Abstract
Mechanisms by which eukaryotic internal ribosomal entry sites (IRESs) initiate translation have not been well described. Viral IRESs utilize a combination of secondary/tertiary structure concomitant with sequence specific elements to initiate translation. Eukaryotic IRESs are proposed to utilize the same components, although it appears that short sequence specific elements are more common. In this report we perform an extensive analysis of the IRES in the human tau mRNA. We demonstrate that the tau IRES exhibits characteristics similar to viral IRESs. It contains two main structural domains that exhibit secondary interactions, which are essential for internal initiation. Moreover, the tau IRES is extremely sensitive to small nucleotide substitutions. Our data also indicates that the 40S ribosome is recruited to the middle of the IRES, but whether it scans to the initiation codon in a linear fashion is questioned. Overall, these results identify structural and sequence elements critical for tau IRES activity and consequently, provide a novel target to regulate tau protein expression in disease states including Alzheimer disease and other tauopathies.
Introduction
The hyperphosphorylation of tau, a microtubule associated protein, results in its dissociation from microtubules, microtubule destabilization and accumulation of hyperphosphorylated tau in paired helical filaments or tangles.Citation1,Citation2 The formation of tangles intracellularly concomitant with insoluble Aβ peptide aggregates extracellularly are the key components contributing to neuronal death in Alzheimer disease (AD).Citation3-Citation5 However, decreased tau expression relieves symptoms associated with Alzheimer disease including cognitive decline and neuronal loss in mouse models.Citation6-Citation8 Consequently, targeting tau protein expression is a valid approach for the treatment of Alzheimer disease.
Our goal is to understand the post-transcriptional regulation of tau, primarily at the step of initiation, the major regulatory step in protein synthesis. The principal initiation mechanism involves recognition of the 7-methyl guanosine cap structure by the canonical initiation complex eIF4F, which in turn recruits the 40S ribosome. The ribosome then purportedly migrates along the 5′ leader until it reaches the initiation codon situated in proper context and begins translation.Citation9,Citation10
In a subset of viruses including members of the Picornaviridae and Flaviviridae families, an alternative initiation mechanism dispenses with the cap structure and recruits the ribosome directly to sites in the mRNA (generally the 5′ leader) called internal ribosomal entry sites (IRES). Viral IRESs are denoted by being long structured 5′ leaders that often contain multiple upstream open reading frames (uORFs).Citation11,Citation12 The structural component of viral IRESs is underscored by the observation that nucleotide sequences encompassing the IRES are not identical, yet phylogenetic preservation of the structure exists.Citation12-Citation14 The structural conservation of particular Hepatitis C Virus (HCV) IRES domains within HCV-like IRESs as well as other flaviviruses allow for similar function enabling the recruitment of the ribosome with a minimum number of initiation factors.Citation13,Citation15-Citation17 The structural complexity of another viral IRES family the Discistroviridae IGR IRESs permit the direct recruitment of the ribosome without any initiation factors.Citation18
In addition to structural conservation, specific sequence elements are often important and in some cases essential to initiating translation of viral IRESs. These small sequences present in apical or internal loops serve as binding sites for canonical initiation factors, IRES trans-acting factors termed ITAFs or they facilitate RNA-RNA interactions allowing for proper secondary and tertiary structure formation.Citation19-Citation21 For example, the conserved GNRA tetraloop of picornaviral IRESs facilitates tertiary interactions in the FMDV IRES that are essential for structural formation and IRES function.Citation21,Citation22 Additionally, the association of the ITAF Polypyrimidine Tract-Binding protein (PTB) with pyrimidine tracts presented in stem-loops of the encephalomyocarditis virus (EMCV) IRES stabilizes the secondary and tertiary structure allowing for 40S ribosomal recruitment.Citation20,Citation23
Eukaryotic IRESs are present in a subset of mRNAs, however the mechanisms by which these IRESs recruit the ribosome are less well known.Citation24,Citation25 Indeed, there are few examples that illustrate the role of both RNA secondary structure and RNA sequence specificity in mediating IRES activity. The IRES located in the 5′ leader of the mouse potassium channel mKv1.4 is one example that exhibits a predicted pseudoknot interaction, stem loop formations, as well as a polypyrimidine motif that is necessary for IRES activity.Citation26 Interestingly, the polypyrimidine tract, often in a hairpin, appears to be one motif that is common in many eukaryotic IRESs and has been suggested to be sufficient to recruit the ribosome internally.Citation27
In contrast to the larger structure based IRESs found in most viral and some eukaryotic IRESs, there are a subset of eukaryotic IRESs that rely on multiple shorter cis-elements within the RNA.Citation28-Citation32 Cis-elements of 7–50 nucleotides that mediate IRES-dependent translation are situated in several eukaryotic IRESs including TrkB, OCT4 and APP.Citation33-Citation35 In contrast to viral IRESs that are dependent on the proper folding of secondary structure and tertiary interactions, these cis-elements likely recruit the ribosome via a specified sequence, possibly through the initial recruitment of non-canonical factors.Citation36,Citation37
In the present report we sought to characterize the cis-elements required for internal initiation in the IRES located in the 5′ leader of the human tau mRNA. We show that the smallest and most prominent transcribed leader of 240 nucleotides is required for tau IRES activity. It generates an extensive secondary structure exhibiting interactions critical for IRES activity. In addition, multiple small nucleotide substitutions throughout the IRES dramatically decrease activity by affecting secondary structure or sequence specific binding. The requisite for specific cis-elements and RNA structure suggest the tau IRES represents another class of eukaryotic IRES, which is more viral like in its characteristics. Furthermore, this analysis also generates novel targets for regulating tau expression in tauopathies including Alzheimer disease.
Results
The goal of this study was to identify cis-elements required for internal initiation of the tau mRNA. The primary tau transcript contains a 240 nucleotide 5′ leader,Citation38-Citation40 which we discovered contains an IRES.Citation41 There is evidence that a minor transcriptional start site produces a 320 nucleotide leader (an 80 nucleotide extension at the 5′ end of the leader).Citation42 To determine if IRES activity was affected by the 80 nucleotide extension, the larger leader was inserted into the intercistronic region of a dicistronic construct.Citation43 The dicistronic construct contains a Renilla luciferase open reading frame (ORF) and a Photinus luciferase ORF as the first and second cistrons, respectively. Translation of the Renilla luciferase cistron occurs by a cap-dependent mechanism and is used to normalize for transfection efficiency. Translation of the second cistron (Photinus luciferase) essentially will not occur unless there is an IRES present to initiate translation. Dicistronic luciferase RNA transcripts were prepared that contained the tau 320 leader and the 240 tau 5′ leader as well as the BACE 1Citation44,Citation45 and β-globin 5′ leaders,Citation46 which were utilized as negative controls (). As a positive control the IRES from the encephalomyocarditis virus (EMCV) was employed.Citation47 Transcripts were transfected into the SK-N-SH neuroblastoma cell line and harvested for luciferase assay 4 h later. The Photinus to Renilla (P/R) luciferase ratio attained from the mRNAs containing the two tau 5′ leaders were similar at approximately 7 fold greater than either negative control (). The transfected RNA was isolated and semi-quantitative PCR was performed with Photinus luciferase ORF and GAPDH ORF specific primers (see Methods) in order to access the integrity of the RNA. Similar amounts of RNA were observed for each transfection (). This result suggests that the IRES is contained within the 240 nucleotide region of the tau 5′ leader.
Figure 1. The tau IRES is restricted to 240 nucleotides and contributes to total translation levels. (A) A dicistronic RNA transfection was done in SK-N-SH neuroblastoma cells for 4 hrs. The BACE 1 5′ leader, β-globin 5′ leader, 240 nucleotide tau, 320 nucleotide tau and EMCV 5′ leaders were inserted upstream of the Photinus luciferase gene and tested for IRES activity. IRES activity is reported as a ratio of Photinus luciferase/Renilla luciferase. The P/R ratio for the negative control BACE 1 was normalized to 1 (n=5). (B) An A-capped monocistronic RNA containing the β-globin 5′ leader, 240 nucleotide tau, or 320 nucleotide tau was transfected along with an m7G-capped Renilla luciferase gene to control for transfection efficiency. The P/R ratio for β-globin was normalized to 1 and the P/R ratios for tau were normalized accordingly (n=4). (C,D,F) Semi-quantitative PCR of transfected RNAs amplified with gene specific primers to Photinus luciferase or GAPDH. (E) A-capped and m7G capped monocistronic RNAs were transfected separately into SK-N-SH cells along with a co-transfected m7G capped Renilla luciferase. The P/R ratios for the m7G capped mRNAs were normalized to 1. The P/R ratios of the A-capped messages were then normalized to the m7G counterpart to obtain a relative amount of IRES activity (n=4). All transfections were performed in triplicate and the average ± SEM is plotted in all cases.
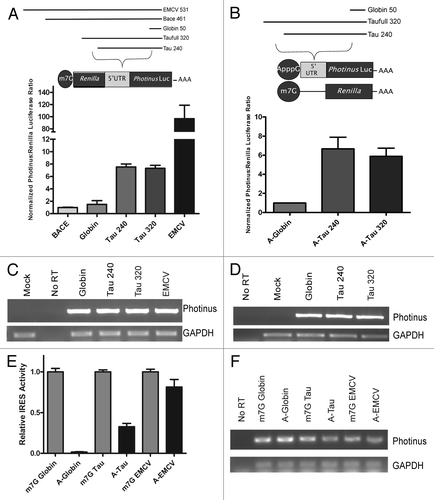
To further compare the IRES activity between the two tau 5′ leaders, they were examined in a monocistronic RNA containing an ApppG (A) cap. The A-cap analog provides stability to the RNA by preventing degradation due to exonuclease activity. It is also not recognized by the cap-binding protein eIF-4ECitation48,Citation49 thus translation is initiated in a cap-independent manner. Accordingly, A-capped mRNAs containing either of the two tau 5′ leaders or the β-globin 5′ leader, as a negative control, were transfected into the SK-N-SH neural cell line. The A-capped RNAs were co-transfected with an m7GpppG (m7G) capped mRNA coding for humanized Renilla luciferase, phRL, to control for transfection efficiency. The P/R ratio derived from the translation of both tau 5′ leaders was similar at 6-fold higher than the negative control β-globin (). As before, the RNA was isolated after the transfection to examine if the quality of the RNA was similar. The semi-quantitative analysis indicates a comparable amount of RNA was transfected (). Taken together, the discistronic and monocistronic assay further confirm that the tau 5′ leader contains an IRES and when present, the upstream 80 nucleotides of the larger 5′ leader does not affect IRES activity.
