Abstract
The hok/sok toxin-antitoxin system of Escherichia coli plasmid R1 increases plasmid maintenance by killing plasmid-free daughter cells. The hok/sok locus specifies two RNAs: hok mRNA, which encodes a toxic transmembrane protein, and sok antisense RNA, which binds a complementary region in the hok mRNA and induces transcript degradation. During cell growth, the cis-encoded sok RNA inhibits expression of the Hok toxin. In plasmid-free segregants, the rapid decay of sok RNA relative to hok mRNA permits Hok translation, leading to cell death. This post-segregational killing mechanism relies upon the ability of the hok mRNA to adopt alternative structural configurations, which affect ease of translation and the susceptibility of the molecule to degradation. The full-length hok transcript is stable, highly structured and immune to ribosome and antisense RNA binding. Gradual 3′ end processing produces dramatic structural rearrangements in the mRNA, which render the molecule translationally active and expose the sok RNA binding site. During transcription, premature ribosome and sok binding are prevented through the formation of transient metastable hairpins in the 5′ end of the nascent transcript. Several hok mRNA paralogs have been identified in the genome of E. coli, and Hok protein orthologs found in the genomes of Enterobacteria. Using a combination of automated search and extensive manual editing, we compiled a multiple sequence alignment for the hok mRNA. All three experimentally validated hok mRNA structures are mapped onto this alignment, which has been submitted to the Rfam database for RNA families.
Introduction
The hok/sok toxin-antitoxin system of Escherichia coli plasmid R1
The hok/sok locus of E. coli plasmid R1 mediates plasmid stability through a mechanism known as post-segregational killing (PSK).Citation1,Citation2 This toxin-antitoxin system features three partially overlapping genes.Citation3 The “host-killing” hok gene encodes a toxic transmembrane protein of 52 amino acids.Citation4 Upon translation, the Hok protein disrupts the E. coli plasma membrane, inducing cell death.Citation4 The “modulation of killing” mok gene partially overlaps the hok gene, and the two are transcribed as a single mRNA.Citation3 Translation of the two genes is coupled, such that the mok reading frame must first be translated before translation of the hok reading frame can take place.Citation3 The cis-encoded “suppression of killing” sok gene produces an RNA antisense to the hok/mok mRNA that blocks mok translation, thereby indirectly inhibiting Hok protein expression.Citation3 For simplicity, the hok/mok mRNA shall hereby be referred to as the hok mRNA. Plasmid stabilization depends upon two system characteristics: (1) the preferential decay of sok antisense RNA relative to hok mRNA, and (2) alternative hok mRNA structural conformations which affect the accessibility of the transcript to both the ribosome and sok antisense binding.Citation3
Mechanism for plasmid preservation
With a half-life of approximately 30 sec, sok RNA is highly unstable.Citation5 In contrast, hok mRNA is very stable with a half-life of about 20 min.Citation5 During cell growth, transcription of sok RNA is driven by a strong promotor, such that it is produced in considerable molar excess relative to hok mRNA.Citation5,Citation6 Upon binding, the hok mRNA-sok RNA duplex is rapidly cleaved by RNase III, thereby preventing hok expression in cells possessing the R1 plasmid.Citation7 Following cell division, sok RNA is rapidly degraded in any plasmid-free daughter cell so that mok and hok translation is permitted, and cell death is induced.Citation5 Thus, the hok/sok toxin-antitoxin system promotes plasmid maintenance.
