Abstract
6S RNA is a highly abundant small non-coding RNA widely spread among diverse bacterial groups. By competing with DNA promoters for binding to RNA polymerase (RNAP), the RNA regulates transcription on a global scale. RNAP produces small product RNAs derived from 6S RNA as template, which rearranges the 6S RNA structure leading to dissociation of 6S RNA:RNAP complexes. Although 6S RNA has been experimentally analysed in detail for some species, such as Escherichia coli and Bacillus subtilis, and was computationally predicted in many diverse bacteria, a complete and up-to-date overview of the distribution among all bacteria is missing. In this study we searched with new methods for 6S RNA genes in all currently available bacterial genomes. We ended up with a set of 1,750 6S RNA genes, of which 1,367 are novel and bona fide, distributed among 1,610 bacteria, and had a few tentative candidates among the remaining 510 assembled bacterial genomes accessible. We were able to confirm two tentative candidates by Northern blot analysis. We extended 6S RNA genes of the Flavobacteriia significantly in length compared to the present Rfam entry. We describe multiple homologs of 6S RNAs (including split 6S RNA genes) and performed a detailed synteny analysis.
Introduction
6S RNA is a highly abundant small non-coding RNA (ncRNA), which was first identified in E. coli.Citation1 Although it was one of the first small RNAs to be sequenced,Citation2 its function remained unclear for almost 30 years. In 2000, E. coli 6S RNA was discovered to form a complex with the RNA polymerase (RNAP).Citation3 By binding to the σ70-containing housekeeping RNAP holoenzyme, it inhibits a large number of σ70-dependent genes and thus enables a better adaption to stationary phase and environmental stress.Citation4-Citation7 For B. subtilis, the second major model organism, it was found that its counterpart to E. coli 6S RNA, 6S-1 RNA, delays the onset of sporulation by decreasing the rate of nutrient consumption.Citation8 One striking feature of 6S RNA is its ability to serve as template for the synthesis of small product RNAs (pRNAs), which mediate the release of 6S RNA from the housekeeping holoenzyme (σ70-RNAP in E. coli).Citation5,Citation9 This dissociation is the result of a structural rearrangement of the 6S RNA during transcription of the pRNAs.Citation10,Citation11
6S RNAs are widespread among bacteria: They have been identified in many diverse bacterial groups, either by sequencing, or more often using computational approaches that search directly for 6S RNA or for sRNAs in general.Citation12–Citation17 Due to low sequence conservation, computational searches are mainly based on secondary structure comparison. The secondary structure of 6S RNA consists of a large double-stranded hairpin with a central bulge. This bulge is essential for the function of 6S RNA as it resembles an open promoter complex.Citation12,Citation13,Citation18
In the Rfam database the 6S RNA is described by two entries, 6S (RF00013) with 153 sequences in the seed alignment and 6S-Flavo RNA (RF01685) with 89 sequences in the seed alignment. The general entry of the 6S RNA covers mainly large bacterial groups, such as γ-Proteobacteria and Firmicutes. The model of 6S-Flavo RNA contains, as the name already indicates, sequences of Flavobacteriia and mainly marine metagenome sequences (77 sequences). Some low-GC Gram positive bacteria, including B. subtilis and Thermoanaerobacter tengcongensis, encode two divergent 6S RNA homologs,Citation12,Citation13 which are part of the Rfam entry.
Here, we present a complete overview of the distribution of 6S RNA genes within the entire domain of bacteria. We screened available bacterial genomes for the existence of 6S RNA genes using new approaches (hairpin formation, structural rearrangement upon pRNA binding, and manual dot plot analysis) and present a comprehensive set of 1,750 detected 6S RNA candidates in 1,610 bacterial genomes. We give an overview of species that have more than one 6S RNA gene and present results of an extensive synteny study. Selected tentative candidates were confirmed by Northern blot analyses.
Results and Discussion
Detected 6S RNA genes
We detected, manually inspected and therefore verified in silico 1,750 6S RNA genes in 1,611 bacterial genomes. We had a few tentative 6S RNA candidates among the 510 remaining bacteria (see ). The average length of 6S RNAs of the different phyla is 183 nt, varying from 153 nt in Erysipelothrix rhusiopathiae str. Fujisawa (Firmicutes) to 237 nt in Pelodictyon phaeoclathratiforme BU-1 (Chlorobi). The Rfam entry RF01685 for Flavobacteriia contains sequences with a length of 103 nt, which are erroneous annotations, see below (Bacteroidetes). Altogether, we were able to explore large phyla such as the Firmicutes and Proteobacteria nearly completely, and could identify 6S RNA candidates in some smaller, so far neglected phyla such as the Chloroflexi or Spirochaetes.
Table 1. Overview of investigated bacteria and identified 6S RNAs. Phyla are ordered according to the phylogenetic tree of Wu et al. [Citation19], disregarding those with less than five species, which are listed at the bottom of the table. NCBI – Number of bacteria, which had a 6S RNA gene entry in their NCBI annotation; Explored – Number of available bacterial genomes; Identified in this study – Number of species with a 6S RNA gene are listed according to the number of detected genes; ? – Candidates; Ther. – Thermus; s – For the group of Flavobacteriia, only partial 6S RNA sequences were previously reported; u – Number of species not investigated in this study (no assembled genome available, including genes derived from metagenomic studies); bold font – highly significant results of this study; – Others: *Five Rfam entries: Caenorhabditis remanei (Opisthokonta), Chlorella vulgaris (Viridiplantae), Oryza sativa Japonica (Viridiplantae), and metagenomic data; ** – Candidate of Acidilobus saccharovorans (Crenarchaeota)
Consensus secondary structures can be found in the Supplemental Material.
Firmicutes
The phylum Firmicutes has been described quite well before, as it can be seen from the high number of species present in the Rfam seed alignment or those with an annotated gene in their NCBI annotations. Furthermore, several experimental studies such as for B. subtilisCitation10,Citation13,Citation20–Citation23 exist. We identified in 468 of 472 genomes at least one 6S RNA gene and thus only missed Halobacteroides halobius DSM 5150 and three Lactobacillus species. Some species of the Firmicutes are known to exhibit multiple homologs of 6S RNA, which we describe in detail below.
Chloroflexi and Cyanobacteria
For only a few members (6/16) of the Chloroflexi a 6S RNA could be identified. This group attracted our attention as detected 6S RNAs were not uniformly distributed. While we found 6S RNA genes in the genus Roseiflexus, searches in the genus Chloroflexus, which belongs also to the order Chloroflexales, returned no results. Therefore, we selected Roseiflexus castenholzii for experimental validation (see below).