Identifying the contribution of the tau IRES to the translation of tau mRNA
The endogenous mRNA contains an m7G cap, and thus can be translated in a cap-dependent manner. However, in mRNAs that contain an IRES in the 5′ leader, translation can occur potentially by either a cap-dependent and/or cap-independent mechanism. Therefore to estimate the potential contribution of the tau IRES to the total level of translation, we compared the translation of monocistronic mRNAs with a m7G-cap or A-cap, containing the β-globin, EMCV, or tau 5′ leader in SK-N-SH cells. Transfections of the Photinus luciferase RNA were co-transfected with m7G capped Renilla luciferase mRNA to serve as a transfection control.
The A-cap/m7G cap ratio for each 5′ leader could be interpreted as the potential amount of cap-independent translation that contributes to the total (cap + IRES) translation of an m7G capped mRNA. The A-cap/m7G-cap ratio for β-globin was < 1% indicating that there is minimal cap-independent translation initiation occurring (). On the other hand, there were similar levels of translation between the m7G and A-cap Photinus luciferase mRNA containing the EMCV IRES. This result indicates that the presence of the m7G cap does not appear to have a significant role in the translation of an mRNA containing the EMCV IRES. Inclusion of the tau 5′ leader resulted in the A-cap/m7G cap ratio of ~30% of the amount of translation attained from the m7G capped counterpart (). Further isolation of the transfected RNA and semi-quantitative PCR was done to ensure that differential effects on RNA stability from the m7G or A-cap did not skew the interpretation of the data (). These results suggest that under basal conditions the tau IRES potentially contributes about one third to the total amount of translation. This value is comparable to what is observed for other eukaryotic IRESs.Citation50 It is likely that under different cellular conditions such as hypoxia or UV irradiation in which cap-dependent translation is downregulated, IRES-dependent translation may play a more significant role as has been reported for other cellular IRESs.Citation51,Citation52
The tau IRES requires the majority of the 5′ leader to facilitate internal initiation
To determine the region within the 240 nucleotides upstream of the initiation codon that contribute to IRES activity, a series of 5′ and 3′ truncations were created. The resulting truncations ranged from 60 to 200 nucleotides and were individually cloned into a monocistronic vector upstream of a Photinus luciferase ORF (). A-capped mRNA containing the β-globin, BACE 1, EMCV, tau 5′ leader and all truncations were co-transfected with an m7G capped Renilla luciferase RNA to normalize for transfection efficiency. The level of IRES activity from the wild type tau 5′ leader was ~7 fold over that obtained from the mRNA containing the β-globin 5′ leader. This result was similar to what had been observed previously in . In contrast, each truncation dramatically reduced or completely abolished IRES activity ().
Figure 2. The majority of the tau 5′ leader is required for IRES activity. (A) Schematic representation of deletions made from the 5′ or 3′ ends of the tau 5′ leader. Each deletion was inserted upstream of the Photinus luciferase gene. All RNAs were co-transfected with an m7G capped Renilla luciferase mRNA as a transfection control. (B) Transfections were done in SK-N-SH cells for 4 hrs. Photinus to Renilla luciferase ratios are normalized to the negative control, β-globin, which is given a value of 1. BACE 1 was also used as a negative control and EMCV IRES serves as the positive control for IRES activity. All transfections were performed in triplicate. Normalized P/R ratios are reported, (n = 6 ± S.E.M.). (C) Semi quantitative PCR of RNA isolated from the transfected cells was amplified with gene specific primers to either the Photinus luciferase or GAPDH ORF.
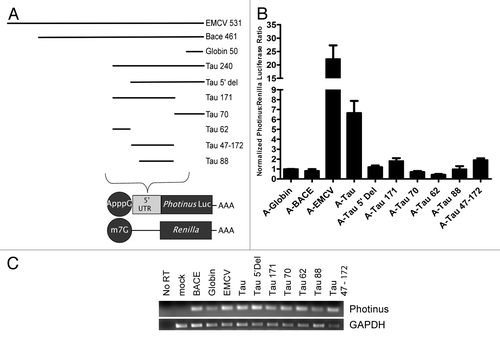
To determine if differential RNA stability contributed to the transfection results, the RNA from each transfection was re-isolated and semi-quantitative PCR was performed. The results show that RNA was present in the cell after each transfection in roughly comparable amounts suggesting that degradation of the RNA was an unlikely explanation for the dramatic decrease in IRES activity (). Taken together, these results indicate that the entire 240 nucleotide sequence is required for essentially any amount of IRES activity.
Long-range RNA interactions participate in tau IRES function
The observation that IRES activity required the entire 240 nucleotide tau 5′ leader for essentially any IRES activity suggested that either the large deleted segments were a sequence specific requirement for IRES activity and/or the deleted regions contributed to a global secondary structure. In order to determine the significance of any structural component of the tau IRES, SHAPE (Selective 2’ Hydroxyl Acylation analyzed by Primer Extension) analysis was performed. The SHAPE reaction provides a non-discriminatory look at nucleotides that are in an open conformation suggesting that they are single stranded vs. a more closed or double stranded conformation.Citation53,Citation54 Although SHAPE analysis primarily examines secondary structure, folding tertiary structure information can also be obtained. Since SHAPE chemistry is not limited by solvent accessibility, unconstrained nucleotides that are solvent inaccessible may still be modified by NMIA.Citation55 Conversely nucleotides constrained by base pair interaction (either secondary or tertiary) are unreactive thus allowing for a global interpretation of the RNA structure.Citation53,Citation55 In vitro transcribed RNA was subjected to the chemical reaction with 65mM or 30mM N-methylisatoic anhydride (NMIA) or DMSO for a total of 1hr. Cellular ion conditions and temperature may alter nucleotide binding specificity not observed in vitro. In order to address this issue the in vitro transcribed RNA was folded at near physiological concentrations of MgCl2 and at 37°C.Citation56 The resulting modifications were visualized on a sequencing gel (). Modified regions were identified by subtracting the negative control lane from either modified lane and plotted as a percentage of the highest modified residue (). Analyzing multiple sequencing gels produced a set of constraints that were used in a structural folding algorithim, mFold.Citation57 The RNA secondary structure that most accurately fit the data obtained by SHAPE is shown in .
Figure 3. Structural analysis of the tau IRES. (A) Reverse transcription of SHAPE analysis conducted on the tau 5′ leader. 65 mM NMIA or DMSO as control was used to modifiy the RNA. (B) Constraints identified from the SHAPE analysis were used in the mFold algorithim to produce the secondary structure of the tau IRES. The color coordination corresponds to the SHAPE reactivity of the nucleotides once the background (DMSO lane) is subtracted out. Red, highly modified; orange, moderate modification; yellow, elevated level of modification; green, low modification; black, no modification. (C) Normalized SHAPE reactivity with corresponding color coordination plotted against nucleotide position.
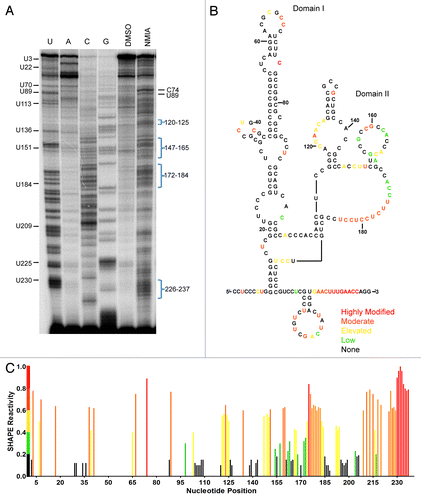
The predicted secondary structure of the tau IRES reveals two domains (). Domain I (C1-C101) is a large hairpin consisting of several internal bulges and a protruding stem loop. Moderate to low level NMIA modifications within domain I were mapped to residues in loops at C37-G40/A62-C69 and bulges at C74, C86-C90 and C-96-C98 ( and ). NMIA modifications were at or below background for the majority of domain I suggesting the nucleotides were constrained through base-pair interaction or by structural conformation. Domain II (C109-G240) is a more open structure as evidenced by the longer looped out single stranded region and the increased number of NMIA modifications (). Strong NMIA modifications were detected on the internal bulge at A119-A125, the polypyrimidine stretch at U175-U184, the stem loop at C207-G224 and the 3′end of the 5′ leader G226-C237. Less pronounced modifications were mapped to a stem loop at G154-C167 and adjacent to the polypyrimidine stretch at A172-C174 in domain II.
There are two regions of complementary sequence located at nucleotides 18–25 and 59–65 in domain I. Although the projected structure maps the complementary sequences to an internal bulge and apical loop, the regions were unmodified by NMIA suggesting the nucleotides are constrained possibly by base-pair interaction (). To examine whether it was probable that the apical loop at 59–65 interacts with the bulge at 18–25 creating a kissing hairpin, both regions were mutated by substituting adenosine with cytosine and guanosine with uridine and vice versa. These substitutions are used for all further mutations made in this study. Initially each site was disrupted individually and then both mutations were created as compensatory mutations. A-capped transcripts were prepared and transfected into the SK-N-SH cell line. In comparison to the tau wild type IRES, the mutations either resulted in greatly diminished IRES activity (mut 18–25, ) or abolished IRES activity (mut 59–65, ). The compensatory mutation (i.e., both mutations in the same construct) restored activity to a similar level as wild type. The restoration of IRES activity strongly suggested that these two regions interact. Semi-quantitative PCR demonstrated that the integrity of the RNA was not differentially affected ().