Role of alternative RNA structures
Hok mRNA exists in two forms: (1) as a stable and inactive full-length transcript, and (2) as an unstable and active truncated mRNACitation5 (). The full-length hok transcript adopts an inert, highly structured conformation that is inaccessible to both ribosome binding and sok antisense suppression.Citation5 Translation of mok and hok is dependent upon nucleotides +1 to +7 in the most 5′ end of the transcript, termed the translational activator element (tac).Citation8 In the full-length transcript, this region is sequestered through binding to a fold-back inhibitory element (fbi) in the 3′ end of the mRNA.Citation8 The fbi-tac stem renders the transcript translationally inactive, and has been demonstrated to slow transcript processing, contributing to the molecule's longevity.Citation8 Similarly, the mok Shine-Dalgarno (SD) sequence is sequestered by pairing to an upstream region termed the upstream complementary box (ucb), preventing ribosome entry.Citation9 Finally, in the full-length transcript the target site for sok antisense binding (SokT) is also sequestered, rendering the mRNA immune to degradation through sok antisense binding.Citation9
Figure 1. Arc plot of the mRNA for the Hok and Mok proteins, featuring the reference sequence from Escherichia coli plasmid R1, along with known structure motifs. Every arc represents a base-pair between the corresponding sequence positions. The legend at right corresponds to the arc colors, and indicates the structural conformation (active, inactive, or transient) in which the base-pair is found. The legend at left elucidates the identity of the nucleobases in the reference sequence. Annotated black lines indicate the positions of notable sequence motifs: tac, translational activator element; ucb, upstream complementary box; dcb, downstream complementary box; mok SD, mok Shine-Dalgarno sequence; hok SD, hok Shine-Dalgarno sequence; fbi, fold-back inhibitory element. Black horizontal arrows delineate the positions of the hok and mok protein-coding regions. Note that the sok antisense RNA is complementary to the region indicated by the gray horizontal arrow and not part of the hok/mok mRNA. The vertical black arrow denotes the position of the 3′ cleavage site. Figure generated using the R-chie program.Citation29
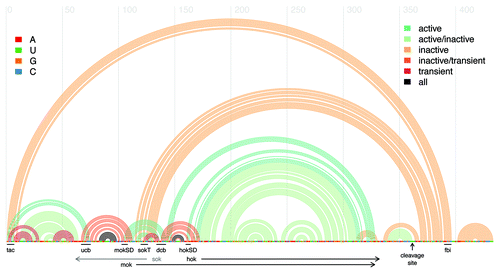
Transcript maturation is initiated through the cleavage of 39 nt from the 3′ end of the full-length hok mRNA by the enzymes RNase II and PNPase.Citation9 The sequence is truncated upstream of the fbi element, triggering substantial structural rearrangements in the 5′ end of the molecule.Citation9 In the truncated mRNA, the tac element pairs with the ucb sequence, while the mok SD sequence pairs with a downstream complementary box (dcb) that is an almost perfect duplicate of the ucb.Citation9 In this conformation, both the tac element and mok SD are accessible to ribosome binding, rendering the truncated transcript translationally active.Citation9 Binding of the mok SD and the dcb also produces a hairpin whose unpaired loop region exposes the 7 nt sok target sequence, rendering the mature transcript vulnerable to degradation through sok antisense binding.Citation9
The presence of the highly-structured inactive hok mRNA is critical to the killing mechanism.Citation5 In the growing cell, mature transcripts are rapidly suppressed and degraded, while inert full-length transcripts may accumulate to form a pool of hok mRNA that may be passed on to segregants.Citation5 In plasmid-free daughter cells, sok RNA is rapidly degraded while full-length hok mRNA is gradually processed and translated, resulting in cell death.Citation5 The secondary structures of both the inactive and active forms of hok have been experimentally mapped.Citation9 In addition, transient structures in the co-transcriptional folding pathway of the hok mRNA were predicted using a genetic algorithm, and later verified through mutational analyses.Citation10,Citation11
Evidence for alternative active and inactive conformations
Initial structural mapping of the inactive full-length hok mRNA from plasmid R1 was conducted using a combination of structural probing (DMS and CMCT modification; cleavage by nucleases T1, T2 and V1), computational prediction (using the Mfold program packageCitation12) and mutational analysis.Citation13 This initial structure was refined in subsequent experiments.Citation13 Franch and Gerdes (1996) established the role of the fbi-tac stem through mutational analysis.Citation8 The structural rearrangements triggered by 3′ end processing of the full-length mRNA where investigated through structural probing (DMS modification; cleavage by nucleases T2 and V1) and mutation.Citation9 Finally, Franch, Gultyaev and Gerdes (1997) presented a refined structural configuration for the full-length inactive hok mRNA, proposed a structure for the truncated active hok mRNA and established the role of the tac-ucb stem and mok SD-dcb stem in the active hok structure.Citation9
