliced together in the IE2 deletion constructs (). These data indicate that the IE1 sequences involved in formation of the RNA hairpin acted as steric interference for exon 5 selection.
The RNA hairpin is evolutionarily conserved in Lepidoptera
To explore whether this RNA hairpin is evolutionarily conserved in Lepidoptera, genomic DNA sequences spanning the same region studied in silkworm were obtained for 14 species of moths and butterflies. These 14–3-3ζ orthologs contained two duplicated exons, which underwent mutually exclusive splicing. Sequence analyses indicated that this ~40-bp region was nearly conserved between silkworm and other Lepidoptera 14–3-3ζ orthologs (). RNA structural analysis using the MFOLD programCitation29 indicated that the RNA hairpin was evolutionarily conserved across all Lepidopteran species investigated, suggesting that the RNA hairpin was more than 140 million years old.Citation30 The size of the intron between exon 5a and exon 5b was around 90 bp in these orthologs, which is well above the minimal length of 60 nt identified in Drosophila.Citation28 However, if we exclude the stem-loop structure, this effective distance would be only around 50 nt, which is smaller than the intron size limit. An analogous example has been described in the mutually exclusive splicing of mammalian α-tropomyosin and α-actinin.Citation17,Citation31 In this case, the intron (215 nt) is at first sight above the minimum size, but the exon 3 branch point is only 41 nt from the 5′ splice site of exon 2, and the intron is effectively too small. However, the 14–3-3ζ RNA hairpin is not conserved in Drosophila, Coleopteran Tribolium castaneum (red flour beetle), or the Hymenopteran Apis mellifera. Together, these data suggest that RNA-hairpin-mediated steric hindrance is an evolutionarily conserved mechanism in Lepidopteran species. Although intron size commonly interferes with splicing, this is the first case mediated by dsRNA.
Figure 2. Hairpins are evolutionarily conserved in lepidopteran species. Sequences aligned from the indicated lepidopteran species (for abbreviations used, see Supp Materials). Symbols used are the same as in . Identical nucleotides at each position are shown in different colors. RNA hairpins within IE1 and the intronic RNA pairings between the selector sequences and the docking site were predicted using the Mfold program.Citation25
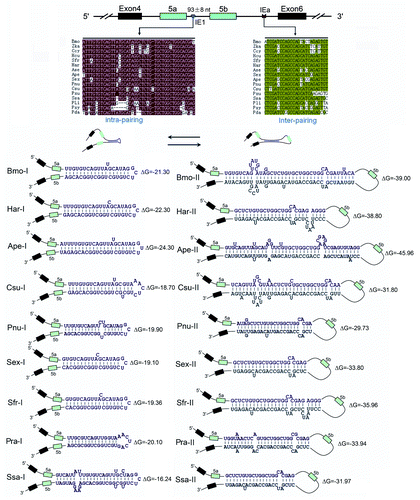
Regulation of mutually exclusive splicing by competitive RNA pairing between intra- and inter-introns
In an attempt to elucidate how the strength of intronic RNA pairing modulates the exon 5 selection, a series of mutations were generated to change RNA pairing competition between intra- and inter-introns to test how RNA pairings between intra- and inter-introns contributes to the selection of exon 5 variants (). Mutations that strengthened the RNA hairpin prominently increased the exon 5b frequency (). Analysis of the series of mutations indicated that the RNA pairing competition between intra- and inter-introns positively correlated with the exon 5a frequency ().
Figure 3. Regulation of mutually exclusive splicing by competitive RNA pairing between intra- and inter-introns. (A) Genomic organization of the 14–3-3ζ gene of silkworm. The symbols used are the same as in . (B) Schematic diagrams of mutant constructs for splicing. The predicted mutually exclusive RNA pairings are shown. Mutations introduced into dsRNA are indicated (M1-M6). Also shown are the predicted RNA pairings for wild-type RNA and a series of mutants (point mutations are shown in boldface). The arrow depicts the suppression of exon 5b. (C) Modulation of exon 5a selection by mutation analysis. (D) Correlation analysis of the RNA pairing competition between intra- and inter-introns using exon 5a selection.