Figure 4. Long range interaction in domain I. (A) Schematic representation of mutated residues within the structure. Mutant 18–25 was mutated to 5′-TATCCAA. Mutant 59–65 was mutated to 5′-TTGGATA. Mutations were inserted upstream of the Photinus luciferase gene to test for IRES activity (B) P/R ratios are normalized to the negative control β-globin. A-capped transfections were done in SK-N-SH cells for 4 h and performed in triplicate, (n = 5 ± S.E.M.) (C) Semi quantitative PCR of isolated RNAs from transfected cells. Amplification was done with Photinus luciferase and GAPDH specific primers. (D) Native gel of the mutant RNAs. 1 µg of RNA was run on a 10% native gel for 8 h and stained with EtBr. Different migration rates correspond to the overall change in structure.
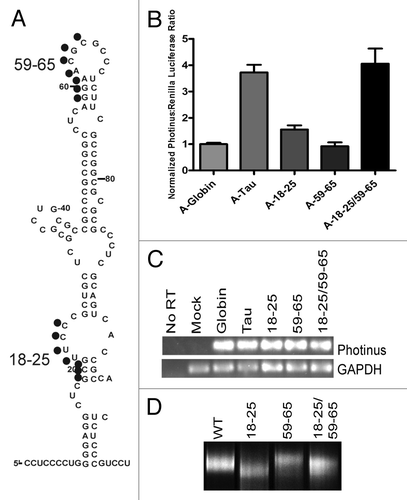
To further confirm the presence of a kissing hairpin interaction in domain I, native gel analysis was performed. The structural alterations due to mutations that were made are observed by running the folded RNA on a non-denaturing gel. Native gel analysis of the wild type and mutant tau 5′ leaders show subtle shifts in the migration rate of the 18–25 and 59–65 mut, inferring a change in their structural conformation (). Mut 18–25 ran at a faster migration rate while mut 59–65 migrated slower than wild type. The compensatory mutation has a migration rate that is very similar to that of wild type further suggesting that the RNA-RNA interaction has been restored (). Taken together, these results indicate that nucleotides 18–25 and 59–65 potentially associate forming a tertiary interaction by folding upon itself.
SHAPE analysis of the mutant tau 5′ leaders and the compensatory mutation were done in order to determine the effect of the nucleotide substitutions on secondary structure formation. Modified regions were determined as previously described and used to identify a set of constraints for secondary structure prediction. The mut 18–25 exhibited an overall decrease in the highest modified residues compared with wild type as well as a decrease in clusters of nucleotides that were modified (). Strong modifications were detected at U113 and U151 corresponding to two apical loops and moderate to low level modifications were detected in the substituted regions at G14-C23 and at A61, which is a distinction from wild type where only residue C65 is modified in the substituted region ( and ). Additional nucleotides predicted to form domain I were modified suggesting an increase in accessibility. The mut 18–25 predicted secondary structure suggests a more compacted domain II (C109-G240) correlating with the faster migration rate observed on the native gel analysis (). The majority of nucleotides were unmodified by NMIA on the 59–65 mutant leader (). Lower level modifications were detected at G19 and G78, and residues U60-G62 were now modified suggesting a shift in the accessibility of nucleotides previously constrained in the wild type structure (). Other distinguishing features of the mut 59–65 included the presence of several open loops in addition to a longer unstructured 3′ end of domain II (). These structural characteristics could impede migration of the RNA on a native gel supporting the observation that the mut 59–65 RNA ran slower than the wt leader.
Figure 5. Structure Analysis of Mutant Tau 5′ Leaders 18–25 and 59–65. (A) Secondary structure model of mut 18–25. The color coordination corresponds to the SHAPE reactivity of the nucleotide once the background (DMSO lane) is subtracted out. Red, highly modified; orange, moderate modification; yellow, elevated level of modification; green, low modification; black, no modification. (B) Reverse transcription analysis of the modified RNA in the presence of 65mM NMIA or DMSO. (C) Normalized SHAPE reactivity plotted vs. nucleotide position. (D) Secondary structure model of 59–65. The color coordination corresponds to the SHAPE reactivity of the nucleotide once the background (DMSO lane) is subtracted out. Red, highly modified; orange, moderate modification; yellow, elevated level of modification; green, low modification; black, no modification. (E) Reverse transcription analysis of the modified RNA in the presence of 65 mM NMIA or DMSO. (F) Normalized SHAPE reactivity plotted vs. nucleotide position.
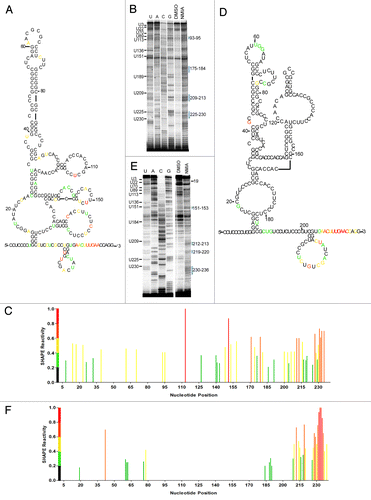
A comparison of the compensatory mutant (18–25/59–65) and wild type shows low level modifications at A58, U64-A65 and C67 suggesting the nucleotides 59–63 and 18–25 are constrained through base pair interaction (). Additionally, low level to strong modification of nucleotides at G94, A110-U113, U194, U212-C221and U225-G240 correspond to similar locations in domain II of the wild type structure (). The majority of the compensatory mutant was minimally modified suggesting that a restoration of the wild type IRES structure was not fully obtained. However, the predicted secondary structure shows similar characteristics to the wild type structure including the major hairpin of domain I (). The structural prediction of domain II also has similarities with wild type including an open polypyrimidine tract (C173-U184) and nucleotides A119-A125 are predicted to be in an open conformation (). In addition, the 3′ end of the IRES (G200-G240) is completely reiterated. The overall structural effect of the mutant tau 5′ leaders indicates that substitutions made within domain I alter the folding and accessibility of domain II. Furthermore, the two complementary regions 18–25 and 59–65 when mutated become more accessible while in the compensatory mutation nucleotides 59–63 and 18–25 are not modified. The results indicate that these regions are likely constrained by base pair interaction in the wild type structure, which could occur through a kissing hairpin interaction.
Figure 6. Structural Probing of the Compensatory Mutant 18–25/59–65. (A) Structural model of the compensatory mutant 18–25/59–65 with corresponding nucleotide reactivity. Red, highly modified; orange, moderate modification; yellow, elevated level of modification; green, low modification; black, no modification. (B) Reverse transcription of the modified RNA in the presence of 65 mM NMIA or DMSO as control. (C) Normalized SHAPE reactivity plotted against nucleotide position.
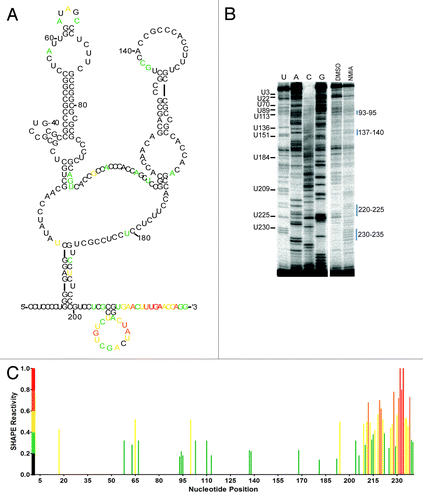
Analysis of predicted helix formation with additional domain I mutations
The proposed structural model of the tau IRES was further tested by creating additional mutations within domain I. The first set of mutations was made at the base of the hairpin (10–14 and 195–199) (). A-capped monocistronic RNA transfections were performed as described previously. The mut 10–14 did not exhibit any IRES activity, whereas the compensatory mutation (mut 10–14/195–199) led to IRES activity that was approximately 50% of wild type, () reinforcing the interpretation that these regions interact forming a major stem.
Figure 7. Helical mutations support structure formation. (A) Schematic representation of mutations made within the hairpin of domain I. Mutations were made in the following manner: A/C and G/T. Mutations were in vitro transcribed, capped with an A-cap and tailed. (B) P/R ratios are reported normalized to β-globin, which has been given the value of 1. A-capped RNAs were transfected in SK-N-SH cells for 4 hrs and performed in triplicate, (n = 4 ± S.E.M.) (C) Semi quantitative PCR of isolated transfected RNAs. PCR amplification was conducted with Photinus luciferase and GAPDH specific primers.
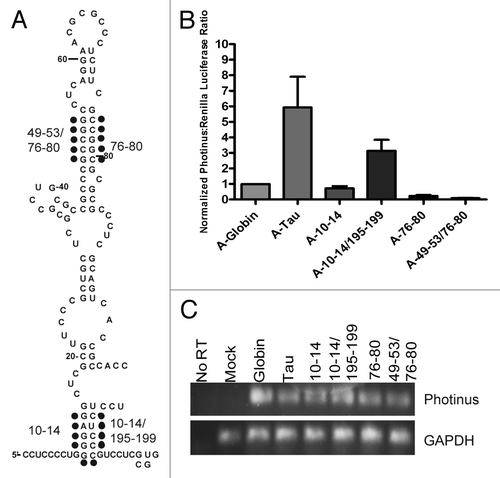
The second region to be manipulated was within the stem portion of the hairpin (G44-C53/G75-C84) (). Interestingly, both the initial set of nucleotide substitutions (mut 76–80) as well as combining it with the compensatory mutation (mut 49–53/76–80) resulted in the loss of all IRES activity (). The mutation within the stem portion of the hairpin suggests that this specific sequence is required for IRES activity. It is probable that the formation of this stem is dependent on the increased stability provided by seven contiguous G-C base pairs. The substitution with 4 A-U base pairs may not provide the bonding strength to accomplish stem formation. Alternatively, the sequence projected to form this stem may base pair with another region of the IRES not modified by NMIA.