Evidence for transient co-transcriptional structures
The inhibitory fbi-tac stem preserves the full-length hok transcript in an inactive state by preventing the formation of the tac-ucb stem. During transcription, the tac element (which lies in the extreme 5′ end of the molecule) is synthesized long before its 3′ fbi pairing partner. This raises the question of how the tac element is sequestered—and ribosome or sok binding prevented—during transcript synthesis. Gultyaev, Franch and Gerdes (1997) sought to answer this question by simulating the co-transcriptional folding pathways of seven hok homologs using a genetic algorithm.Citation10 Their simulations suggested that as the toxin-encoding mRNA is transcribed, the tac element pairs with a complementary region immediately downstream, forming a metastable transient hairpin.Citation10 This local hairpin appears to persist until the simulated completion of transcription, preventing the formation of the tac-ucb stem (required for ribosome and sok binding). The formation of the 5′ hairpin favored the formation of additional transient local hairpins; simulations predicted that the mok and hok SD sequences are sequestered in the nascent transcript through binding with the ucb and dcb elements, respectively.Citation10 The genetic algorithm predicted the formation of the fbi-tac stem following completion of transcription; furthermore, simulation of 3′ end processing predicted structural reconfiguration and the formation of the active hok mRNA structure. Thus, several hok mRNA sequence elements were predicted to possess multiple pairing partners. Alignment of five of the homologs examined revealed the presence of nucleotide covariations in all proposed hairpins. Furthermore, the authors identified multiple instances of bases that covary such that pairing with multiple partners is preserved, and defined the term “coupled nucleotide covariations” to denote such instances.Citation10 Subsequent structural probing and mutational analyses supported the existence of the metastable transient hairpin.Citation11 As expected, mutations that destabilize the proposed hairpin result in rapid cleavage of the hok transcript in the presence of sok RNA, while stabilizing mutations result in reduced rates of antisense RNA binding and protein translation.Citation11
Paralogs in Escherichia coli
The hok/sok locus encoded by E. coli plasmid R1 has been the focus of experimental investigation.Citation5 Several Hok protein paralogs have since been discovered in the E. coli genome.Citation14 These include the plasmid-encoded flm, srnB and pnd genes, as well as five chromosome-encoded genes.Citation14 The flm gene found on plasmid F features a nucleotide sequence nearly identical to hok (94% identity).Citation15 An alignment of the two sequences indicates conservation of crucial structural components, and the function of this gene in maintenance of the F-plasmid through post-segregational killing has been experimentally confirmed.Citation15 The srnB gene, also found on plasmid F, and the pnd genes of plasmids R483 and R16, are more distantly related to hok.Citation14 SrnB and pnd have been shown to encode stable mRNAs and unstable antisense RNAs, and to mediate plasmid stabilization by inducing membrane damage.Citation16 Five hok homologs exist on the chromosome of E. coli K-1217. Of these, three are inactivated by insertion elements (hokA, hokC or gef and hokE), one by a point mutation (hokB) and the fifth by a major rearrangement (hokD).Citation17 With the exception of hokB all chromosomal K-12 hok homologs are also missing critical sequence or structural elements, such as the start codon for the mok-homologous reading frame.Citation17 Intact hok homologs have been found on the chromosomes of other strains of E. coli.Citation17 While these homologs possess all known sequence and structure motifs required for hok function, they fail to mediate post-segregational killing when cloned unto a plasmidCitation17; the function of these genes is not known. Orthologs in related species have also been discovered.Citation18
Orthologs in Enterobacteria
Hok protein orthologs are confined to Gammaproteobacteria, namely Enterobacteria and a few species in closely related families.Citation18 Faridani et al. (2006) conducted a BLAST search of the genomes of approximately 200 prokaryotic species and found that multiple hok-homologous proteins existed in the genomes of several enteric bacteria, including those of the genera Escherichia, Salmonella, Shigella, Photorhabdus and Erwinia.Citation18 Homologs were also identified in the genome of Vibrio vulnificus, a closely related species not classified among enteric bacteria.Citation18 Our analysis, which utilizes RNA sequences along with secondary structure information in place of a protein-BLAST search, features hok homologs from several additional species.