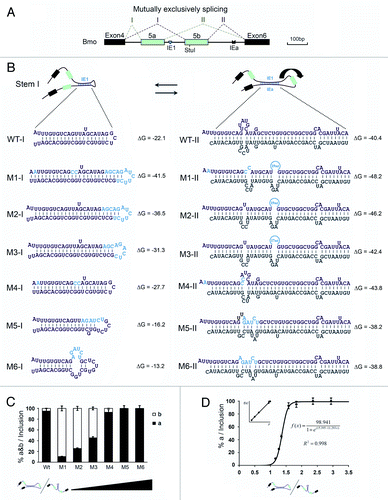
These results are largely consistent with the correlation of dynamic competition between intra- and inter-intronic RNA pairing and the ratio of exon 5a/5b in the Lepidopteran species. RT-PCR experiments revealed that the exon 5 cluster is subject to alternative splicing in a development- and tissue-specific manner. In contrast, exon 5a was generally selected at greater frequency (> 85%) in all tissues examined (Fig. S1). Combining the analyses of alternative splicing patterns with RNA secondary structure prediction from 15 Lepidopteran species indicated that the exon 5a frequency was relatively independent of the strength of intronic RNA pairing or inter-intronic RNA pairing. However, there was a relatively good correlation with competitiveness between intra- and inter-intronic RNA pairing (). This suggests that dynamic intronic RNA pairing could potentially regulate alternative splicing.
Figure 4. Correlation of the dynamic competition between intra- and inter-intronic RNA pairing with the ratio of exon 5a/5b. Correlation analysis of intra-intronic RNA pairing strength (A), inter-intronic RNA pairing strength (B), and their relative strength (C) with the ratio of exon 5a in Lepidopteran species.
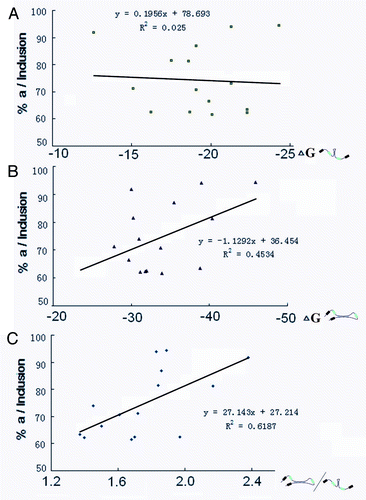
Approximation/activation for RNA pairing in silkworm was not as evident as in D. melanogaster
Theoretically, the combination of RNA-hairpin-mediated steric hindrance or the “looping-out” mechanism is sufficient to control mutually exclusive behaviors in silkworm. Indeed, mutual exclusivity in splicing is assured in the absence of inter-intronic RNA pairing by deleting the IE1 element. We were curious to know whether the approximation-activation mechanism also functions in Drosophila.Citation22 To address this, we created a series of constructs that mutated IE1 and/or IEa in combination with deleting the exon 5b sequence in silkworm (). These deletions were designed to mimic the approximation of sequences caused by the inter-intronic IE1-to-IEa pairing. RT-PCR analysis and sequencing revealed that exon 5a was almost exclusively included in all constructs even though these constructs lacked IE1-to-IEa pairing (), suggesting that that RNA pairing is not essential for exon 5a activation. However, this splicing pattern is indistinguishable from that observed for the deletion constructs in Drosophila.Citation22 This is due to the smaller size of the downstream intron in silkworm; approximation was not as evident as in D. melanogaster. In the latter case, the activation of the target exon was more sensitive to the distance to the approximated elements, while exon 5a could be efficiently activated even with ~200 bp of interval distance upstream the approximated elements in silkworm (). This implies that tighter and finer approximation/activation mechanism evolved in D. melanogaster 14–3-3ζ with three mutually exclusive exons.
Figure 5. Effects of mutations and deletions on exon 5a inclusion. (A) Overview of the minigene constructs used to test the importance of inter-intronic RNA pairing. The red arrow in IE or IEa denotes the disrupting mutation. IE1, IE1, and IEa elements are indicated as in . WT: wildtype. (B) Effects of exon 5 selection by the series of deletions and mutations. Exon 5a is exclusively included in all constructs even though these constructs lack IE1 and IEa.