Mutational analysis of domain II reveals sequences important for IRES activity
In domain II, two additional regions of complementary sequence were identified between nucleotides 109–118 and 130–137, although the predicted structure indicated these regions may interact with other parts of domain II (). Accordingly, each region was mutated as previously described and the compensatory mutation was also created. Mutation 109–118 led to a 50% decrease and mutation 130–137 led to a 75% decrease in tau IRES activity. The compensatory mutation resulted in a complete loss of IRES activity (). This data suggested that these regions likely do not interact. Since large nucleotide substitutions were created initially (~7–9 nucleotides), smaller substitutions were made within each region to identify key nucleotides that alter IRES activity. Within nucleotides 130–37 a triple substitution between nucleotides 135–137 and single nucleotide mutation U136G were made as well as a four nucleotide substitution of the apical loop region between nucleotides 130–134 (). Substitutions in the stem had no effect on IRES activity indicating that not all nucleotide substitutions in the tau IRES are deleterious. In contrast, the mutated apical loop (130–134) exhibited no IRES activity demonstrating that the nucleotide sequence is critical for maintaining IRES activity ().
Figure 8. Domain II mutations decrease tau IRES activity. (A) Schematic representation of mutations made within domain II. Mutations followed the A/C and G/T pattern. The blue dots refer to mutation 109–118 and purple dots indicate mutation 130–137. (D) Mutations made within mutant 130–137. Yellow stars indicate mutant 135–137, the arrow points to the single nucleotide mutation U136G and red boxes are mutant 130–134. (G) Mutations made within mutation 109–118. Black stars indicate mutation 109–111, arrow is the single nucleotide mutation A110C and orange squares are mutation 112–115. Mutations were in vitro transcribed, capped with an A-cap and tailed. (B, E and H) P/R ratios are reported normalized to β-globin, which was given a value of 1. A-capped transfections were done in the SK-N-SH cell line for 4 hrs and performed in triplicate, (n = 5 ± S.E.M.) (C, F and I) Semi quantitative PCR of isolated RNAs from each transfected well. Photinus luciferase and GAPDH specific primers were used to amplify products.
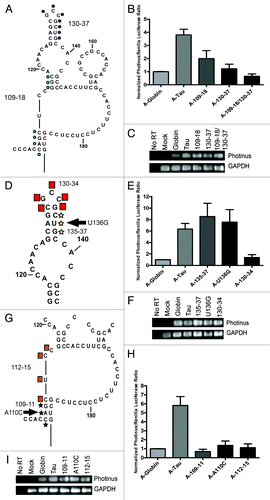
The seven contiguous nucleotides within 109–115 were also subdivided. The triple mutation (109–111) and single nucleotide mutation (A110C) resulted in a total loss of IRES activity (). An additional mutation (112–115) which internally bridges domain II also abolished IRES activity (). Taken together, mutations within domain II suggest that these regions of the tau IRES are dependent on a specific sequence and may also indicate a structural requirement in order to maintain a functional IRES.
Parkinson disease SNPs alter tau IRES activity
In our characterization of the tau IRES we queried if the tau 5′ leader had accumulated mutations that were relevant to neurodegenerative disease. The relationship of tau expression and formation of neurofibrillary tangles is an important component to the progression of Alzheimer disease. The formation of these tangles has also been documented in Parkinson disease patients.Citation58,Citation59 Analyses of the tau gene revealed a series of SNPs (single nucleotide polymorphisms) that are linked to the development of Parkinson disease.Citation60,Citation61 Two SNPs were present within the tau 5′ leader.Citation61,Citation62 The first SNP located at nucleotide 108 is a C to T transition and the second SNP is a deletion of a cytosine at position 199. The two SNPs were tested individually in an A-capped monocistronic RNA to determine if there was an effect on IRES activity (). Interestingly, both the C108T substitution as well as the 199C deletion resulted in a loss of IRES activity (). This result emphasizes the sensitivity of a single nucleotide mutation or deletion on the function of the tau IRES.
Figure 9. Parkinson's Disease related SNPs eliminate tau IRES activity. (A) Schematic representation of single nucleotide polymorphisms that were made in the tau 5′ leader to ascertain their effect on IRES activity. The first mutation is a C/T transition at nucleotide 108 and the second is a deletion of C199. Both mutations were in vitro transcribed, capped with an A-cap and tailed to test for IRES activity. (B) P/R ratios are reported normalized to β-globin, which was given a value of 1. A-capped transfections were done in the SK-N-SH cell line and performed in triplicate for 4 hrs, (n = 7 ± S.E.M.). (C) Semi quantitative PCR of isolated RNAs from each transfected well. Photinus luciferase and GAPDH specific primers were used to amplify products.
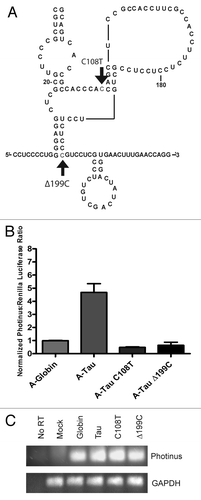
Introduction of upstream ORFs has differential effects on tau IRES activity
Our characterization of the tau IRES indicates there is a structured hairpin at the 5′ end of the IRES followed by a less structured region of the IRES that is more sequence dependent. To resolve where the ribosome is recruited to the IRES, out-of-frame upstream AUGs in a good Kozak sequence were inserted into the leader at locations 50, 140, 170, 200, 220 and 230 nucleotides from the 5′ end (). Translation of the uORFs would result in a corresponding decrease in Photinus luciferase activity as the uORFS are out of frame with the native AUG and the stop codon for the uORFs is located in the Photinus luciferase reading frame. Alternatively, Photinus luciferase expression will be uninhibited if the upstream AUGs are not recognized. AUA substitutions were also created as a negative control to ensure that the substitutions were not simply disrupting the structure and/or sequence of the IRES and therefore preventing IRES activity.
Figure 10. Upstream AUGs suggest a ribosome entry site. (A) Upstream AUG/AUAs were substituted out of frame with the native initiation codon, but in a Kozak sequence. Each mutated nucleotide is circled, in some cases surrounding nucleotides were mutated to create an optimal Kozak sequence. (B) The AUG/AUA substitutions were in vitro transcribed, capped with an A-cap and tailed. The A-capped RNAs were transfected into SK-N-SH cells to analyze for IRES activity. The P/R ratios were normalized to the wild type tau levels for comparison. Wild type tau was designated 1. (n = 3 ± S.E.M.) (C) Semi quantitative PCR was done on isolated RNA from each transfected well. Gene specific primers were used to amplify Photinus luciferase and GAPDH.
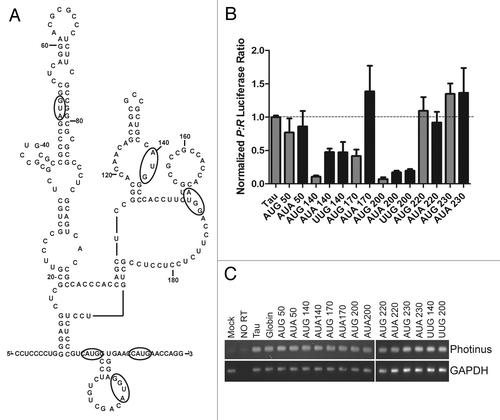
A-capped mRNAs containing the AUG/AUA insertions were created and transfected as previously described. The initial uORF (AUG) and control AUA at 50nt from the 5′ end of the leader did not affect Photinus luciferase expression (). These results indicate that the ribosome was not scanning from the 5′ end of the A-capped RNA and that the control AUA also did not affect translation by altering the secondary structure/sequence. In contrast, a dramatic decrease in IRES activity was observed for insertions of upstream ORFs at 140, 170 and 200 suggesting that the ribosome can bypass domain I and associate with the IRES between nucleotides between 140 and 200. However, AUAs placed at 140 and 200 did show decreases in translation compared with the wild type leader. These decreases in translation could indicate interference from improper structure formation or disruption of a protein binding site. An additional substitution of UUG was created at 140 and 200 and showed similar decreases in translation further indicating the sensitivity of these particular nucleotide locations. Interestingly, further downstream initiation codons as well as AUAs placed at 220 and 230, located -10 and -20 nucleotides from the main ORF, showed no decrease in translation (). The possibility that differential RNA stability confounded the interpretation of our results was ruled out by demonstrating that similar levels of transfected mRNA were obtained after semi-quantitative PCR (). Taken together, these experiments suggest that the ribosome lands in the middle of domain II (~170 nt) and either scans or bypasses the intervening sequence to arrive at the initiation codon. Alternatively, it is possible that the ribosome is directly recruited to the initiation start site.
Discussion
In this study we characterized the IRES located in the 5′ leader of the human tau mRNA. The entire 240 nucleotide sequence that comprises the minimal 5′ leader is required for any IRES activity. Moreover, the tau IRES is dependent upon secondary structure and is exceptionally sensitive to minute changes in sequence as small as one to three nucleotides. Finally, the 40S ribosome is predicted to land in the middle of the IRES, but whether it scans progressively to the initiation start site is unclear. Taken together, these results show an IRES that has similar characteristics to viral IRESs and as noted below may represent a novel group of eukaryotic IRESs.
Our approach to identifying cis-elements in the tau IRES is similar to those taken for viral IRESs, in vitro structural and mutational analysis.Citation17,Citation22,Citation56 Indeed, the 72% guanosine/cytosine composition of the tau IRES is equal or greater than that observed in many viral IRESs. Therefore, it was not surprising that the SHAPE analysis yielded an IRES that exhibits extensive secondary structure. It should be noted that there are limitations to this approach as in vitro transcribed RNA transfected directly into the cytoplasm may be structurally different than RNA transcripts generated through nuclear processes. However, an in vitro RNA approach permits the use of an A-cap structure and allows for the study of IRES and not cap-dependent translation mediated by the tau 5′ leader.