Purpose
The Rfam database of RNA familiesCitation19 features an alignment for the sok antisense RNA (RF01794), but lacks an alignment for the hok mRNA. We aim to ameliorate this by compiling an annotated alignment for the entire hok transcript using a combination of automated search and extensive manual editing. The formation of alternative structures is critical to the function of the hok/sok toxin-antitoxin system, and transient intermediates in the co-transcriptional folding pathway of the hok mRNA have been experimentally probed. We therefore set out to map all three experimentally examined structures onto the hok alignment.
Results
Hok mRNA multiple sequence alignment
A reference hok mRNA sequence derived from the Escherichia coli plasmid R1, along with the experimentally validated structure of the active transcript, were utilized to build a covariance model using the Infernal program.Citation20 This was used to search the complete RefSeq genomes of Gammaproteobacteria derived from NCBI Nucleotide.Citation21 Sequence hits that met selection criteria were mapped to the covariance model, and the alignment was extensively manually edited using the 4sale editing program.Citation22 The structure of the inactive transcript was then manually mapped onto this initial alignment in order to build a second covariance model. Additional sequence fragments derived from NCBI Nucleotide were searched with this model to expand the diversity of species included.Citation21 The resulting alignment is 515 characters in length and is comprised of 29 sequences. The average pairwise sequence identity is 79%, the fraction of gaps 0.15 and the average sequence length 439 nt.
Alternative structure mapping
Three structures were mapped onto the hok mRNA alignment: the active structure of the truncated hok transcript, the inactive structure of the full-length hok transcript, and transient helices in the co-transcriptional folding pathway of the mRNA. Alignment properties relating to each of the three structures are presented in . Arc diagrams and covariance plots for the active and inactive conformations are presented in , specifying the degree of conservation, covariation and invalid mutations found in the alignment.
Table 1. Alignment properties relating to the three mapped structures: the active structure of the truncated hok mRNA, the inactive structure of the full-length mRNA and the transient upstream structures which form during hok transcription
Figure 2. Double covariance plot and arc diagram for the alignment of the hok mRNA. The top covariance plot and arc diagram represents the active hok structure. Every arc represents a base-pair between the two respective columns of the alignment. For each pair of alignment columns in the structure, the bases of each sequence are colored according to their status, as indicated in the legend at right: green denotes conservation of the most common ordered base-pair in the pair of columns, dark blue indicates that the bases in both columns differ from the most common pair but retain base-pairing potential, light blue denotes a one-sided mutation in which base-pairing is retained, orange denotes the presence of mutations which invalidate base-pairing, black indicates nucleotides not involved in base-pairing and gray indicates the presence of alignment gaps. The lower covariance plot and arc diagram represent the inactive hok structure. Finally, the arc colors (presented in the legend at left) indicate the fraction of canonical base-pairs in the corresponding columns: red, 0–25%; orange, 25–50%; light blue 50–75%; dark blue, 75–100%. Figure generated using the R-chie program.Citation29
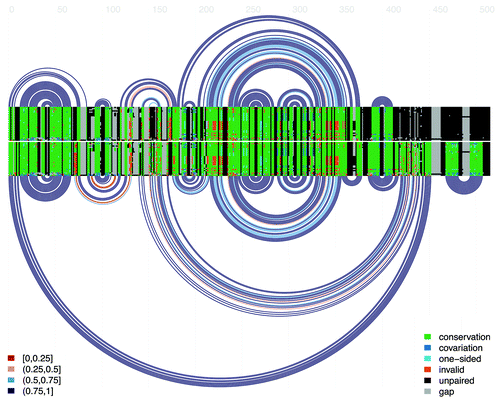
Conclusions
The hok/sok locus mediates plasmid stability through a complex multi-step mechanism that features three alternative structural configurations.Citation10 The high percentage of canonical base-pairs for all three structures in the alignment for the hok mRNA family suggests that these alternative configurations are highly conserved among different E. coli strains and closely related Enterobacteria.