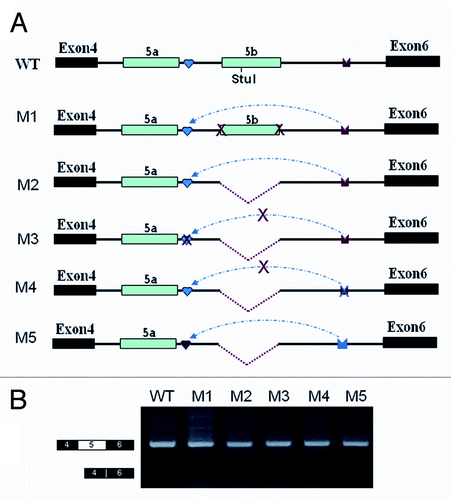
RNA structural architecture acts as a physical barrier to splicing
Our deletion analyses revealed that inter-intronic RNA pairing may not assist the direct activation of the upstream exon 5a. Thus, we hypothesized that if mutually exclusive splicing is mainly controlled by physical barriers, the context of exon 5a would have little effect on it. Indeed, the deletion of the middle part of exon 5a had little effect on the exon selection pattern (Fig. S1A, B). These results are supported by the splicing outcome in heterologous cells. A similar mutually exclusive splicing pattern was achieved when the silkworm 14–3-3ζ minigene was transfected into heterologous Drosophila S2 cells, and even HeLa cells (i.e., cells from a distantly related organism; Fig. S1C). Thus, splicing regulation appears to be highly conserved between mammals and insects. Overall, these observations indicate that exon 5 sequences have no remarkable effect on the splicing pattern, implying that RNA structure plays a key role in splicing regulation. These data suggest that RNA secondary structures largely act as physical barriers or frames for alternative splicing, whereas multiple linear cis-elements and several trans-acting factors fine-tune this process.
Discussion
Implications for splicing regulation
Traditional models of regulated alternative splicing involve other cis-regulatory elements (i.e., splicing enhancers and silencers), in addition to the canonical splice site signals. They function through binding of trans-acting factors, either enhancing or repressing the ability of the spliceosome to recognize particular splice sites.Citation2,Citation32,Citation33 Although single-stranded pre-mRNAs typically are depicted in a linear fashion, secondary structures are assumed to exist in a large fraction of protein-coding regions.Citation34 Indeed, some examples have indicated that RNA secondary structures are involved in splicing regulation.Citation7,Citation8,Citation19,Citation21,Citation35-Citation38 In yeast, new discoveries using high-throughput sequencing have lent strong support to the general hypothesis that the information encoded in large mRNAs is regulated and organized by RNA structure.Citation39 Therefore, RNA secondary structure strongly contributes to alternative splicing regulation in higher organisms.
Our results demonstrate that RNA structures not only present specific binding sites for trans-acting splicing factors, but also act as cis-acting physical frames or barriers. Moreover, intra-molecular RNA pairing competition can generate an alternative RNA structure of pre-mRNA, thus controlling alternative splicing. Recent studies have revealed that alternative RNA structure potentially affects alternative splicing.Citation36,Citation40 RNA secondary structures such as Dscam (38,016 isoforms) and Mhc (480 isoforms) play a determining role in complex alterative splicing regulation.Citation19,Citation20,Citation22,Citation41,Citation42 More generally, alterative splicing may be regulated by a combination of RNA structure with multiple linear cis-elements and several trans-acting factors. In this model, RNA structures act as a physical barrier or a backbone for the splicing machinery, whereas multiple linear cis-elements and several trans-acting factors provide fine-scale regulation. Moreover, it is worth noting how splicing can occur upon transcription when it is complicated by RNA secondary structures.Citation42
Evolution of splicing control mechanisms
The 14–3-3ζ gene underwent species-specific exon expansion during metazoan evolution. We identified three mutually exclusive exons in the 14–3-3ζ gene from the Drosophila simulans complex and A. mellifera, while only two copies exist in other insect species.Citation22 In addition, two duplicated exons were also discovered in 14–3-3ζ orthologs of Caenorhabditis elegans and closely related species (). However, phylogenetic analyses revealed that duplication events occurred independently in the insect and worm lineages, suggesting that these splice variants have intrinsic functional benefits. Interestingly, control mechanisms for alternative splicing diverged during subsequent evolution.