We were easily able to detect a copy of 6S RNA for all Cyanobacteria, due to the extensive previous exploration that resulted in 6S RNA annotations in 40/71 Cyanobacteria.Citation14,Citation24–Citation26
Actinobacteria
The Actinobacteria are comprised of mainly Gram-positive species with a high GC-content (> 55%),Citation27–Citation29 which are highly diverse in terms of their morphology, physiology, and metabolic capabilities.Citation27,Citation29,Citation30 We found possible 6S RNA genes in seven of 235 species, all being Coriobacteridae: Atopobium parvulum DSM 20469, Coriobacterium glomerans PW2, Cryptobacterium curtum DSM 15641, Eggerthella sp. YY7918, Eggerthella lenta DSM 2243, Olsenella uli DSM 7084, and Slackia heliotrinireducens DSM 20476.
Interestingly, Gao et al.Citation29 proposed the exclusion of the Coriobacteriia from the phylum Actinobacteria based on analysis of conserved signature proteins (CSPs) and conserved signature indels (CSI). Additionally, Coriobacteriia are separated from all other members of the Actinobacteria by a long branch in a phylogenetic tree based upon concatenated protein sequences.Citation29
The presence of 6S RNAs in Actinobacteria has been previously addressed. Panék et al.Citation31 used suboptimal structures to find 6S RNAs and reported candidates in Streptomyces and Mycobacterium. The candidate 6S RNA of M. smegmatis did not bind to the σ70-RNAP homolog of this bacterium, as inferred from co-immunoprecipitation experiments.Citation31 It has to be seen whether this RNA is either not a genuine 6S RNACitation32 or may specifically bind to core RNAP or RNAP associated with other sigma factors. For S. coelicolor a deep sequencing analysis was performed,Citation33 reporting that the predicted 6S RNA candidate of Panék et al.Citation31 corresponds to a highly expressed putative ncRNA. However, genomic positions of the observed transcripts and the predicted 6S RNA overlap only in a few nucleotides. Our searches did not reveal any candidates in Streptomyces or in Mycobacterium. We analysed the suggested candidates with our approaches focusing on possible rearrangements. We found rearrangements to be possible, but not favourable. As the candidates did not fulfill our criteria for bona fide or tentative 6S RNAs, especially on the basis of our experience with previous data, we did not include them in further analyses of this study.
Spirochaetes
This phylum is more diverse than other phyla as it comprises families with (Brachyspiraceae) and without (Leptospiraceae) a 6S RNA. Within the Spirochaetaceae, the genus Borrelia generally harbours a 6S RNA gene, while we found it only in some Spirochaeta and completely failed to detect it within the Treponema. In total, one 6S RNA per genome could be identified in 27 of the 53 Spirochaetes. Interestingly, Borrelia 6S RNAs differ in their secondary structure from other Spirochaetes with an idiosyncratic 6S RNA dot plot, as they show a different rearrangement and hairpin indicating an inverse refolding (see ).
Figure 1. 6S RNA dot plots and corresponding secondary structures without and with pRNA annealing. (A) Top: ”Standard” dot plot of free 6S RNA, illustrated for 6S RNA of Clostridium acidurici 9a. According to this type of dot plot, the characteristic rod-shaped structure (bottom, left structure) is among the energetically suboptimal conformers, whereas the central hairpin formed after pRNA annealing (bottom, right structure) is already evident (prevalent in this example) in the free structure. (B) ”Borrelia” dot plot (Borrelia burgdorferi B31) – here, the central bulge region is predicted to be more compacted in the ground state (bottom, left structure) than in complex with pRNA (bottom, right structure). (C) “Spirochaete” dot plot (Brachyspira pilosicoli 95) – highly variable secondary structures being a specific feature of Spirochaetes. Used constraints for secondary structure prediction are provided in the Supplemental Material.
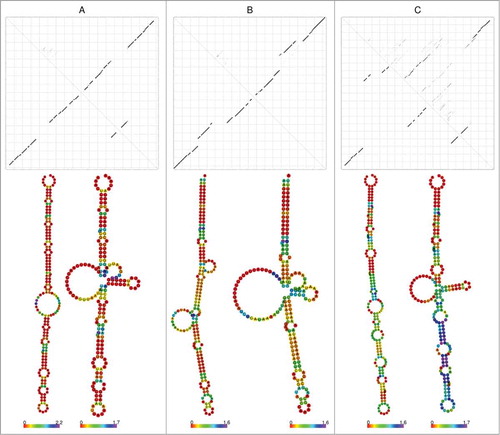
Planctomycetes, Chlamydiae and Verrucomicrobia
These phyla are closely related to each other according to 16S rRNA analysis.Citation34 For five of seven Planctomycetes a 6S RNA gene could be identified, whereas the absence of 6S RNA genes is suggested for Pirellula staleyi and Rhodopirellula baltica. We found vague candidates for a putative 6S RNA in Protochlamydia amoebophila UWE25, Parachlamydia acanthamoebae UV7, Simkania negevensis Z, and Waddlia chondrophila WSU 86-1044, of which the latter candidate is plasmid-encoded. Furthermore, we detected in all four analysed members of the Verrucomicrobia (namely Opitutus terrae PB90-1, Coraliomargarita akajimensis DSM 45221, Akkermansia muciniphila ATCC BAA-835, and Methylacidiphilum infernorum V4) a possible 6S RNA gene.
Bacteroidetes, Chlorobi and Ignavibacteria
About 100 species are known within the Bacteroidetes, including the class Flavobacteriia, for which a separate Rfam class has been created (RF01685). This class contains sequences with lengths from 103 nt to 132 nt. However, these sequences did not show the typical features of 6S RNAs. Therefore, we extended them and found Flavobacteriia 6S RNAs to have a length of 160 nt to 178 nt. The here proposed sequences are able to form a long hairpin with a central bulge. In addition, the refolding after pRNA annealing is comparable to that known from other 6S RNAs. Here we are able to report, for the first time, 6S RNAs for the green sulfur bacteria Chlorobi, the Ignavibacteria and for most of the other Bacteroidetes, missing mainly species of the Sphingobacteriia and Cytophagia.
Aquificae
We were able to identify a 6S RNA gene in all members of the Aquificae.Citation35 In Desulfurobacterium thermolithotrophum DSM 11699 and Thermovibrio ammonificans HB-1, both members of the family Desulfurobacteriaceae, the detected 6S RNA candidates are tentative, as they differed too much from the 6S RNAs predicted in the other members of the phylum Aquificae. For A. aeolicus the 6S RNA was already identified via an experimental RNomics approach.Citation36
Proteobacteria
In 905 of the 961 available proteobacterial genomes, we detected at least one 6S RNA gene.