The presence of two alternative structures is critical to the function of many non-coding RNAs, including transcription attenuators and riboswitches.Citation23,Citation24 The formation of co-transcriptional transient helices has been shown to facilitate the folding of several non-coding RNAs, including the hepatitis delta virus ribozyme, ribonuclease P RNA, bacterial transfer-messenger RNA and the bacterial signal recognition particle RNA.Citation25,Citation26 Due to the prevalence and importance of transient and alternative structures in non-coding RNAs, future contributors to the Rfam database may consider further expanding the number of alignments featuring multiple structural annotations.
Materials and Methods
Reference sequence and structures
The hok mRNA sequence from plasmid R1 of E. coli strain CSH50 was used as the reference sequence (accession number X05813.1, start and end coordinates 571–1011). Consensus structures for the full-length, truncated and nascent transcript were derived from the literature and mapped to the reference sequence.Citation9,Citation10,Citation27 The reference sequence and structure of the active hok transcript where utilized to build and calibrate a covariance model using the Infernal program.Citation20
Initial alignment compilation
The covariance model based on the E. coli reference sequence and active structure was utilized to search a database consisting of all complete RefSeq genomes for Gammaproteobacteria derived from NCBI Nucleotide.Citation21 The search was confined to Gammaproteobacterial genomes as previous analyses indicate that the hok gene is confined to Enterobacteria and a few closely related species.Citation18 The Rfam alignment for the sok RNA family,Citation19 as well as the Pfam alignment for the Hok protein,Citation28 both reflect this. Of the resulting hits, only sequences which met the following criteria were retained: the hit spanned the entire length of the reference sequence (non-local), possessed all critical sequence motifs and was not missing notable structural features. All sequences that met these criteria were aligned using our covariance model, along with the reference. The resulting alignment was extensively manually edited using the alignment editing program 4sale.Citation22 These initial alignments, along with the structure of the inactive transcript, were utilized to build a second covariance model using Infernal.
Expanded alignment compilation
A second Infernal search was undertaken in order to include species with annotated hok genes in the alignment. A search for species featuring annotated Hok proteins was conducted in NCBI.Citation21 For each species not yet included in the alignment, a genome fragment spanning the 1000 nucleotides upstream and downstream of the protein-coding region was extracted. In addition, we extracted the entire genomes of species which did not feature Hok protein annotations in NCBI but which are cited in the literature as featuring the hok gene.Citation18 The covariance model based on the initial alignment and inactive structure was utilized to search these sequences. Once again, we retained all hits that spanned the length of the alignment and included all critical sequence and structure motifs. These sequences were mapped to the initial alignment using the covariance model, and extensive manual editing was once again undertaken in 4sale to generate the final, expanded alignment.
Additional material
Download Zip (32.8 KB)Acknowledgments
This project was supported by grants to I.M.M. from the Natural Sciences and Engineering Research Council (NSERC) of Canada and from the Canada Foundation for Innovation (CFI). A.S. holds an NSERC Undergraduate Student Research Award from the Graduate Program in Genome Science and Technology at the University of British Columbia.
Disclosure of Potential Conflicts of Interest
No potential conflicts of interest were disclosed.