Figure 6. A refined model for regulating mutually exclusive splicing of silkworm 14–3-3ζ pre-mRNA. (A) Divergence of control mechanisms of mutually exclusive splicing. Exons that are duplicated in tandem are shown in colored boxes while constitutive exons (CE) flanking the duplicated exons are shown in black. Docking sites (marked by saddle shapes) are reverse-complementary to an upstream selector sequence (marked by hearts). The exon duplications (solid circles) produce the new exon copy. The introns are represented by lines, and not drawn to scale. “+” and “-” indicate the presence or absence, respectively, of underlying mechanisms for mutually exclusive splicing. “(RP+)” and “(RP-)” denote the RNA pairing-dependent and RNA pairing-independent mechanisms, respectively. “n.d” means “not determined.” (B) A refined model for controlling mutually exclusive splicing. The red and green circles represent the selector sequences and the docking site, respectively. The red diamond in the exon depicts the exonic enhancer. The arrow depicts the suppression of exon 5b. When the selector sequence (IE1) can interact with the docking site (IEa), the exon 5a variant is included in the 14–3-3ζ mRNA. Then inter-intronic RNA pairing excludes in-loop exon 5b via a looping-out mechanism. Alternatively, the RNA hairpin is assumed to form within the IE1 sequence. Thus, the resulting steric hindrance mechanism should dominantly control mutually exclusive splicing of these pre-mRNAs.
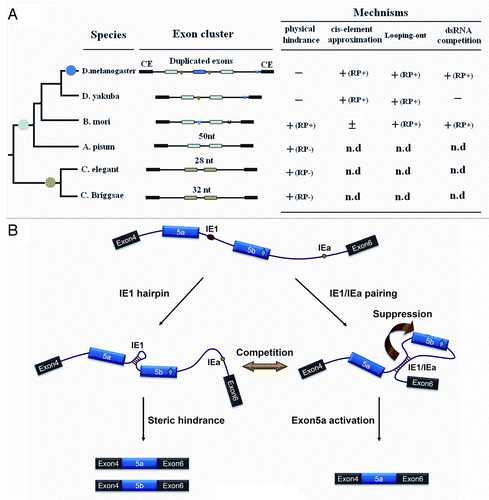
In D. melanogaster 14–3-3ζ, mutually exclusive splicing appears to be synergistically controlled by the superimposition of three combinatorial mechanisms, through activation of the proximal variable exon outside the loop by the approximation of cis elements, and by simultaneous repression of the exon within the loop, in combination with the physical competition of RNA pairing.Citation22 In D. yakuba 14–3-3ζ with two duplicated exons, mutually exclusive splicing was controlled via the former two mechanisms (data not shown). In silkworm, the RNA hairpin-mediated steric hindrance involves mutually exclusive splicing, in addition to the repression of the exon within the loop by inter-intronic RNA pairing (). Activation of the proximal variable exon outside the loop by the approximation of cis elements was not as evident as in Drosophila. Interestingly, the intron between exon 5a and exon 5b was only 50 nt in the 14–3-3ζ ortholog from another insect species, Acyrthosiphon pisum. This was below the intron threshold (around 60 nt) for splicing in Drosophila.Citation28 Similarly, the intron between exon 5a and exon 5b was only 28 nt and 32 nt in C. elegents and C. briggsae orthologs, respectively (). In these cases, steric hindrance should dominantly control mutually exclusive splicing of these pre-mRNAs.
Theoretically, the presence of either steric hindrance mechanism (in C. elegents) is sufficient to control mutually exclusive behavior. Why have some species evolved such a complex mechanism to control mutually exclusive behavior? For example, the process of Dscam and 14–3-3ζ variable exon choice in D. melanogaster relies on a combination of alternative RNA structure and repressors, which act in concert to activate a variable exon.Citation14,Citation22 First, the latter arrangement would be advantageous when considering the evolutionary expansion from the two-duplicate-exon cluster to a three-duplicate-exon or a multiple-exon cluster.Citation22 Some complex alternative splicing has been achieved by expansion of the exon cluster through non-allelic homologous recombination.Citation22,Citation43 Second, this combinatorial code of exon choice adds an additional layer to the higher-order regulation of splicing. In this manner, the number of protein regulators needed to govern the selection of a variable exon may be reduced. Moreover, this multi-layer control system may help increase the efficiency and flexibility of this splicing regulation.