The conserved consensus secondary structures of α-, β-, γ-, δ/ε-Proteobacteria can be found in the Supplemental Material. In δ/ε-Proteobacteria, which include the recently published sequences of Helicobacter pyloriCitation15 and Campylobacter jejuni,Citation37 we predict for each genome a reliable 6S RNA candidate. Most genomes without reliable predictions of 6S RNA genes within the Proteobacteria belong to the species Candidatus. Besides these non-cultivable species, no 6S RNA was found in Alpha proteobacterium HIMB59, Thalassobaculum L2 and in the genera Orientia tsutsugamushi, all belonging to the α-Proteobacteria. Furthermore, negative results were obtained for the genus Taylorella of the β-Proteobacteria and some genera of the γ-Proteobacteria, including Buchnera, Serratia and Wigglesworthia.
Other Phyla
6S RNA genes were detected in all members of the Fusobacteria, Acidobacteria, Caldiserica, Chrysiogenetes, Deferribacteres, Dictyoglomi, Gemmatimonadetes, Nitrospirae, Synergistetes, and Thermodesulfobacteria.
For bacteria of the phyla Deinococcus, Thermus, Thermotogae, Tenericutes, Elusimicrobia, and Fibrobacteres, no 6S RNA gene could be discovered, with the exception of some very vague candidates, which are reported in the Supplemental Material.
Bacteria with multiple 6S RNA genes
To our knowledge, we present here for the first time a complete overview of genomes exhibiting at least two 6S RNA genes. The function of such additional 6S RNAs is under debate. In the model system B. subtilis, the second homolog (6S-2 RNA) was shown to be able to basically exert the same functions as the canonical variant (6S-1 RNA) in vitro, i.e. to serve as template for pRNA transcription, to inhibit transcription from DNA promoters, and to form stable 6S RNA:pRNA hybrid structures that trigger 6S RNA release from RNAP.Citation23 However, pRNA initiation on 6S-2 RNA is less efficient than on 6S-1 RNA in vitro,Citation22 and so far only 6S-1 but not 6S-2 RNA-derived pRNAs have been detected in total RNA preparations from B. subtilis.Citation20,Citation21
Two divergent 6S RNAs have previously been described for some low-GC-Gram-positive bacteria, such as Firmicutes with B. subtilis, Bacillus halodurans, Clostridium acetobutylicum, Oceanobacillus iheyensis, and Thermoanaerobacter tengcongensisCitation12 and for the γ-Proteobacterium Legionella pneumophila.Citation17
According to the phylogenetic tree containing all multiple 6S RNA genes, paralogous 6S RNA genes of Firmicutes developed separately, see Supplemental Material.
Firmicutes
We detected in the phylum Firmicutes 95 species with two copies. In all 79 Bacillaceae (except for Amphibacillus xylanus NBRC 15112, Anoxybacillus flavithermus WK1, Bacillus amyloliquefaciens FZB42, and Halobacillus halophilus DSM 2266) a second 6S RNA gene was found. Interestingly, in Bacillus coagulans 2-6 one 6S RNA gene is split up into two parts with different locations on the chromosome. When these two partial sequences are concatenated to one sequence and aligned with the second copy of the closely related B. coagulans 36D1, it becomes obvious that these sequences are almost identical. The respective alignment is shown in the Supplemental Material. An example for a partial 6S RNA gene is B. amyloliquefaciens FZB42, where only a single full-length 6S RNA gene could be identified. Additional searches for the second copy resulted in a short hit of 65 nt covering perfectly the last third of the 6S RNA. (see Supplemental Material). Whether these examples reflect genuine 6S RNA homologs or assembly artefacts remains to be clarified. Interestingly, the two 6S genes in B. anthracis, B. cereus, B. cytotoxicus, B. thuringiensis, and B. weihenstephanensis are arranged as tandem copies within their genomes.
Furthermore, two 6S RNA genes are identifiable in other Firmicutes (see Supplemental Material), including Brevibacillus brevis NBRC 100599, and Solibacillus silvestris StLB046. For Bacillus cellulosilyticus DSM 2522, Bacillus megaterium QM B1551, and B. subtilis BEST7613 even three 6S RNA genes could be identified. However, in the case of B. subtilis BEST7613, the third gene was very likely acquired by horizontal gene transfer (see below).
The genus Clostridium is most diverse: The number of 6S RNA genes varies between one and four (C. ljungdahlii). C. acetobutylicum and related genomes encode three, of which two are identical.
Almost all 6S RNAs of Firmicutes seem to date back to two common ancestors, which could be the result of a gene duplication early in Firmicutes evolution, see . Within the Bacilli we see a clear separation of the paralogous genes per species. The only exception is a more recent duplication event including the tandem copies of some Bacilli (-right part).
Figure 2. Phylogenetic network of the multiple 6S RNA homologs within the Firmicutes . The grey triangle covers homologs occurring as tandem 6S RNA gene copies that are closely related to each other, indicating that they originate from recent gene duplications. The phylogenetic tree can be divided into two parts (separated by the red lines), each representing mainly one of the two homologs per Firmicutes genome. Note that Clostridia are exceptional, as they have more than two 6S RNA genes. In order to keep the color code legible, several bacteria respresented by single species were indicated by numbers. A phylogenetic network with all named species can be found in the Supplemental Material.
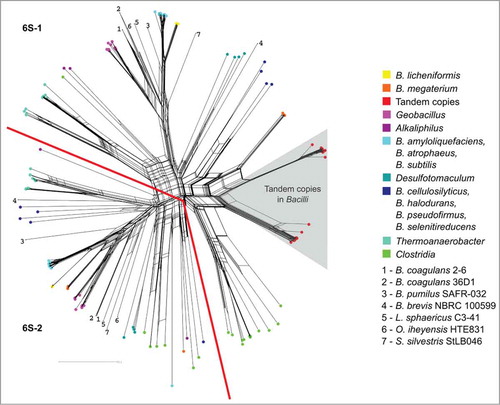
Actinobacteria and Cyanobacteria
We discovered two vague candidates in Bifidobacterium animalis lactis, whereas all closely related Bifidobacteria contained no homologous gene. However, two candidates showed promising secondary structures.
Two distinct types of 6S RNA were also described in Prochlorococcus MED4.Citation24 However, these 6S RNAs differ only in length and have the same genomic location. According to our definition, only two distinct genomic locations were considered to be different 6S RNAs. Therefore, we report one gene locus per cyanobacterial genome, with the exception of Synechococcus RCC307, for which we found a second 6S RNA gene, which is with 132 nt strikingly shorter than the typical 6S RNA with a length of about 180 nt. Although secondary structure and pRNA-induced refolding predictions support 6S RNA identity, the lack of conservation among closely related organisms makes this candidate questionable.