References
- Gerdes K, Larsen JE, Molin S. Stable inheritance of plasmid R1 requires two different loci. J Bacteriol 1985; 161:292 - 8; PMID: 2981804
- Gerdes K, Rasmussen PB, Molin S. Unique type of plasmid maintenance function: postsegregational killing of plasmid-free cells. Proc Natl Acad Sci U S A 1986; 83:3116 - 20; http://dx.doi.org/10.1073/pnas.83.10.3116; PMID: 3517851
- Thisted T, Gerdes K. Mechanism of post-segregational killing by the hok/sok system of plasmid R1. Sok antisense RNA regulates hok gene expression indirectly through the overlapping mok gene. J Mol Biol 1992; 223:41 - 54; http://dx.doi.org/10.1016/0022-2836(92)90714-U; PMID: 1370544
- Gerdes K, Bech FW, Jørgensen ST, Løbner-Olesen A, Rasmussen PB, Atlung T, et al. Mechanism of postsegregational killing by the hok gene product of the parB system of plasmid R1 and its homology with the relF gene product of the E. coli relB operon. EMBO J 1986; 5:2023 - 9; PMID: 3019679
- Gerdes K, Wagner EG. RNA antitoxins. Curr Opin Microbiol 2007; 10:117 - 24; http://dx.doi.org/10.1016/j.mib.2007.03.003; PMID: 17376733
- Gerdes K, Thisted T, Martinussen J. Mechanism of post-segregational killing by the hok/sok system of plasmid R1: sok antisense RNA regulates formation of a hok mRNA species correlated with killing of plasmid-free cells. Mol Microbiol 1990; 4:1807 - 18; http://dx.doi.org/10.1111/j.1365-2958.1990.tb02029.x; PMID: 1707122
- Gerdes K, Nielsen A, Thorsted P, Wagner EG. Mechanism of killer gene activation. Antisense RNA-dependent RNase III cleavage ensures rapid turn-over of the stable hok, srnB and pndA effector messenger RNAs. J Mol Biol 1992; 226:637 - 49; http://dx.doi.org/10.1016/0022-2836(92)90621-P; PMID: 1380562
- Franch T, Gerdes K. Programmed cell death in bacteria: translational repression by mRNA end-pairing. Mol Microbiol 1996; 21:1049 - 60; http://dx.doi.org/10.1046/j.1365-2958.1996.771431.x; PMID: 8885274
- Franch T, Gultyaev AP, Gerdes K. Programmed cell death by hok/sok of plasmid R1: processing at the hok mRNA 3′-end triggers structural rearrangements that allow translation and antisense RNA binding. J Mol Biol 1997; 273:38 - 51; http://dx.doi.org/10.1006/jmbi.1997.1294; PMID: 9367744
- Gultyaev AP, Franch T, Gerdes K. Programmed cell death by hok/sok of plasmid R1: coupled nucleotide covariations reveal a phylogenetically conserved folding pathway in the hok family of mRNAs. J Mol Biol 1997; 273:26 - 37; http://dx.doi.org/10.1006/jmbi.1997.1295; PMID: 9367743
- Møller-Jensen J, Franch T, Gerdes K. Temporal translational control by a metastable RNA structure. J Biol Chem 2001; 276:35707 - 13; http://dx.doi.org/10.1074/jbc.M105347200; PMID: 11461923
- Zuker M. Mfold web server for nucleic acid folding and hybridization prediction. Nucleic Acids Res 2003; 31:3406 - 15; http://dx.doi.org/10.1093/nar/gkg595; PMID: 12824337
- Thisted T, Sørensen NS, Gerdes K. Mechanism of post-segregational killing: secondary structure analysis of the entire Hok mRNA from plasmid R1 suggests a fold-back structure that prevents translation and antisense RNA binding. J Mol Biol 1995; 247:859 - 73; http://dx.doi.org/10.1006/jmbi.1995.0186; PMID: 7536849
- Gerdes K, Poulsen LK, Thisted T, Nielsen AK, Martinussen J, Andreasen PH. The hok killer gene family in gram-negative bacteria. New Biol 1990; 2:946 - 56; PMID: 2101633