Materials and Methods
Materials
Fruit flies (D. melanogaster, D. yakuba) and some lepidopteran species were obtained as previously reported.Citation22,Citation44 Pyrausta nubilalis were donated by the Sericulture Research Institute, Zhejiang Academy of Agricultural Sciences. Plutella xylostella and Pieris rapae were donated by the Institute of Insect Sciences, Zhejiang University. Additional lepidopteran species were collected from our campus. Total RNA was isolated using the RNeasy Mini Kit (Qiagen, Hilden, Germany). Genomic DNA was isolated using the Universal Genomic DNA Extraction Kit (TaKaRa, Dalian, China).
Cloning and sequencing of 14–3-3ζ orthologs
Whole genomic DNA was isolated using the QIAamp DNA kit (Qiagen). The same primers were utilized for members within lepidopteran species.Citation22 In most cases, degenerate primers were necessary to amplify products from difficult species. Amplification products were cloned into the pGEM-T Easy vector (Promega, Madison, WI) for sequencing.
Sequence alignments and RNA pairing predictions
Alignments of specific regions between species were performed using the ClustalW2 program (ebi.ac.uk/clustalw/index.html). The intronic RNA pairings were predicted using Mfold program.Citation25
Minigene constructions and mutagenesis
Plasmid DNA constructions used in this study were made by standard cloning methods as previously described.Citation22 Deletion and site mutagenesis was performed as described in the schematic diagrams of minigene constructs by PCR (, , ; Fig. S3). Silkworm mutant constructs were further cloned into pBluescript-SK+ under the Baculovirus IE1 promoter (a gift from C. Gong, Shuzhou University, China). Constructs are summarized in the text and figure legends. Primer sequences are listed in Table S1. More specific details can be obtained upon request from the authors.
To investigate whether the splicing pattern of these minigenes was dependent on the cell line used, we used different cell lines. The silkworm minigene was further cloned into the pMT/V5-HisA vector (Invitrogen, Carlsbad, CA) under control of the metallothionein promoter, and cloned into the pcDNA3.0 vector (Invitrogen), which has the CMV promoter, respectively. All constructs were confirmed by sequencing.
Transfection
Linearized DNA was transfected into silkworm cells (BmN) with Lipofectin (Invitrogen, Carlsbad, CA) according to the manufacturer’s protocol. The linearized recombinant pMT/V5-HisA DNA was transfected into Drosophila S2 cells, while linearized recombinant pcDNA3.0 DNAs was transfected into HeLa cells (gift from N. Zhou, Zhejiang University, China). Total RNAs were harvested 48 h after transfection using Trizol (Invitrogen, Carlsbad, CA). RT-PCR and exon-specific restriction enzyme analyses were performed to detect the alternative splicing status. Error bars were calculated from the average of three independent experiments.
Quantification of mRNA splice isoforms
We assayed the RNA splice isoform ratio using RT-PCR followed by exon-specific restriction digestion. Total RNA was isolated from different tissues and transfected cells. Total RNA was reverse transcribed using SuperScript III RTase (Invitrogen, Carlsbad, CA) with oligo(dT)15 or specific primers, and the resulting single-stranded cDNA product was treated with DNase I at 37°C for 30 min. Then the RT-PCR products were digested by exon-specific restriction enzymes, based on the minigene schematic diagrams (, , ; Fig. S3). Images were taken with a CCD camera, and the mRNA isoforms were quantified by comparing the integrated optical density of detected bands measured by the GIS 1D Gel Image System ver. 3.73 (Tanon, Shanghai, China). Data were expressed as the percentage of the mean and standard deviation from the average of three independent experiments.
Additional material
Download Zip (181.5 KB)Acknowledgments
This work was partly supported by research grants from the National Natural Science Foundation of China (31125011, 31071148), the Natural Science Foundation of Zhejiang Province (No. R3090177), and the Doctoral Foundation of Ministry of Education (20110101130012), and Fundamental Research Funds for the Central Universities.