α- and β-Proteobacteria
All α- and β-Proteobacteria are consistently predicted to encode a single 6S RNA. The only exception is Magnetococcus sp. MC-1 containing four 6S RNA candidates, three of them being highly similar. The fourth gene is most closely related to that of the γ-proteobacterium Acidithiobacillus, being a possible inclusion via horizontal gene transfer in combination with a known syntenic protein of 6S RNA, the 5-formyltetrahydrofolate cyclo-ligase (see Synteny section below). Barrick et al.Citation12 identified multiple 6S RNA genes in Magnetococcus sp. MC-1 and Magentospirillum magnetotacticum sp. MS-1. However, at this time the genomes were not fully assembled.
γ-Proteobacteria
In all Legionella species two 6S RNA genes were identified, which cross-validates the findings of Weissenmayer et al.Citation17 who also detected a second 6S RNA in their deep sequencing study of L. pneumophila. Furthermore, we bring up here a possible third 6S RNA candidate discovered in Legionella discovered by infernal. However, this copy had a lower bit-score and also its secondary structure differed strongly from typical 6S RNA structures.
For all Marinobacteres we detected two copies of 6S RNA being annotated roughly at the same genomic location, but antisense to each other. This seems to be a specific feature confined to the Marinobacteres.
δ-Proteobacteria
We found at least two 6S RNA genes in nearly all Desulfuromonadales, which include the Geobacteraceae and the Pelobacteraceae, except for Geobacter lovely SZ and Pelobacter propionicus DSM 2379. For Geobacter uraniireducens Rf4 and Pelobacter carbinolicus DSM 2380 even more than two, namely four and three, 6S RNAs could be detected.
All non-Firmicutes multiple 6S RNA genes were analysed (see Supplemental Material), suggesting that most homologs are more recent duplications, with the exception of Geobacter species that harbour more divergent 6S RNA copies, similar to the Firmicutes.
Vague Candidate Validation
Besides our in silico studies, we validated two 6S RNA candidates (Roseiflexus castenholzii DSM 13941 and Sulfurihydrogenibium sp. YO3AOP1) additionally by Northern blot experiments. R. castenholzii is a thermophilic, photosynthenic bacterium, which belongs to the Chloroflexi, the earliest branching phylum of bacteria that includes phototrophs.Citation38,Citation39
S. sp. YO3AOP1 is also a thermophilic bacterium and is a representative of the Aquificales, whose exact phylogenetic position is still under debate.Citation40 According to 16S rRNA analysis they represent the most rooted bacterial group, whereas phylogenetic reconstructions based on proteins retrieve other positions.Citation41,Citation42 We selected these species as they belong to smaller phyla, for which 6S RNAs are often neither annotated nor described, in contrast to the large phyla such as the Proteobacteria or Firmicutes. While within the Aquificales some studies were already performed for A. aeolicus,Citation36 nothing is known about 6S RNA in the Chloroflexi. For 6S RNA candidates of R. castenholzii and S. sp., RNAfold analyses revealed long hairpin structures among the near-isoenergetic structure predictions (, see minor dots along the diagonales in the upper triangles of the dot plots). Introduction of only minor folding constraints (see Supplemental Material) predicted the rod-shaped structure as the prevalent one. The rod-shaped structures were also among the energetically most favourable ones.
Figure 3. Detection of 6S RNAs by Northern blot analysis. Ground state secondary structures (predicted with minor constraints, see Supplemental Material), unconstrained dot plots and Northern blots of Roseiflexus castenholzii (top) and Sulfurihydrogenibium sp. (bottom) 6S RNAs are shown. The experimentally detected 6S RNAs are expressed at a length as bioinformatically predicted (R. castenholzii: 173 nt and S. azorense: 189 nt, respectively). In the left halves of Northern blots, probes for sense 6S RNAs were used, while probes for antisense 6S RNAs were used in the right halves. 3, 5 and 10 μg of total cellular RNA were loaded on 10% denaturing PAA gels. T7 transcripts were used as sense (+) and antisense (-) controls, where sense transcripts correspond to the predicted 6S RNA, whereas antisense RNAs are complementary to the respective 6S RNA candidate. The RNA size marker (in nt) was a mixture of different T7 transcripts. Additionally, the 6S RNA in vitro transcript for each bacterium was added to the marker mix as a further length control (indicated by asterisks).
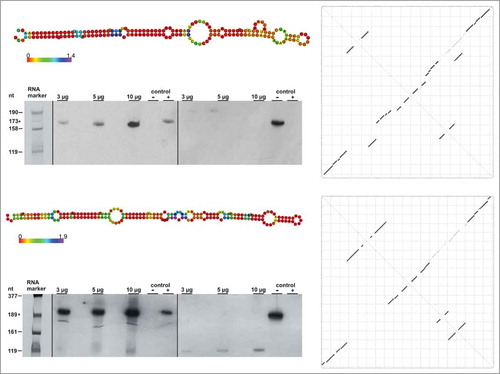
Northern blot analysis showed that both 6S RNAs were expressed as predicted by our in silico studies (see ). For both 6S RNAs we could not detect significant amounts of 6S RNA antisense transcripts. RNAs were extracted from the late-exponential to stationary growth phase, where most 6S RNAs have their highest expression levels.
pRNAs
One striking feature of 6S RNAs is their function as templates for the synthesis of short pRNA transcripts, a process that triggers 6S RNA release from RNAP, particularly under conditions when stationary cells enter a new exponential growth phase.Citation5,Citation9,Citation43 pRNA synthesis has been investigated in detail in E. coli and B. subtilis,Citation44 but has also been observed to occur in H. pyloriCitation15 and in SynechoystisCitation45 as well as in other Cyanobacteria.Citation26 The lengths of the described pRNAs were mainly in the range of 10-20 nt,Citation46 whereas in Cyanobacteria slightly longer pRNA transcripts (∼ 30 nt) were observed.Citation45 E. coli,Citation43 SynechocystisCitation26,Citation45 and HelicobacterCitation15 6S RNAs as well as B. subtilis 6S-2 RNACitation47 give rise to synthesis of pRNAs with a 5’-terminal A residue, whereas pRNAs synthesized on B. subtilis 6S-1 RNA initiate with a G residue Citation47.
Nowadays, more and more transcriptome studies (RNA-Seq) are accessible and the question arises whether 6S RNA identification is straightforward based on transcriptome profiles. In we display 6S RNA regions, transcripts and secondary structures (6S RNA in the ground state of Helicobacter pylori (ε-Proteobacteria), Listeria monocytogenes (Firmicutes), B. subtilis (Firmicutes), E. coli (γ-Proteobacteria), and Xanthomonas campestris (γ-Proteobacteria).