- Loh SM, Cram DS, Skurray RA. Nucleotide sequence and transcriptional analysis of a third function (Flm) involved in F-plasmid maintenance. Gene 1988; 66:259 - 68; http://dx.doi.org/10.1016/0378-1119(88)90362-9; PMID: 3049248
- Nielsen AK, Thorsted P, Thisted T, Wagner EG, Gerdes K. The rifampicin-inducible genes srnB from F and pnd from R483 are regulated by antisense RNAs and mediate plasmid maintenance by killing of plasmid-free segregants. Mol Microbiol 1991; 5:1961 - 73; http://dx.doi.org/10.1111/j.1365-2958.1991.tb00818.x; PMID: 1722558
- Pedersen K, Gerdes K. Multiple hok genes on the chromosome of Escherichia coli. Mol Microbiol 1999; 32:1090 - 102; http://dx.doi.org/10.1046/j.1365-2958.1999.01431.x; PMID: 10361310
- Faridani OR, Nikravesh A, Pandey DP, Gerdes K, Good L. Competitive inhibition of natural antisense Sok-RNA interactions activates Hok-mediated cell killing in Escherichia coli. Nucleic Acids Res 2006; 34:5915 - 22; http://dx.doi.org/10.1093/nar/gkl750; PMID: 17065468
- Griffiths-Jones S, Moxon S, Marshall M, Khanna A, Eddy SR, Bateman A. Rfam: annotating non-coding RNAs in complete genomes. Nucleic Acids Res 2005; 33:Database issue D121 - 4; http://dx.doi.org/10.1093/nar/gki081; PMID: 15608160
- Nawrocki EP, Kolbe DL, Eddy SR. Infernal 1.0: inference of RNA alignments. Bioinformatics 2009; 25:1335 - 7; http://dx.doi.org/10.1093/bioinformatics/btp157; PMID: 19307242
- Pruitt KD, Tatusova T, Klimke W, Maglott DR. NCBI Reference Sequences: current status, policy and new initiatives. Nucleic Acids Res 2009; 37:Database issue D32 - 6; http://dx.doi.org/10.1093/nar/gkn721; PMID: 18927115
- Seibel PN, Müller T, Dandekar T, Schultz J, Wolf M. 4SALE--a tool for synchronous RNA sequence and secondary structure alignment and editing. BMC Bioinformatics 2006; 7:498; http://dx.doi.org/10.1186/1471-2105-7-498; PMID: 17101042
- Merino E, Yanofsky C. Transcription attenuation: a highly conserved regulatory strategy used by bacteria. Trends Genet 2005; 21:260 - 4; http://dx.doi.org/10.1016/j.tig.2005.03.002; PMID: 15851059
- Mandal M, Breaker RR. Gene regulation by riboswitches. Nat Rev Mol Cell Biol 2004; 5:451 - 63; http://dx.doi.org/10.1038/nrm1403; PMID: 15173824
- Chadalavada DM, Cerrone-Szakal AL, Bevilacqua PC. Wild-type is the optimal sequence of the HDV ribozyme under cotranscriptional conditions. RNA 2007; 13:2189 - 201; http://dx.doi.org/10.1261/rna.778107; PMID: 17956974
- Wong TN, Sosnick TR, Pan T. Folding of noncoding RNAs during transcription facilitated by pausing-induced nonnative structures. Proc Natl Acad Sci U S A 2007; 104:17995 - 8000; http://dx.doi.org/10.1073/pnas.0705038104; PMID: 17986617
- Micura R, Höbartner C. On secondary structure rearrangements and equilibria of small RNAs. Chembiochem 2003; 4:984 - 90; http://dx.doi.org/10.1002/cbic.200300664; PMID: 14523915
- Finn RD, Mistry J, Tate J, Coggill P, Heger A, Pollington JE, et al. The Pfam protein families database. Nucleic Acids Res 2010; 38:Database issue D211 - 22; http://dx.doi.org/10.1093/nar/gkp985; PMID: 19920124
- Lai D, Proctor JR, Zhu JY, Meyer IM. R-CHIE: a web server and R package for visualizing RNA secondary structures. Nucleic Acids Res 2012; 40:e95; http://dx.doi.org/10.1093/nar/gks241; PMID: 22434875