Disclosure of Potential Conflicts of Interest
No potential conflicts of interest were disclosed.
Accession Numbers
The insect 14–3-3ξ gene sequences were deposited into GenBank with accession numbers HQ848651- HQ848651.
Note
Supplemental material can be found at: http://www.landesbioscience.com/journals/rnabiology/article/20205
References
- Wang Z, Burge CB. Splicing regulation: from a parts list of regulatory elements to an integrated splicing code. RNA 2008; 14:802 - 13; http://dx.doi.org/10.1261/rna.876308; PMID: 18369186
- Nilsen TW, Graveley BR. Expansion of the eukaryotic proteome by alternative splicing. Nature 2010; 463:457 - 63; http://dx.doi.org/10.1038/nature08909; PMID: 20110989
- Ozsolak F, Milos PM. RNA sequencing: advances, challenges and opportunities. Nat Rev Genet 2011; 12:87 - 98; http://dx.doi.org/10.1038/nrg2934; PMID: 21191423
- Pan Q, Shai O, Lee LJ, Frey BJ, Blencowe BJ. Deep surveying of alternative splicing complexity in the human transcriptome by high-throughput sequencing. Nat Genet 2008; 40:1413 - 5; http://dx.doi.org/10.1038/ng.259; PMID: 18978789
- Sultan M, Schulz MH, Richard H, Magen A, Klingenhoff A, Scherf M, et al. A global view of gene activity and alternative splicing by deep sequencing of the human transcriptome. Science 2008; 321:956 - 60; http://dx.doi.org/10.1126/science.1160342; PMID: 18599741
- Smith CW. Alternative splicing--when two’s a crowd. Cell 2005; 123:1 - 3; http://dx.doi.org/10.1016/j.cell.2005.09.010; PMID: 16213205
- Muh SJ, Hovhannisyan RH, Carstens RP. A Non-sequence-specific double-stranded RNA structural element regulates splicing of two mutually exclusive exons of fibroblast growth factor receptor 2 (FGFR2). J Biol Chem 2002; 277:50143 - 54; http://dx.doi.org/10.1074/jbc.M207409200; PMID: 12393912
- Baraniak AP, Lasda EL, Wagner EJ, Garcia-Blanco MA. A stem structure in fibroblast growth factor receptor 2 transcripts mediates cell-type-specific splicing by approximating intronic control elements. Mol Cell Biol 2003; 23:9327 - 37; http://dx.doi.org/10.1128/MCB.23.24.9327-9337.2003; PMID: 14645542
- Baraniak AP, Chen JR, Garcia-Blanco MA. Fox-2 mediates epithelial cell-specific fibroblast growth factor receptor 2 exon choice. Mol Cell Biol 2006; 26:1209 - 22; http://dx.doi.org/10.1128/MCB.26.4.1209-1222.2006; PMID: 16449636
- Gromak N, Rideau A, Southby J, Scadden AD, Gooding C, Hüttelmaier S, et al. The PTB interacting protein raver1 regulates alpha-tropomyosin alternative splicing. EMBO J 2003; 22:6356 - 64; http://dx.doi.org/10.1093/emboj/cdg609; PMID: 14633994
- Expert-Bezançon A, Sureau A, Durosay P, Salesse R, Groeneveld H, Lecaer JP, et al. hnRNP A1 and the SR proteins ASF/SF2 and SC35 have antagonistic functions in splicing of beta-tropomyosin exon 6B. J Biol Chem 2004; 279:38249 - 59; http://dx.doi.org/10.1074/jbc.M405377200; PMID: 15208309
- Lin JC, Tarn WY. Exon selection in alpha-tropomyosin mRNA is regulated by the antagonistic action of RBM4 and PTB. Mol Cell Biol 2005; 25:10111 - 21; http://dx.doi.org/10.1128/MCB.25.22.10111-10121.2005; PMID: 16260624
- Wagner EJ, Baraniak AP, Sessions OM, Mauger D, Moskowitz E, Garcia-Blanco MA. Characterization of the intronic splicing silencers flanking FGFR2 exon IIIb. J Biol Chem 2005; 280:14017 - 27; http://dx.doi.org/10.1074/jbc.M414492200; PMID: 15684416