Figure 4. 6S RNA and pRNA transcripts. (A) General sketch of rearrangements upon pRNA synthesis. While these rearrangements are well described for pRNA synthesis in the 5’-bulge region, resulting in the formation of a central bulge collapse helix (magenta) and/or a local hairpin (orange)Citation10), further investigations are needed with respect to possible rearrangements induced by pRNA synthesis in the 3’-bulge region. (B) - (F) RNA-Seq screenshots of 6S RNA loci illustrated using the Integrated Genome Browser; (B) H. pylori (3,000/50); on the y-axis 3,000 reads are shown for 6S RNA, indicated by black vertical lines; pRNA reads (antisense to 6S RNA) are indicated by red vertical lines, the scale is set to 50; (C) L. monocytogenes (100/100), Ion Torrent sequencing; (D) B. subtilis bsrA (6S-1 RNA) locus (50,000/40); (E) E. coli (10,000/100); (F) X. campestris (100/50). Green boxes – secondary structure of free 6S RNA; unpaired regions represented by gaps between the green boxes.
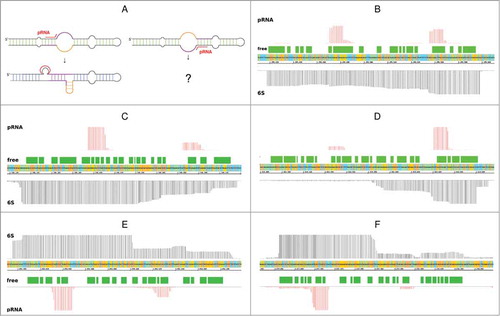
Interestingly, in each of the shown RNA-Seq examples, we were able to see not only one, but two regions of short transcripts antisense to 6S RNA. The region antisense to the 5’- portion of the central bulge is in the majority of cases transcribed more intensely, with the exception of H. pylori () and L. monocytogenes, the only Ion Torrent sample, (). Generally, both product RNAs are transcribed from opposite sites of the central bulge towards the 5’ end of the 6S RNA, suggesting that both strands serve as template for pRNA synthesis in vivo.
Secondary structure analysis showed that in most of the cases both parts of the central bulge are able to form hairpin structures (see Supplemental Material), consistent with the notion that RNAP can in principle rearrange and release 6S RNAs when the latter are bound in in reverse orientations.
Synteny
We analysed the syntenic regions for all predicted 6S RNA sequences, also including tentative candidates. For this purpose, we took the six up- and downstream located protein coding genes of each potential 6S RNA location into account and performed an all-against-all homology search scanning for similarities in the corresponding regions.
As expected, we found no syntenic pattern valid for all bacteria. The most widespread syntenic conservation was observed among the γ-Proteobacteria within the Enterobacteriaceae. Here we were able to find the same neighbour proteins in Citrobacter, Cronobacter, Enterobacter, Erwinia, Escherichia, Klebsiella, Pantoea, Shigella and Salmonella.
However, we could identify some proteins that frequently occur in the syntenic regions of the 6S RNA genes (see and Supplemental Material, where a full list of the proteins and their location in relation to the 6S RNA genes is provided).
Table 2. Syntenic proteins of the 6S RNA loci. For synteny analyses six protein-coding genes located up- and downstream of putative 6S RNA genes were investigated. Frequently occurring ones are listed here with their corresponding Pfam accession number. Bold font – at least half of the known 6S RNA genes of this group are linked to the indicated protein gene; #6S – Number of 6S RNAs identified in this study; 5-FTHF_cyc-lig – 5-formyltetrahydrofolate cyclo-ligase; ZapA – Cell division protein ZapA; AAA(+) – P-loop ATPase; Peptidase_M24 – Metallopeptidase family M24.
ygfA
In line with previous studies we found ygfA genes adjacent to 6S RNA sequences. Barrick et al.Citation12 described this conserved juxtaposition of 6S RNA and ygfA in Proteobacteria, mainly for α-, γ-, and only for a few β-Proteobacteria. We could extend this observation also to some δ-Proteobacteria (7 species) and Firmicutes (6 species). In smaller phyla, such as the Aquificae (described for A. aeolicus inCitation36) and Deferribacteres, a ygfA homolog was detected next to all predicted 6S RNA genes. ygfA codes for the 5-formyltetrahydrofolate cyclo-ligase, which catalyses the conversion of 5-formyltetrahydrofolate (5-FTHF) to 5-10-methenyltetrahydrofolate requiring ATP and Mg2+.Citation48 As 5-FTHF inhibits other folate-dependent enzymes, it is possible that the 5-formyltetrahydrofolate cyclo-ligase regulates indirectly several key metabolic processes, such as purine, pyrimidine and amino acid biosynthesis.Citation49,Citation50
Interestingly, 6S RNA was found in some species to overlap with ygfA in sense direction. This occurrence was observed in 35 species (8 Firmicutes, 13 α-Proteobacteria and 14 γ-Proteobacteria), suggesting that expression of 6S RNA and ygfA are counter-regulated by alternative processing or termination mechanisms.Citation44
zapA
Another gene, which was found even more frequently within the syntenic regions of 6S RNA genes, namely in 727 species, is zapA encoding the cell division protein ZapA. ZapA interacts with the cell division gene ftsZ, whose protein product assembles into a ring, the FtsZ ring, serving as a scaffold for the recruitment of division proteins during early cytokinesis. ZapA tunes the Z-ring spatio-temporally by driving the polymerisation and filament bundling of FtsZ.Citation51,Citation52 In the vicinity of 6S RNA genes, we identified genes coding for proteins of the ZapA family not only among all phyla of the Proteobacteria, including 661 species, but also in bacteria belonging to the phyla Bacteroidetes (33), Chlorobi (11), and Acidobacteria (5, whereas the remaining 3 genomes have ’Candidatus’ status and lack the linkage to zapA).
AAA(+) proteins
The superfamily of P-loop ATPases associated with diverse cellular activities (AAA(+) proteins) are involved in energy-dependent unfolding, disassociation and remodeling of macromolecules.Citation53 We detected members of this protein family mainly within syntenic regions of exactly one of the 6S RNA gene homologs in Firmicutes (in 316 of 360 species). Interestingly, the same applies to all 6S RNA homologs described here for the Deferribacteres, Synergistetes and nearly all Actinobacteria. For the Firmicutes, the linkage of 6S-1 RNA to AAA(+) protein genes now facilitates the annotation of 6S-1 vs. 6S-2 RNA genes.