Overall, the tau IRES contains two major domains. Domain I is characterized by a large hairpin with multiple internal bulges and an extended stem loop. This result is supported by the loss of IRES activity from mutating a potential stem at the base of domain 1 (mut 10–14) and its partial recovery from the compensatory mutant (mut 10–14/195–199). A further distinguishing characteristic of domain 1 is the potential kissing hairpin or tertiary interaction between nucleotides 18–25 and 59–65. SHAPE analysis of the 18–25 and 59–65 mutant leaders reveal differential modification of the residues in the vicinity of the nucleotide substitution suggesting the previously constrained nucleotides are now accessible. Moreover, a decrease in IRES activity observed from each mutation (18–25 and 59–65) was rescued with the compensatory mutation indicating that a long range RNA-RNA interaction is possible. Finally, the rate at which the two mutants ran on the native gel was upheld by the SHAPE analysis. The mut 18–25 was predicted to be more compact and migrated faster than the wt leader while the mut 59–65 contained several open loops and a longer 3′ single stranded stretch which could contribute to the slower migration observed. Taken together, these results indicate the presence of a kissing hairpin interaction. We postulate that the formation of the hairpin may compact domain I allowing for canonical factor or ribosome binding, a feature observed in some viral IRESs.Citation16,Citation63,Citation64
Domain II is relatively structured at the 5′ end and becomes progressively less structured near the 3′ end or initiation codon. The formation of domain II appears to be linked with the correct folding of domain I. Structural analysis showed nucleotide substitutions in domain I (18–25 and 59–65) alter the folding of domain II significantly. However, maintaining the overall integrity of domain II does not appear to be as essential compared with domain I. For example, substituting nucleotides 135–37 or U136G located within a stem sub-region had little effect on IRES activity, whereas substituting nucleotides at 130–34 located within the loop of the same sub-region effectively reduced IRES activity. Similarly, substitutions made in another single stranded region 112–15 decreased IRES activity. These regions underline specific sequences that are necessary for IRES activity. The more flexible and single stranded characteristics of the 3′ end of domain II is a characteristic of many eukaryotic IRESs such as c-myc as well as some picornavirus IRESs.Citation31,Citation65 It is proposed that these single stranded regions may serve as landing pads for the ribosome, possibly positioning the ribosome on the start site. We postulate that the folding of domain II projects the extensive flexible single stranded sequences as a landing pad for canonical and non-canonical translation factors that in turn assist in ribosomal recruitment or positioning of the ribosome near the initiation codon.
In the tau IRES, our results indicate that the 40S ribosome is recruited to the unstructured segment in the middle of domain II, approximately 70 nucleotides upstream of the native initiation codon. Although, the ribosome is proposed to scan the final third of the leader, insertion of uORFs ten or twenty nucleotides upstream from the main ORF had no effect on the translation of the luciferase coding region. Though the two ORFs were in a proper Kozak context, it is possible that the ribosome could not read the start codon. Indeed, the uORF at 220 may have been obscured by the stem at 217–220. On the other hand, the structure of domain II may physically bring the segment at 170 and the 240 start site in close proximity allowing the ribosome to skip or jump the interceding nucleotides. A bypass event such as this has been shown to occur with Gtx elements that flank a large hairpin.Citation36 Alternatively, the ribosome may be recruited directly to the initiator codon as has been proposed for the Mnt and MTG8a eukaryotic IRESs.Citation66 These studies noted a similar phenomenon where uORFs close to the main coding region had no effect on translation.
Short complementary base pairing sequences between 18S rRNA and the eukaryotic IRES are proposed as an additional mechanism to recruit the ribosome.Citation36,Citation67 It is mechanistically analogous to the Shine-Dalgarno sequence in prokaryotes.Citation68 Supporting this hypothesis are several six to eight contiguous nucleotide segments that are complementary to 18S rRNA in domain II of the tau IRES (data not shown). Indeed, there are complementary sites near the two proposed ribosome binding sites: at 140–149 in the middle of domain II and at 234–240 near the initiation codon. Consequently, these sites could also contribute to positioning the ribosome to the vicinity of the initiator codon.
The ability of small and single nucleotide mutations to alter IRES activity is an uncommon feature for eukaryotic IRESs. For instance, four nucleotide mutations in a stem or apical loop of the c-myc IRES resulted in a minimal reduction and in some cases enhancement of IRES activity.Citation31 However, our analysis of the tau IRES revealed one to three nucleotide substitutions in domain II that reduced IRES activity by > 75–80%. Furthermore, two single nucleotide polymorphisms associated with Parkinson disease (C108T and a cytosine deletion at 199) dramatically reduced tau IRES activity alluding to a functional role of the tau IRES in disease development. There are additional eukaryotic IRESs in which activity is equally altered by small changes in nucleotide identity. In the URE2 IRES, altering a single nucleotide affects the stability of a stem, decreasing IRES activity.Citation69,Citation70 Larger mutations (5 nucleotides) flanking a stem in the cat-1 IRES also reduced translation.Citation32 Finally, mutations (1–5 nucleotides) including one SNP associated with the pathogenic development of Charcot-Marie-Tooth disease in the connexin-32 IRES were also shown to abolish IRES activity.Citation71,Citation72 The mechanism by which the small nucleotide changes in the tau 5′ leader exert a dramatic effect on IRES activity is unknown. Modeling the small nucleotides changes in the tau IRES suggests that the Δ199C could affect the stem comprising nucleotides 10–14 and 195–199, and mutating the entire stem is deleterious. The C108T substitution is not in a stem. However, it is possible that the nucleotide substitution affects the stability of this region.
The analysis of the tau IRES contributes to the hypothesis that eukaryotic IRESs can be classified on a continuum. At the most simple, small contiguous segments of ten to 50 nucleotides are sufficient to recruit the ribosome. Examples of this include the poly-A binding site in the Doudna yeast IRESs,Citation73 a double-stranded stem loop with a polypyrimidine tract as shown by Willis and colleagues,Citation27 and we have found the 5′ 50 nucleotides of the APP 5′ leader is sufficient to bind the La autoantigen and internally initiate translation (Beaudoin and Krushel unpublished observation). At the other end of the spectrum are large highly structured IRESs that require both structure and sequence specific motifs. These IRESs are more similar to viral IRESs and include the mKv1.4 and Mnt IRESs.Citation26,Citation66 The tau IRES is also included in this latter group.
In summary, the results from this study leads to the following hypothesis concerning how the tau IRES functions: domain I, which is highly structured, may form an impediment to ribosome scanning from the cap structure. Conversely, compacting domain I possibly through a kissing hairpin interaction exposes domain II for ribosomal recruitment along with ITAF and/or canonical factor binding. These factors then position the ribosome in an unstructured region in the middle of domain II or on the start site; nearby sites that are complementary to 40S rRNA may assist ribosome recruitment. Clearly, identifying the critical ITAFs/canonical factors that position the ribosome will be important for further elucidating the mechanism and concomitantly it will identify novel targets to regulate tau expression in AD and other tauopathies.
Materials and Methods
Constructs
The two potential tau 5′ leaders (240 nucleotides and 320 nucleotides) were PCR amplified from a human fetal brain Marathon®-Ready cDNA (Clontech cat# 639302) with gene specific primers (GenBank accession number: MAPT NM_001123066). The 5′ leaders were inserted into EcoRI and NcoI restriction sites between the Renilla luciferase and the Photinus luciferase cistrons in the dual luciferase vector-RP.Citation43 The dicistronic construct used for the in vitro translation assay was made from digesting the dual luciferase vector-RP with EcoRV and BamHI. The resulting Renilla and Photinus luciferase genes and SV40 3′ untranslated region (3′ UTR) were inserted into the EcoRV and BamHI site, in the multiple cloning site of the PBluescript II SK (+) vector downstream of the T7 promoter. The monocistronic luciferase construct for the in vitro translation assay was created by digesting the RP vector with EcoRI and BamHI and subcloning the Photinus ORF and SV40 3′ UTR into PBluescript II SK (+) downstream of the T7 promoter.
Truncations of the 240 nt leader were achieved with primers designed with EcoRI and NcoI restriction sites. The truncated PCR product was then sequenced and cloned into the monocistronic luciferase construct. Mutagenesis reactions were conducted using the QuikChange®XL Site-Directed mutagenesis kit (Agilent Technologies cat# 200521). Mutations were then sequenced and re-cloned into the monocistronic luciferase construct. For primer sequences refer to Table S1.
Cell culture and transfections
The neuroblastoma cell line SK-N-SH was obtained from ATCC and grown in standard conditions. The cell line was maintained in Modified Eagle’s Media (Cellgro MediaTech cat#15–010-CV) + 10% FBS+ 1% pencillin/streptomycin. Cell lines were validated by STR DNA fingerprinting using the AmpF_STR Identifiler kit according to manufacturer's instructions (Applied Biosystems cat#4322288). The STR profiles were compared with known ATCC fingerprints (ATCC.org), and to the Cell Line Integrated Molecular Authentication database (CLIMA) version 0.1.200808 (http://bioinformatics.istge.it/clima/) (Nucleic Acids Research 37:D925-D932 PMCID: PMC2686526). The STR profiles matched known DNA fingerprints or were unique.
For RNA transfections, cells were seeded at 2 × 105 in a 22.6 mm dish. One µg of monocistronic or dicistronic RNA was mixed in a 1:4 ratio with Enhancer, Buffer, and then Transmessenger reagent (Qiagen cat#301525) according to manufacturer’s specifications. The cells were washed with 1X PBS and 300µL of serum free media was added to each well. Cells were transfected for 4hrs at 37°C. Media was aspirated and cells were harvested by the addition 250 µL of 1x Passive Lysis Buffer (Promega cat#E1941) and rocked for 15 min at RT. Lysates were assayed for luciferase activity using the Dual-Luciferase Reporter Assay System (Promega cat#E1960) on a Luminoskan Ascent Luminometer (ThermoLabsystems).
RNA isolation and semi quantitative PCR
Following the RNA transfection, cells were harvested and lysed with 250 µL TRI Reagent® (Sigma Aldrich cat#93289–100 ML). Phase separation of the homogenized samples was done by the addition of 0.2 mL of chloroform, followed by agitation. RNA was extracted by centrifugation of the samples and removing the upper aqueous phase. The aqueous phase was further purified with the Pure Link total RNA isolation kit (Ambion cat#K156002). Equal amounts of total RNA were used for cDNA synthesis using ImProm-II™ Reverse Transcription kit (Promega cat#A3800). The following cDNA was then PCR amplified using primers directed against the Photinus ORF or to glyceraldehyde 3-phosphate dehydrogenase as control for 20 cycles.