- Olson S, Blanchette M, Park J, Savva Y, Yeo GW, Yeakley JM, et al. A regulator of Dscam mutually exclusive splicing fidelity. Nat Struct Mol Biol 2007; 14:1134 - 40; http://dx.doi.org/10.1038/nsmb1339; PMID: 21188797
- Ohno G, Hagiwara M, Kuroyanagi H. STAR family RNA-binding protein ASD-2 regulates developmental switching of mutually exclusive alternative splicing in vivo. Genes Dev 2008; 22:360 - 74; http://dx.doi.org/10.1101/gad.1620608; PMID: 18230701
- David CJ, Chen M, Assanah M, Canoll P, Manley JL. HnRNP proteins controlled by c-Myc deregulate pyruvate kinase mRNA splicing in cancer. Nature 2010; 463:364 - 8; http://dx.doi.org/10.1038/nature08697; PMID: 20010808
- Smith CW, Nadal-Ginard B. Mutually exclusive splicing of alpha-tropomyosin exons enforced by an unusual lariat branch point location: implications for constitutive splicing. Cell 1989; 56:749 - 58; http://dx.doi.org/10.1016/0092-8674(89)90678-8; PMID: 2924347
- Letunic I, Copley RR, Bork P. Common exon duplication in animals and its role in alternative splicing. Hum Mol Genet 2002; 11:1561 - 7; http://dx.doi.org/10.1093/hmg/11.13.1561; PMID: 12045209
- Graveley BR. Mutually exclusive splicing of the insect Dscam pre-mRNA directed by competing intronic RNA secondary structures. Cell 2005; 123:65 - 73; http://dx.doi.org/10.1016/j.cell.2005.07.028; PMID: 16213213
- Anastassiou D, Liu H, Varadan V. Variable window binding for mutually exclusive alternative splicing. Genome Biol 2006; 7:R2; http://dx.doi.org/10.1186/gb-2006-7-1-r2; PMID: 16507134
- May GE, Olson S, McManus CJ, Graveley BR. Competing RNA secondary structures are required for mutually exclusive splicing of the Dscam exon 6 cluster. RNA 2011; 17:222 - 9; http://dx.doi.org/10.1261/rna.2521311; PMID: 21159795
- Yang Y, Zhan L, Zhang W, Sun F, Wang W, Tian N, et al. RNA secondary structure in mutually exclusive splicing. Nat Struct Mol Biol 2011; 18:159 - 68; http://dx.doi.org/10.1038/nsmb.1959; PMID: 21217700
- Aitken A. 14-3-3 proteins on the MAP. Trends Biochem Sci 1995; 20:95 - 7; http://dx.doi.org/10.1016/S0968-0004(00)88971-9; PMID: 7709434
- Skoulakis EM, Davis RL. Olfactory learning deficits in mutants for leonardo, a Drosophila gene encoding a 14-3-3 protein. Neuron 1996; 17:931 - 44; http://dx.doi.org/10.1016/S0896-6273(00)80224-X; PMID: 8938125
- Kockel L, Vorbrüggen G, Jäckle H, Mlodzik M, Bohmann D. Requirement for Drosophila 14-3-3 zeta in Raf-dependent photoreceptor development. Genes Dev 1997; 11:1140 - 7; http://dx.doi.org/10.1101/gad.11.9.1140; PMID: 9159395
- Philip N, Acevedo SF, Skoulakis EM. Conditional rescue of olfactory learning and memory defects in mutants of the 14-3-3zeta gene leonardo. J Neurosci 2001; 21:8417 - 25; PMID: 11606630
- Ruskin B, Greene JM, Green MR. Cryptic branch point activation allows accurate in vitro splicing of human beta-globin intron mutants. Cell 1985; 41:833 - 44; http://dx.doi.org/10.1016/S0092-8674(85)80064-7; PMID: 3879973
- Kennedy CF, Berget SM. Pyrimidine tracts between the 5′ splice site and branch point facilitate splicing and recognition of a small Drosophila intron. Mol Cell Biol 1997; 17:2774 - 80; PMID: 9111348
- Zuker M. Mfold web server for nucleic acid folding and hybridization prediction. Nucleic Acids Res 2003; 31:3406 - 15; http://dx.doi.org/10.1093/nar/gkg595; PMID: 12824337