Peptidase M24
When screening for proteins occurring frequently in syntenic regions, one often comes upon proteins that are characteristic for large phyla, such as subdivisions of the Proteobacteria. One example is the Xaa-Pro aminopeptidase, belonging to the Peptidase M24 (PF00557) family of metallopeptidases, which was found 331 times in 6S RNA syntenic regions, although largely confined to the γ-Proteobacteria (319 out of 331). This enzyme catalyzes the release of any N-terminal amino acid linked to a proline.Citation54
purK – Cyanobacteria
For Cyanobacteria it was reported that many 6S RNA genes are located directly adjacent to the purK gene, which encodes an enzyme involved in purine metabolism.Citation26 As PurK is a member of the superfamily of ATP-grasp proteins,Citation55 we used the ATP-grasp domain (PF02222) for detection of potential purK homologs and found the domain in the syntenic regions of 6S RNA genes in 40 Cyanobacteria, as well in six α-Proteobacteria.
purD – -Proteobacteria
In H. pylori a co-localization of the 6S RNA gene with another gene involved in purine metabolism, namely purD, encoding a glycinamide ribonucleotide synthetase, has been described.Citation15 We were able to detect the ATP-grasp domain of this protein (PF01071) in 81 of 82 -Proteobacteria and two Cyanobacteria.
The conserved linkage of these two analysed genes (purK and purD), and in general of 6S RNA genes and genes involved in purine metabolism, also including ygfA, suggests an intimate functional link.Citation26 This was indeed observed for a 6S RNA-deficient E. coli mutant strain showing deregulated expression of transporters and several enzymes involved in purine metabolism.Citation56
During our synteny analyses, we detected one interesting synteny relation, which went across phyla borders. We found a similar order of up- and downstream genes for B. subtilis BEST7613 (Firmicutes) and species of the genus Synechocystis (Cyanobacteria). This is especially interesting as B. subtilis BEST7613 is the only B. subtilis species showing three instead of two 6S RNA genes. Two of the genes have syntenies comparable with other Bacilli, whereas the third gene resembles, in terms of synteny, bacteria belonging to the genus Synechocystis. These observations are not so surprising anymore, when a closer look is taken at the origin of B. subtilis BEST7613. This bacterial genome is a result of a stable megacloning approach, in which the Synechocystis PCC6803 genome was cloned in the genome of B. subtilis 168 resulting in a composite genome.Citation57
The knowledge of these syntenic proteins can also be used during searches for 6S RNA homologs and their evaluation, as the presence of such a syntenic protein indicates a 6S RNA gene. We were, for example, able to detect an AAA(+) protein in the syntenic regions of the tentative 6S RNA in B. animalis lactis. However, as no further 6S RNA candidates could be found in the other Bifidobacteria, the status of this sequence remained ’tentative’.
Conclusion
Here, we have given a comprehensive overview of the occurrence of 6S RNA genes within the entire domain of Bacteria, including detailed information about potential multiple 6S RNA genes and syntenic proteins. We observed that many genomes lacking this gene are endosymbionts or parasitic. We were, for example, neither able to find a 6S RNA gene in Buchnera species, such as Buchnera aphidicoloa, a primary endosymbiont of aphids (A. pisum), nor in the extremely reduced genomes of the parasitic genus Mycoplasma. However, not all bacteria apparently lacking a 6S RNA gene have a symbiotic or parasitic lifestyle.
This raises the obvious question: Do we still miss some 6S RNA genes by our current approaches? Or do they simply not exist in some species? It has been shown for E. coli that bacteria survive without a 6S RNA gene under laboratory conditions. However, 6S RNA-deficient cells have disadvantages in circumstances when resources are limited.Citation6
The sequence similarity of the analysed genes is obviously low. However, the characteristic secondary structure, the features of the pRNA-induced rearrangements and the transcription profiles of 6S RNA loci are hallmarks of this ncRNA that facilitate the identification of new members of this class of regulatory RNAs. The finding of paralogous 6S RNAs, e.g. in B. subtilis, suggests that 6S RNA functions have diversified in such bacteria.
Materials and Methods
Homology searches of 6S RNA genes
With 153 known sequences of 6S RNA from RfamCitation58 (RF00013) we performed an iterative homology search against all available bacterial genomes (2121) from NCBI (Download date 22.10.2013) consisting of (a) blast -e 1e-4 (v.2.2.26),Citation59 (b) GotohScan -o 1 -e 1e-3 (v.2.0),Citation60 (c) infernal (v.1.1)Citation61 including secondary structure properties using covariance models (CM), and (d) RNABOBCitation62 for conserved pattern motif search.
Alignments of query and candidates were created using cmalign and locarna.Citation63 The alignments were manually inspected and curated using the Emacs Ralee modeCitation64 after each iteration.
This method has been applied to all query sequences at once, and for phylogenetically closely related subgroups, with a number of iterations until query and ouput file were identical.
All retrieved candidates were evaluated taking into account various criteria: (1) infernal score, (2) alignment of candidate sequence with 6S RNA sequences of closely related species, (3) predicted sencondary structure of the free RNA (a rod-shaped conformer identifiable within the ensemble of nearly isoenergetic structures), (4) predicted secondary structure after pRNA annealing (formation of a central bulge collapse helix and/or a stable hairpin in the 3-central bulge; in many cases, the rearranged structure was already seen as a suboptimal structure in dot plots of the free 6S RNA; seeCitation10), (5) identification of homologs in closely related species, (6) linkage of 6S RNA genes with certain protein genes (synteny). If the putative 6S RNA sequence fulfilled four of these criteria, it was declared as bona fide. If not, we classified it as a tentative 6S RNA. For example, we had some promising candidates showing 6S RNA characteristics such as the potential to adopt a rod-shaped structure and some potential to rearrange its structure upon annealing of putative pRNAs. However, these candidates had a low score and no homologs in closely related species were identified.
Analysis of multiple 6S RNA genes
For the analysis of multiple 6S RNA genes, alignments were calculated with ClustalW (v.2.0),Citation65 and were analysed with the NeighbourNet algorithmCitation66 of SplitTree4 (v.4.12.3).Citation67 This workflow was performed for detected 6S RNA homologs of (a) all bacteria with at least two 6S RNA genes, (b) Firmicutes only, and (c) species not belonging to the Firmicutes (Actinobacteria, α-, γ- and δ-Proteobacteria).