In-vitro transcription
Plasmid DNA was linearized with either BamHI or NotI, and purified. One µg of linearized plasmid DNA was transcribed at 37°C for 3hrs. MEGAscript® T7 kit (Applied Biosystems/Ambion cat#AM1334) was used for in-vitro transcription reactions. Cap priming of messages with an A-cap was done by the addition of 3 µL 40mM G(5′)ppp(5′)A RNA cap structure analog (New England Biolabs Inc. cat# S1406L) and 2 µL 15 mM GTP to transcriptions. m7G capped RNAs were capped with ScriptCap™ m7G Capping system and all RNAs were tailed with the A-Plus™ Poly(A) Polymerase Tailing Kit (EPICENTRE Biotechnologies/CellScript, Inc. cat# SCCE0625, PAP5104H). RNA quality was verified by electrophoresis of 1 µg of RNA on a Reliant™ Precast RNA gel (LONZA cat# 54922) for 1.5 hrs and stained with SYBR® gold (Invitrogen cat# S11494). The RNA was then stored at -80°C.
Template preparation for SHAPE
RNA was created by PCR amplifying the 240 nt tau 5′ leader with the addition of the Hammerhead and Delta Ribozyme sequences added on the 5′ and 3′ end respectively with primers. Tau hammerhead 5′-ccgtgaggacgaaacggtacccggtaccgtccctcccctgggga-3′, Tau Delta ribozyme 5′-gcgccagcgaggaggctgggaccatgccgccccctggttcaaagttc-3′, Hammerhead primer 5′-taatacgactcactatagggagacgcg-3′, Delta Ribozyme primer 5′-ggtcccattcgccatgccgaagcatgt-3′. Transcription reaction protocol was adapted from Batey. 2001.Citation74 Transcription reactions contained 30 mM TRIS-HCl (pH 8.1), 3 mM spermidine, 10 mM dithiothreitol, 0.01% Triton X-100, 80 mM MgCl2, 4 mM each ribonucleotide 5′ triphosphate, 100 μg/ml DNA, 0.04 mg/ml T7 RNA polymerase and 80 units of RNAsin® Plus RNase Inhibitor (Promega cat# N2611). Transcriptions were incubated at 37°C for 4hrs. Transcriptions were precipitated through the addition of 3× volume of 100% ice cold EtOH, and centrifuged at 3,700rpm for 15 mins. The pellet was dried and resuspended in 3 mLs of 9 M Urea loading dye and loaded onto a 10% 7 M Urea slab gel for purification. The RNA was visualized via UV-shadowing and excised from the gel. The gel was chopped up in a 50 mL conical and eluted in 50 mLs of DEPC dH20 overnight at 4°C. The RNA was filtered from the gel pieces and concentrated by centrifugation through Amicon Ultra 30,000 MWM (Millipore cat# UFC903024) at 3,500 rpm for 15 min. 1 µg of the RNA was run on a 10% 7 M Urea gel and stained with EtBr to verify quality. The RNA was stored at -30 or -80°C.
Selective 2’hydroxyl acylation analyzed by primer extension (SHAPE)
N-methylisatoic anhydride (Invitrogen, Molecular Probes® cat# M25) was diluted in RNase free anhydrous Dimethyl sulfoxide (DMSO, Sigma Aldrich cat# 276855) to 65 mM or 30 mM. 6 pmols of RNA was brought to 12 µL volume with DEPC dH20. The RNA was heated to 85°C for 2 min and then bench top cooled for 2 min. 6 µL of the folding mix (333 mM HEPES, pH8.0, 20 mM MgCl2, 333 mM NaCl) was added and mixed. The reaction was incubated at 37°C for 5–10 min, simultaneously split into two tubes, one tube was used as a negative control. 1μL of the 65 mM or 30 mM NMIA or DMSO was added for an hour and incubated at 37°C. The reaction was desalted in Micro-Bio Spin 30 Chromatography column (BioRad cat#732–6223).
For primer extension assay, 1 µL of radiolabeled internal Δ primer was added and incubated at 65°C for 5 min, and then 35°C for 20 min. 6 µL of SHAPE enzyme mix (250 mM KCl, 167 mM TRIS HCl, pH 8.3, 1.67 mM each dNTP, 17 mM DTT) was added and tubes were heated for 1 min at 52°C. A half µL of Superscript® III Reverse Transcriptase (Invitrogen cat#18080–044) was added and heated at 52°C for 5 min. One µL NaOH was added, and heated at 95°C for 5 min. Twenty-nine µL of acid stop mix (1.6 mL 1M TRIS, 8.5 mL formamide, 0.5 mL 10× TBE, 1mL 0.5 M EDTA, 12.6 mg bromophenol blue and xylene cyanol) was then added, and incubated 5 min at 95°C. A sequencing ladder was made with 1 pmol RNA, 1 µL primer, sequencing enzyme mix (250mM KCl, 167 mM TRIS HCl, pH 8.3, 1.67mM dNTP,.167 mM dNTP (sequencing dNTP), 17mM DTT, 10 mM MgCl2), 1 µL dideoxy nucleotide stock 10 mM.
The radiolabeled cDNA fragments were visualized on a denaturing polyacrylamide sequencing gel (29:1 acrylamide:bis acrylamide, 1X TBE, 7M Urea). Ten µL of sample was run on the gel at 65 W for various lengths of time 1.5 h to longer in order to resolve bands close to the 5′end of the RNA. The gel was dried for 1–2 hrs and exposed to a phosphorimager screen overnight. Analysis of bands was done with ImageQuant® software. Absolute NMIA reactivity was calculated by subtracting untreated NMIA lane from the treated NMIA lane and plotted based on percentage of the highest modified residue. Modified nucleotides were identified by comparison to the sequencing ladder. Protocol was adapted from Wilkinson, 2006.Citation54
5′ end labeling SHAPE primer
Three to six µg of internal Δ primer was incubated with 2 µL of 10xPNK Buffer, 1 µL 32P γ-ATP (Perkin Elmer cat# BLU002Z001MC), 2 µL Polynucleotide Kinase 10 U/µL (PNK) (New England Biolabs Inc. cat# M0201S), and nuclease free water for a final volume of 20 μL. The reaction was incubated at 37°C for 1 h and then the reactions were desalted in Micro-Bio Spin 30 Chromatography columns. Twenty µL of urea loading dye (9 M Urea, 0.25 mg bromophenol blue and xylene cyanol in RNase-free dH2O) was added to the labeled primer and then loaded onto a 10% 7M urea-polyacrylamide gel (7 M Urea, 29:1 acrylamide to bisacrylamide, 1xTBE) and run for 1.5 h. The radioactive band was excised and eluted in 250 µL elution buffer (0.5 NaOAc pH 5.2, 0.1% SDS) overnight. The primer was then ethanol precipitated and resuspended to 30,000 counts per minute in RNase-free dH20.
Native gel
A 10% acrylamide solution with 10X TRIS/HEPES buffer (660 mM TRIS, 340 mM HEPES, pH 7.4) was prepared. MgCl2 was added to the acrylamide solution to bring the concentration to 2.5 mM. One µg of RNA was diluted in RNase-free dH2O and heated to 65°C for 3 min then cooled for 5 min. The sample was then added to 2× loading buffer (10 XTRIS/HEPES, 0.5 mg/mL bromophenol blue, 0.5 mg/mL xylene cyanol, 10% glycerol, 5 mM MgCl2 or 0.5M EDTA) and electrophoresed at 10 W for 8 hrs at 4°C. The gel was removed and stained with EtBr (100 µg/100 mL) for 15 min. Visualization of the gel was done on a UV transilluminator.
Abbreviations: | ||
IRES | = | internal ribosomal entry site |
ITAFs | = | IRES trans-acting factors |
uORF | = | upstream open reading frame |
UTR | = | untranslated region |
NMIA | = | N-methyl isatoic anhydride |
SHAPE | = | selective 2’hydroxyl acylation analyzed by primer extension |
Additional material
Download Zip (94.4 KB)Acknowledgments
The authors would like to acknowledge John Hammond, Megan Filbin and Jeff Kieft for their generous technical assistance. We also thank Kris Veo for his critical assistance, Jessica Tyler and Tara Dobson for critically reading our manuscript and insightful suggestions. STR DNA fingerprinting was done by the Cancer Center Support Grant-funded Characterized Cell Line core, NCI # CA016672. This work was supported by NIH grant R01 AG028156.
Disclosure of Potential Conflicts of Interest
No potential conflicts of interest were disclosed.
Supplemental Material
Supplemental material may be found here: http://www.landesbioscience.com/journals/rnabiology/article/22181
References
- Caceres A, Kosik KS. Inhibition of neurite polarity by tau antisense oligonucleotides in primary cerebellar neurons. Nature 1990; 343:461 - 3; http://dx.doi.org/10.1038/343461a0; PMID: 2105469
- Harada A, Oguchi K, Okabe S, Kuno J, Terada S, Ohshima T, et al. Altered microtubule organization in small-calibre axons of mice lacking tau protein. Nature 1994; 369:488 - 91; http://dx.doi.org/10.1038/369488a0; PMID: 8202139
- Feinstein SC, Wilson L. Inability of tau to properly regulate neuronal microtubule dynamics: a loss-of-function mechanism by which tau might mediate neuronal cell death. Biochim Biophys Acta 2005; 1739:268 - 79; http://dx.doi.org/10.1016/j.bbadis.2004.07.002; PMID: 15615645
- Köpke E, Tung YC, Shaikh S, Alonso AC, Iqbal K, Grundke-Iqbal I. Microtubule-associated protein tau. Abnormal phosphorylation of a non-paired helical filament pool in Alzheimer disease. J Biol Chem 1993; 268:24374 - 84; PMID: 8226987
- Lee G, Rook SL. Expression of tau protein in non-neuronal cells: microtubule binding and stabilization. J Cell Sci 1992; 102:227 - 37; PMID: 1400630
- Roberson ED, Scearce-Levie K, Palop JJ, Yan F, Cheng IH, Wu T, et al. Reducing endogenous tau ameliorates amyloid beta-induced deficits in an Alzheimer’s disease mouse model. Science 2007; 316:750 - 4; http://dx.doi.org/10.1126/science.1141736; PMID: 17478722
- Santacruz K, Lewis J, Spires T, Paulson J, Kotilinek L, Ingelsson M, et al. Tau suppression in a neurodegenerative mouse model improves memory function. Science 2005; 309:476 - 81; http://dx.doi.org/10.1126/science.1113694; PMID: 16020737
- Spires TL, Orne JD, SantaCruz K, Pitstick R, Carlson GA, Ashe KH, et al. Region-specific dissociation of neuronal loss and neurofibrillary pathology in a mouse model of tauopathy. Am J Pathol 2006; 168:1598 - 607; http://dx.doi.org/10.2353/ajpath.2006.050840; PMID: 16651626
- Gingras AC, Raught B, Sonenberg N. eIF4 initiation factors: effectors of mRNA recruitment to ribosomes and regulators of translation. Annu Rev Biochem 1999; 68:913 - 63; http://dx.doi.org/10.1146/annurev.biochem.68.1.913; PMID: 10872469
- Pestova TV, Kolupaeva VG, Lomakin IB, Pilipenko EV, Shatsky IN, Agol VI, et al. Molecular mechanisms of translation initiation in eukaryotes. Proc Natl Acad Sci USA 2001; 98:7029 - 36; http://dx.doi.org/10.1073/pnas.111145798; PMID: 11416183
- Belsham GJ. Jackson. R.J. Translation Initiation on Picornavirus RNA. Cold Spring Harbor: Cold Spring Harbor Laboratory Press, 2000.