- Novikova O, Sliwińska E, Fet V, Settele J, Blinov A, Woyciechowski M. CR1 clade of non-LTR retrotransposons from Maculinea butterflies (Lepidoptera: Lycaenidae): evidence for recent horizontal transmission. BMC Evol Biol 2007; 7:93; http://dx.doi.org/10.1186/1471-2148-7-93; PMID: 17588269
- Southby J, Gooding C, Smith CW. Polypyrimidine tract binding protein functions as a repressor to regulate alternative splicing of alpha-actinin mutally exclusive exons. Mol Cell Biol 1999; 19:2699 - 711; PMID: 10082536
- Wang Z, Rolish ME, Yeo G, Tung V, Mawson M, Burge CB. Systematic identification and analysis of exonic splicing silencers. Cell 2004; 119:831 - 45; http://dx.doi.org/10.1016/j.cell.2004.11.010; PMID: 15607979
- Barash Y, Calarco JA, Gao W, Pan Q, Wang X, Shai O, et al. Deciphering the splicing code. Nature 2010; 465:53 - 9; http://dx.doi.org/10.1038/nature09000; PMID: 20445623
- Katz L, Burge CB. Widespread selection for local RNA secondary structure in coding regions of bacterial genes. Genome Res 2003; 13:2042 - 51; http://dx.doi.org/10.1101/gr.1257503; PMID: 12952875
- Howe KJ, Ares M Jr.. Intron self-complementarity enforces exon inclusion in a yeast pre-mRNA. Proc Natl Acad Sci U S A 1997; 94:12467 - 72; http://dx.doi.org/10.1073/pnas.94.23.12467; PMID: 9356473
- Miriami E, Margalit H, Sperling R. Conserved sequence elements associated with exon skipping. Nucleic Acids Res 2003; 31:1974 - 83; http://dx.doi.org/10.1093/nar/gkg279; PMID: 12655015
- Kreahling JM, Graveley BR. The iStem, a long-range RNA secondary structure element required for efficient exon inclusion in the Drosophila Dscam pre-mRNA. Mol Cell Biol 2005; 25:10251 - 60; http://dx.doi.org/10.1128/MCB.25.23.10251-10260.2005; PMID: 16287842
- Raker VA, Mironov AA, Gelfand MS, Pervouchine DD. Modulation of alternative splicing by long-range RNA structures in Drosophila. Nucleic Acids Res 2009; 37:4533 - 44; http://dx.doi.org/10.1093/nar/gkp407; PMID: 19465384
- Kertesz M, Wan Y, Mazor E, Rinn JL, Nutter RC, Chang HY, et al. Genome-wide measurement of RNA secondary structure in yeast. Nature 2010; 467:103 - 7; http://dx.doi.org/10.1038/nature09322; PMID: 20811459
- Glazov EA, Pheasant M, Nahkuri S, Mattick JS. Evidence for control of splicing by alternative RNA secondary structures in Dipteran homothorax pre-mRNA. RNA Biol 2006; 3:36 - 9; http://dx.doi.org/10.4161/rna.3.1.2719; PMID: 17114937
- McManus CJ, Graveley BR. RNA structure and the mechanisms of alternative splicing. Curr Opin Genet Dev 2011; 21:373 - 9; http://dx.doi.org/10.1016/j.gde.2011.04.001; PMID: 21530232
- Jin Y, Yang Y, Zhang P. New insights into RNA secondary structure in the alternative splicing of pre-mRNAs. RNA Biol 2011; 8:450 - 7; http://dx.doi.org/10.4161/rna.8.3.15388; PMID: 21558794
- Lee C, Kim N, Roy M, Graveley BR. Massive expansions of Dscam splicing diversity via staggered homologous recombination during arthropod evolution. RNA 2010; 16:91 - 105; http://dx.doi.org/10.1261/rna.1812710; PMID: 19934230
- Lv J, Yang Y, Yin H, Chu F, Wang H, Zhang W, et al. Molecular determinants and evolutionary dynamics of wobble splicing. Mol Biol Evol 2009; 26:1081 - 92; http://dx.doi.org/10.1093/molbev/msp023; PMID: 19221008