Secondary structure analysis
For secondary structure prediction and analysis we used various programs of the Vienna RNA PackageCitation68 and the Freiburg Tools, including RNAfold, RNAsubopt, RNAcofold, RNAduplex, and locarna. Dot plots of each candidate were assessed by eye. For pRNA-induced rearrangement analyses, the known validated rearranged structures and pRNA initiation sites were used to define corresponding folding constraints.Citation10
Synteny
For syntenic investigations we took the six closest annotated protein genes up- and downstream of each predicted 6S RNA gene according to the NCBI annotation as input for blast searches. For tblastn an E-Value of <10−10 and for blastn an E-Value of <10−5 was applied. We searched in the genomic regions corresponding to the selected syntenic genes of related 6S RNA genes. For the genes presented here in detail, we performed additionally a homology search using their corresponding seed alignments provided by the Pfam (v.27.0)Citation69 to detect synonyms.
Detection of 6S RNAs by Northern blot analysis
Total cellular RNA from bacterial cells in late exponential or stationary phase was extracted using the hot-phenol method.Citation70 6S RNAs were separated by 10% denaturing PAGE (8 M urea) with 1x TBE as electrophoresis buffer. For gel loading, 1 volume of denaturing gel loading buffer (0.02% (w/v) bromophenol blue, 0.02% (w/v) xylene cyanol blue, 8 M urea, 50% (v/v) formamide, 2x TBE) was mixed with 1 volume of RNA sample (for loaded amounts, see ) and heated to 98°C for 3 min, followed by immediate cooling on ice. For Northern blotting, RNA was blotted onto a positively charged nylon membrane (Roche Diagnostics, Cat. No. 11209299001) for 1 h by using a semi-dry blotter with 1.15 mA/cm2 in 0.5x TBE transfer buffer. After transfer, the RNA was fixed by UV crosslinking (120 mJ/cm2). For pre-hybridisation as well as hybridisation, 10 ml of hybridisation solution (Roche Diagnostics, Cat. No. 11796895001) were heated to 68°C and added to the membrane. The membrane was incubated by slow rotation for 2 h (pre-hybridisation) or overnight (hybridisation) at 68°C. Digoxigenin-labeled probes were denatured for 3 min at 98°C and cooled down on ice before addition to pre-heated hybridisation solution. Immunological detection of RNAs on the membrane was performed by using the Northern Starter Kit (Roche Diagnostics, Cat. No. 11585762001 ) followed by exposing an X-ray film for 40 min. Sense and antisense 6S RNA in vitro transcripts were used as positive and negative controls in Northern blot analyses. Full-length RNA probes (either sense or antisense 6S RNA) were internally labeled with digoxigenin-11-UTP according to the DIG RNA Labeling Mix protocol (Roche Diagnostics, Cat. No. 11277073910) for chemiluminescence detection. For Roseiflexus castenholzii HLO8, RNAs were transcribed from PCR templates, whereas linearized pUC18 plasmid derivatives were used as templates for T7 transcription of sense and antisense 6S RNAs of Sulfurihydrogenibium Y03AOP1. For primer sequences, see Supplemental Material.
Submitted Data
In the online Supplemental Material, start and stop positions of detected 6S RNAs in gff-format and their corresponding sequences in fasta-format are provided. Furthermore the alignments with secondary structure information are given. The Supplemental Material can be found at http://www.rna.uni-jena.de/supplements/6SRNA.
Disclosure of Potential Conflicts of Interest
No potential conflicts of interest were disclosed.
Acknowledgments
Roseiflexus castenholzii HLO8 was kindly provided by Robert E. Blankenship, Department of Biology and Chemistry, Washington University, St. Louis, MO 63130, USA. Sulfurihydrogenibium Y03AOP1 was kindly provided by Anna-Louise Reysenbach, Department of Biology, Portland State University, Portland, OR 97207-0751, USA.
Funding
This work was supported in part by DFG-Graduiertenkolleg 1384 “Enzymes and multienzyme complexes acting on nucleic acids” (SW, KD, RKH, MM), and DFG project MA-5082/1 (SW, MM). MM was funded by the Carl-Zeiss-Stiftung.
References
- Hindley J. Fractionation of 32P-labelled ribonucleic acids on polyacrylamide gels and their characterization by fingerprinting. J Mol Biol 30, 1967:125-136.
- Brownlee GG. Sequence of 6S RNA of E. coli. Nat New Biol 229, 1971:147-149.
- Wassarman KM, Storz G. 6S RNA regulates E. coli RNA polymerase activity. Cell 101, 2000:613-623.
- Cavanagh AT, Klocko AD, Liu X, Wassarman KM. Promoter specificity for 6S RNA regulation of transcription is determined by core promoter sequences and competition for region 4.2 of sigma70. Mol Microbiol 67, 2008:1242-1256. doi:10.1111/j.1365–2958.2008.06117.x
- Gildehaus N, Neusser T, Wurm R, Wagner R. Studies on the function of the riboregulator 6S RNA from E. coli : RNA polymerase binding, inhibition of in vitro transcription and synthesis of RNA-directed de novo transcripts. Nucleic Acids Res 35, 2007:1885-1896. doi:10.1093/nar/gkm085
- Trotochaud AE, Wassarman KM. 6S RNA function enhances long-term cell survival. J Bacteriol 186, 2004:4978-4985. doi:10.1128/JB.186.15.4978-4985.2004
- Trotochaud AE, Wassarman KM. 6S RNA regulation of pspFtranscription leads to altered cell survival at high pH. J Bacteriol 188, 2006:3936-3943. doi:10.1128/JB.00079–06
- Cavanagh AT, Wassarman KM. 6S-1 RNA function leads to a delay in sporulation in Bacillus subtilis. J Bacteriol 195, 2013:2079-2086. doi:10.1128/JB.00050–13
- Wassarman KM, Saecker RM. Synthesis-mediated release of a small RNA inhibitor of RNA polymerase. Science 314, 2006:1601-1603. doi:10.1126/science.1134830
- Beckmann BM, Hoch PG, Marz M, Willkomm DK, Salas M, Hartmann RK. A pRNA-induced structural rearrangement triggers 6S-1 RNA release from RNA polymerase in Bacillus subtilis. EMBO J 31, 2012:1727-1738. doi:10.1038/emboj.2012.23
- Steuten B, Wagner R. A conformational switch is responsible for the reversal of the 6S RNA-dependent RNA polymerase inhibition in Escherichia coli. Biol Chem 393, 2012:1513-1522
- Barrick JE, Sudarsan N, Weinberg Z, Ruzzo WL, Breaker RR. 6S RNA is a widespread regulator of eubacterial RNA polymerase that resembles an open promoter. RNA 11, 2005:774-784. doi:10.1261/rna.7286705
- Trotochaud AE, Wassarman KM. A highly conserved 6S RNA structure is required for regulation of transcription. Nat Struct Mol Biol 12, 2005:313-319. doi:10.1038/nsmb917
- Axmann IM, Kensche P, Vogel J, Kohl S, Herzel H, Hess WR. Identification of cyanobacterial non-coding RNAs by comparative genome analysis. Genome Biol 6, 2005. doi: 10.1186/gb-2005–6–9-r73
- Sharma CM, et al. The primary transcriptome of the major human pathogen Helicobacter pylori. Nature 464, 2010:250-255. doi:10.1038/nature08756
- Faucher SP, Friedlander G, Livny J, Margalit H, Shuman HA. Legionella pneumophila 6S RNA optimizes intracellular multiplication. Proc Natl Acad Sci U S A 107, 2010:7533-7538. doi:10.1073/pnas.0911764107
- Weissenmayer BA, Prendergast JG, Lohan AJ, Loftus BJ. Sequencing Illustrates the Transcriptional Response of Le-gionella pneumophila during Infection and Identifies Seventy Novel Small Non-Coding RNAs. PLoS One 6, 2011. doi: 10.1371/journal.pone.0017570
- Wassarman KM. Small RNAs in bacteria: diverse regulators of gene expression in response to environmental changes. Cell 109, 2002:141-144.