- Jang SK, Pestova TV, Hellen CU, Witherell GW, Wimmer E. Cap-independent translation of picornavirus RNAs: structure and function of the internal ribosomal entry site. Enzyme 1990; 44:292 - 309; PMID: 1966843
- Honda M, Beard MR, Ping L-H, Lemon SM. A phylogenetically conserved stem-loop structure at the 5′ border of the internal ribosome entry site of hepatitis C virus is required for cap-independent viral translation. J Virol 1999; 73:1165 - 74; PMID: 9882318
- Kühn R, Luz N, Beck E. Functional analysis of the internal translation initiation site of foot-and-mouth disease virus. J Virol 1990; 64:4625 - 31; PMID: 2168956
- Fraser CS, Hershey JW, Doudna JA. The pathway of hepatitis C virus mRNA recruitment to the human ribosome. Nat Struct Mol Biol 2009; 16:397 - 404; http://dx.doi.org/10.1038/nsmb.1572; PMID: 19287397
- Kieft JS, Zhou K, Jubin R, Doudna JA. Mechanism of ribosome recruitment by hepatitis C IRES RNA. RNA 2001; 7:194 - 206; http://dx.doi.org/10.1017/S1355838201001790; PMID: 11233977
- Easton LE, Locker N, Lukavsky PJ. Conserved functional domains and a novel tertiary interaction near the pseudoknot drive translational activity of hepatitis C virus and hepatitis C virus-like internal ribosome entry sites. Nucleic Acids Res 2009; 37:5537 - 49; http://dx.doi.org/10.1093/nar/gkp588; PMID: 19596815
- Jan E, Sarnow P. Factorless ribosome assembly on the internal ribosome entry site of cricket paralysis virus. J Mol Biol 2002; 324:889 - 902; http://dx.doi.org/10.1016/S0022-2836(02)01099-9; PMID: 12470947
- López de Quinto S, Lafuente E, Martínez-Salas E. IRES interaction with translation initiation factors: functional characterization of novel RNA contacts with eIF3, eIF4B, and eIF4GII. RNA 2001; 7:1213 - 26; http://dx.doi.org/10.1017/S1355838201010433; PMID: 11565745
- Jang SK, Wimmer E. Cap-independent translation of encephalomyocarditis virus RNA: structural elements of the internal ribosomal entry site and involvement of a cellular 57-kD RNA-binding protein. Genes Dev 1990; 4:1560 - 72; http://dx.doi.org/10.1101/gad.4.9.1560; PMID: 2174810
- Psaridi L, Georgopoulou U, Varaklioti A, Mavromara P. Mutational analysis of a conserved tetraloop in the 5′ untranslated region of hepatitis C virus identifies a novel RNA element essential for the internal ribosome entry site function. FEBS Lett 1999; 453:49 - 53; http://dx.doi.org/10.1016/S0014-5793(99)00662-6; PMID: 10403373
- Fernández-Miragall O, Ramos R, Ramajo J, Martínez-Salas E. Evidence of reciprocal tertiary interactions between conserved motifs involved in organizing RNA structure essential for internal initiation of translation. RNA 2006; 12:223 - 34; http://dx.doi.org/10.1261/rna.2153206; PMID: 16373480
- Kafasla P, Morgner N, Pöyry TA, Curry S, Robinson CV, Jackson RJ. Polypyrimidine tract binding protein stabilizes the encephalomyocarditis virus IRES structure via binding multiple sites in a unique orientation. Mol Cell 2009; 34:556 - 68; http://dx.doi.org/10.1016/j.molcel.2009.04.015; PMID: 19524536
- Qin X, Sarnow P. Preferential translation of internal ribosome entry site-containing mRNAs during the mitotic cycle in mammalian cells. J Biol Chem 2004; 279:13721 - 8; http://dx.doi.org/10.1074/jbc.M312854200; PMID: 14739278
- Johannes G, Sarnow P. Cap-independent polysomal association of natural mRNAs encoding c-myc, BiP, and eIF4G conferred by internal ribosome entry sites. RNA 1998; 4:1500 - 13; http://dx.doi.org/10.1017/S1355838298981080; PMID: 9848649
- Jang GM, Leong LE, Hoang LT, Wang PH, Gutman GA, Semler BL. Structurally distinct elements mediate internal ribosome entry within the 5′-noncoding region of a voltage-gated potassium channel mRNA. J Biol Chem 2004; 279:47419 - 30; http://dx.doi.org/10.1074/jbc.M405885200; PMID: 15339906
- Spriggs KA, Mitchell SA, Willis AE. Investigation of interactions of polypyrimidine tract-binding protein with artificial internal ribosome entry segments. Biochem Soc Trans 2005; 33:1483 - 6; http://dx.doi.org/10.1042/BST20051483; PMID: 16246151
- Komar AA, Hatzoglou M. Internal ribosome entry sites in cellular mRNAs: mystery of their existence. J Biol Chem 2005; 280:23425 - 8; http://dx.doi.org/10.1074/jbc.R400041200; PMID: 15749702
- Chappell SA, Edelman GM, Mauro VP. A 9-nt segment of a cellular mRNA can function as an internal ribosome entry site (IRES) and when present in linked multiple copies greatly enhances IRES activity. Proc Natl Acad Sci USA 2000; 97:1536 - 41; http://dx.doi.org/10.1073/pnas.97.4.1536; PMID: 10677496
- Jopling CL, Spriggs KA, Mitchell SA, Stoneley M, Willis AE. L-Myc protein synthesis is initiated by internal ribosome entry. RNA 2004; 10:287 - 98; http://dx.doi.org/10.1261/rna.5138804; PMID: 14730027
- Le Quesne JP, Stoneley M, Fraser GA, Willis AE. Derivation of a structural model for the c-myc IRES. J Mol Biol 2001; 310:111 - 26; http://dx.doi.org/10.1006/jmbi.2001.4745; PMID: 11419940
- Yaman I, Fernandez J, Liu H, Caprara M, Komar AA, Koromilas AE, et al. The zipper model of translational control: a small upstream ORF is the switch that controls structural remodeling of an mRNA leader. Cell 2003; 113:519 - 31; http://dx.doi.org/10.1016/S0092-8674(03)00345-3; PMID: 12757712
- Beaudoin ME, Poirel VJ, Krushel LA. Regulating amyloid precursor protein synthesis through an internal ribosomal entry site. Nucleic Acids Res 2008; 36:6835 - 47; http://dx.doi.org/10.1093/nar/gkn792; PMID: 18953033
- Dobson T, Minic A, Nielsen K, Amiott E, Krushel L. Internal initiation of translation of the TrkB mRNA is mediated by multiple regions within the 5′ leader. Nucleic Acids Res 2005; 33:2929 - 41; http://dx.doi.org/10.1093/nar/gki605; PMID: 15908588
- Zhang W, Wang X, Xiao Z, Liu W, Chen B, Dai J. Mapping of the minimal internal ribosome entry site element in the human embryonic stem cell gene OCT4B mRNA. Biochem Biophys Res Commun 2010; 394:750 - 4; http://dx.doi.org/10.1016/j.bbrc.2010.03.064; PMID: 20230781
- Chappell SA, Dresios J, Edelman GM, Mauro VP. Ribosomal shunting mediated by a translational enhancer element that base pairs to 18S rRNA. Proc Natl Acad Sci USA 2006; 103:9488 - 93; http://dx.doi.org/10.1073/pnas.0603597103; PMID: 16769881
- Mitchell SA, Brown EC, Coldwell MJ, Jackson RJ, Willis AE. Protein factor requirements of the Apaf-1 internal ribosome entry segment: roles of polypyrimidine tract binding protein and upstream of N-ras. Mol Cell Biol 2001; 21:3364 - 74; http://dx.doi.org/10.1128/MCB.21.10.3364-3374.2001; PMID: 11313462
- Sadot E, Heicklen-Klein A, Barg J, Lazarovici P, Ginzburg I. Identification of a tau promoter region mediating tissue-specific-regulated expression in PC12 cells. J Mol Biol 1996; 256:805 - 12; http://dx.doi.org/10.1006/jmbi.1996.0126; PMID: 8601831
- Andreadis A, Wagner BK, Broderick JA, Kosik KS. A tau promoter region without neuronal specificity. J Neurochem 1996; 66:2257 - 63; http://dx.doi.org/10.1046/j.1471-4159.1996.66062257.x; PMID: 8632146
- Heicklen-Klein A, Ginzburg I. Tau promoter confers neuronal specificity and binds Sp1 and AP-2. J Neurochem 2000; 75:1408 - 18; http://dx.doi.org/10.1046/j.1471-4159.2000.0751408.x; PMID: 10987820
- Veo BL, Krushel LA. Translation initiation of the human tau mRNA through an internal ribosomal entry site. J Alzheimers Dis 2009; 16:271 - 5; PMID: 19221416
- Maloney B, Lahiri DK. Structural and functional characterization of H2 haplotype MAPT promoter: unique neurospecific domains and a hypoxia-inducible element would enhance rationally targeted tauopathy research for Alzheimer’s disease. Gene 2012; 501:63 - 78; http://dx.doi.org/10.1016/j.gene.2012.01.049; PMID: 22310385
- Stoneley M, Chappell SA, Jopling CL, Dickens M, MacFarlane M, Willis AE. c-Myc protein synthesis is initiated from the internal ribosome entry segment during apoptosis. Mol Cell Biol 2000; 20:1162 - 9; http://dx.doi.org/10.1128/MCB.20.4.1162-1169.2000; PMID: 10648601
- Mihailovich M, Thermann R, Grohovaz F, Hentze MW, Zacchetti D. Complex translational regulation of BACE1 involves upstream AUGs and stimulatory elements within the 5′ untranslated region. Nucleic Acids Res 2007; 35:2975 - 85; http://dx.doi.org/10.1093/nar/gkm191; PMID: 17439957
- Rogers GW Jr., Edelman GM, Mauro VP. Differential utilization of upstream AUGs in the beta-secretase mRNA suggests that a shunting mechanism regulates translation. Proc Natl Acad Sci USA 2004; 101:2794 - 9; http://dx.doi.org/10.1073/pnas.0308576101; PMID: 14981268
- Lockard RE, Lane C. Requirement for 7-methylguanosine in translation of globin mRNA in vivo. Nucleic Acids Res 1978; 5:3237 - 47; http://dx.doi.org/10.1093/nar/5.9.3237; PMID: 212716
- Gingras AC, Svitkin Y, Belsham GJ, Pause A, Sonenberg N. Activation of the translational suppressor 4E-BP1 following infection with encephalomyocarditis virus and poliovirus. Proc Natl Acad Sci USA 1996; 93:5578 - 83; http://dx.doi.org/10.1073/pnas.93.11.5578; PMID: 8643618
- Bergamini G, Preiss T, Hentze MW. Picornavirus IRESes and the poly(A) tail jointly promote cap-independent translation in a mammalian cell-free system. RNA 2000; 6:1781 - 90; http://dx.doi.org/10.1017/S1355838200001679; PMID: 11142378
- Dehlin E, Wormington M, Körner CG, Wahle E. Cap-dependent deadenylation of mRNA. EMBO J 2000; 19:1079 - 86; http://dx.doi.org/10.1093/emboj/19.5.1079; PMID: 10698948
- Andreev DE, Dmitriev SE, Terenin IM, Prassolov VS, Merrick WC, Shatsky IN. Differential contribution of the m7G-cap to the 5′ end-dependent translation initiation of mammalian mRNAs. Nucleic Acids Res 2009; 37:6135 - 47; http://dx.doi.org/10.1093/nar/gkp665; PMID: 19696074
- Holcik M, Yeh C, Korneluk RG, Chow T. Translational upregulation of X-linked inhibitor of apoptosis (XIAP) increases resistance to radiation induced cell death. Oncogene 2000; 19:4174 - 7; http://dx.doi.org/10.1038/sj.onc.1203765; PMID: 10962579
- Young RM, Wang SJ, Gordan JD, Ji X, Liebhaber SA, Simon MC. Hypoxia-mediated selective mRNA translation by an internal ribosome entry site-independent mechanism. J Biol Chem 2008; 283:16309 - 19; http://dx.doi.org/10.1074/jbc.M710079200; PMID: 18430730
- Merino EJ, Wilkinson KA, Coughlan JL, Weeks KM. RNA structure analysis at single nucleotide resolution by selective 2′-hydroxyl acylation and primer extension (SHAPE). J Am Chem Soc 2005; 127:4223 - 31; http://dx.doi.org/10.1021/ja043822v; PMID: 15783204
- Wilkinson KA, Merino EJ, Weeks KM. Selective 2′-hydroxyl acylation analyzed by primer extension (SHAPE): quantitative RNA structure analysis at single nucleotide resolution. Nat Protoc 2006; 1:1610 - 6; http://dx.doi.org/10.1038/nprot.2006.249; PMID: 17406453
- McGinnis JL, Duncan CD, Weeks KM. High-throughput SHAPE and hydroxyl radical analysis of RNA structure and ribonucleoprotein assembly. Methods Enzymol 2009; 468:67 - 89; http://dx.doi.org/10.1016/S0076-6879(09)68004-6; PMID: 20946765
- Kieft JS, Zhou K, Jubin R, Murray MG, Lau JY, Doudna JA. The hepatitis C virus internal ribosome entry site adopts an ion-dependent tertiary fold. J Mol Biol 1999; 292:513 - 29; http://dx.doi.org/10.1006/jmbi.1999.3095; PMID: 10497018
- Zuker M. Mfold web server for nucleic acid folding and hybridization prediction. Nucleic Acids Res 2003; 31:3406 - 15; http://dx.doi.org/10.1093/nar/gkg595; PMID: 12824337
- Arima K, Hirai S, Sunohara N, Aoto K, Izumiyama Y, Uéda K, et al. Cellular co-localization of phosphorylated tau- and NACP/alpha-synuclein-epitopes in lewy bodies in sporadic Parkinson’s disease and in dementia with Lewy bodies. Brain Res 1999; 843:53 - 61; http://dx.doi.org/10.1016/S0006-8993(99)01848-X; PMID: 10528110
- Healy DG, Abou-Sleiman PM, Lees AJ, Casas JP, Quinn N, Bhatia K, et al. Tau gene and Parkinson’s disease: a case-control study and meta-analysis. J Neurol Neurosurg Psychiatry 2004; 75:962 - 5; http://dx.doi.org/10.1136/jnnp.2003.026203; PMID: 15201350
- Evans W, Fung HC, Steele J, Eerola J, Tienari P, Pittman A, et al. The tau H2 haplotype is almost exclusively Caucasian in origin. Neurosci Lett 2004; 369:183 - 5; http://dx.doi.org/10.1016/j.neulet.2004.05.119; PMID: 15464261
- Kwok JB, Teber ET, Loy C, Hallupp M, Nicholson G, Mellick GD, et al. Tau haplotypes regulate transcription and are associated with Parkinson’s disease. Ann Neurol 2004; 55:329 - 34; http://dx.doi.org/10.1002/ana.10826; PMID: 14991810
- Tobin JE, Latourelle JC, Lew MF, Klein C, Suchowersky O, Shill HA, et al. Haplotypes and gene expression implicate the MAPT region for Parkinson disease: the GenePD Study. Neurology 2008; 71:28 - 34; http://dx.doi.org/10.1212/01.wnl.0000304051.01650.23; PMID: 18509094
- Lomakin IB, Hellen CU, Pestova TV. Physical association of eukaryotic initiation factor 4G (eIF4G) with eIF4A strongly enhances binding of eIF4G to the internal ribosomal entry site of encephalomyocarditis virus and is required for internal initiation of translation. Mol Cell Biol 2000; 20:6019 - 29; http://dx.doi.org/10.1128/MCB.20.16.6019-6029.2000; PMID: 10913184
- Borovjagin A, Pestova T, Shatsky I. Pyrimidine tract binding protein strongly stimulates in vitro encephalomyocarditis virus RNA translation at the level of preinitiation complex formation. FEBS Lett 1994; 351:299 - 302; http://dx.doi.org/10.1016/0014-5793(94)00848-5; PMID: 8082784
- Martínez-Salas E, Fernández-Miragall O. Picornavirus IRES: structure function relationship. Curr Pharm Des 2004; 10:3757 - 67; http://dx.doi.org/10.2174/1381612043382657; PMID: 15579069
- Mitchell SA, Spriggs KA, Bushell M, Evans JR, Stoneley M, Le Quesne JP, et al. Identification of a motif that mediates polypyrimidine tract-binding protein-dependent internal ribosome entry. Genes Dev 2005; 19:1556 - 71; http://dx.doi.org/10.1101/gad.339105; PMID: 15998809
- Chappell SA, Edelman GM, Mauro VP. Ribosomal tethering and clustering as mechanisms for translation initiation. Proc Natl Acad Sci USA 2006; 103:18077 - 82; http://dx.doi.org/10.1073/pnas.0608212103; PMID: 17110442
- Shine J, Dalgarno L. Determinant of cistron specificity in bacterial ribosomes. Nature 1975; 254:34 - 8; http://dx.doi.org/10.1038/254034a0; PMID: 803646
- Reineke LC, Komar AA, Caprara MG, Merrick WC. A small stem loop element directs internal initiation of the URE2 internal ribosome entry site in Saccharomyces cerevisiae. J Biol Chem 2008; 283:19011 - 25; http://dx.doi.org/10.1074/jbc.M803109200; PMID: 18460470
- Reineke LC, Merrick WC. Characterization of the functional role of nucleotides within the URE2 IRES element and the requirements for eIF2A-mediated repression. RNA 2009; 15:2264 - 77; http://dx.doi.org/10.1261/rna.1722809; PMID: 19861427
- Hudder A, Werner R. Analysis of a Charcot-Marie-Tooth disease mutation reveals an essential internal ribosome entry site element in the connexin-32 gene. J Biol Chem 2000; 275:34586 - 91; http://dx.doi.org/10.1074/jbc.M005199200; PMID: 10931843
- Kabzińska D, Kotruchow K, Ryniewicz B, Kochański A. Two pathogenic mutations located within the 5′-regulatory sequence of the GJB1 gene affecting initiation of transcription and translation. Acta Biochim Pol 2011; 58:359 - 63; PMID: 21918739
- Gilbert WV, Zhou K, Butler TK, Doudna JA. Cap-independent translation is required for starvation-induced differentiation in yeast. Science 2007; 317:1224 - 7; http://dx.doi.org/10.1126/science.1144467; PMID: 17761883
- Batey RT, Sagar MB, Doudna JA. Structural and energetic analysis of RNA recognition by a universally conserved protein from the signal recognition particle. J Mol Biol 2001; 307:229 - 46; http://dx.doi.org/10.1006/jmbi.2000.4454; PMID: 11243816