- Wu D, et al. A phylogeny-driven genomic encyclopaedia of Bacteria and Archaea. Nature 462, 2009:1056-1060. doi: 10.1038/nature08656
- Beckmann BM, Burenina OY, Hoch PG, Kubareva EA, Sharma CM, Hartmann RK. In vivo and in vitro analysis of 6S RNA-templated short transcripts in Bacillus subtilis. RNA Biol 8, 2011:839-849. doi:10.4161/rna.8.5.16151
- Cavanagh AT, Sperger JM, Wassarman KM. Regulation of 6S RNA by pRNA synthesis is required for efficient recovery from stationary phase in E. coli and B. subtilis. Nucleic Acids Res 40, 2012:2234-2246. doi:10.1093/nar/gkr1003
- Cabrera-Ostertag IJ, Cavanagh AT, Wassarman KM. Initiating nucleotide identity determines efficiency of RNA synthesis from 6S RNA templates in Bacillus subtilis but not Escherichia coli. Nucleic Acids Res 41, 2013:7501-7511. doi:10.1093/nar/gkt517
- Burenina OY, et al. Mechanistic comparison of Bacillus sub-tilis 6S-1 and 6S-2 RNAs–commonalities and differences. RNA 20, 2014:348-359.
- Axmann IM, Holtzendorff J, Voss B, Kensche P, Hess WR. Two distinct types of 6S RNA in Prochlorococcus. Gene 406, 2007:69-78. doi:10.1016/j.gene.2007.06.011
- Watanabe T, Sugiura M, Sugita M. A novel small stable RNA, 6Sa RNA, from the cyanobacterium Synechococcus sp. strain PCC6301. FEBS Lett 416, 1997:302-306.
- Rediger A, Geissen R, Steuten B, Heilmann B, Wagner R, Axmann IM. 6S RNA - an old issue became bluegreen. Microbiology 158, 2012:2480-2491. doi:10.1099/mic.0.058958–0
- Embley TM, Hirt RP, Williams DM. Biodiversity at the molecular level: the domains, kingdoms and phyla of life. Philos Trans R Soc Lond B Biol Sci 345, 1994:21-33. doi: 10.1098/rstb.1994.0083
- Stackebrandt E, Schumann P. Introduction to the taxonomy of actinobacteria. In M Dworkin, S Falkow, E Rosenberg, KH Schleifer, E Stackebrandt, editors, The Prokary-otes. Springer New York, 2006. ISBN 978–0–387–25493–7, 297-321. doi:10.1007/0–387–30743–516
- Gao B, Gupta RS. Phylogenetic framework and molecular signatures for the main clades of the phylum Acti- nobacteria. Microbiol Mol Biol Rev 76, 2012:66-112. doi: 10.1128/MMBR.05011–11
- Stach JE, Bull AT. Estimating and comparing the diversity of marine actinobacteria. Antonie Van Leeuwenhoek 87, 2005:3-9. doi:10.1007/s10482–004–6524–1
- Panek J, Krasny L, Bobek J, Jezkova E, Korelusova J, Vohradsky J. The suboptimal structures find the optimal RNAs: homology search for bacterial non-coding RNAs using suboptimal RNA structures. Nucleic Acids Res 39, 2011:3418-3426. doi:10.1093/nar/gkq1186
- Arnvig K, Young D. Non-coding RNA and its potential role in Mycobacterium tuberculosis pathogenesis. RNA Biol 9, 2012:427-436. doi:10.4161/rna.20105
- Vockenhuber MP, et al. Deep sequencing-based identification of small non-coding RNAs in Streptomyces coelicolor. RNA Biol 8, 2011:468-477
- Gupta RS, Bhandari V, Naushad HS. Molecular signatures for the PVC clade (Planctomycetes, Verrucomicro- bia, Chlamydiae, and Lentisphaerae) of bacteria provide insights into their evolutionary relationships. Front Microbiol 3, 2012:327-327. doi:10.3389/fmicb.2012.00327
- Lechner M, Nickel AI, Wehner S, Riege K, Wieseke N, Beckmann BM, Hartmann RK, Marz M. Genomewide comparison and novel ncRNAs of Aquificales. BMC Genomics 15, 2014:522. doi:10.1186/1471-2164-15-522
- Willkomm DK, Minnerup J, Huttenhofer A, Hartmann RK. Experimental RNomics in Aquifex aeolicus: identification of small non-coding RNAs and the putative 6S RNA homolog. Nucleic Acids Res 33, 2005:1949-1960.
- Dugar G, et al. High-resolution transcriptome maps reveal strain-specific regulatory features of multiple Campylobacter jejuni isolates. PLoS Genet 9, 2013. doi:10.1371/journal.pgen.1003495
- Hanada S, Takaichi S, Matsuura K, Nakamura K. Rosei-flexus castenholzii gen. nov., sp. nov., a thermophilic, filamentous, photosynthetic bacterium that lacks chlorosomes. Int J Syst Evol Microbiol 52, 2002:187-193.
- Collins AM, Xin Y, Blankenship RE. Pigment organization in the photosynthetic apparatus of Roseiflexus castenholzii. Biochim Biophys Acta 1787, 2009:1050-1056. doi:10.1016/j.bbabio.2009.02.027
- Reysenbach AL, et al. Complete and draft genome sequences of six members of the Aquificales. J Bacteriol 191, 2009:1992-1993.
- Pitulle C, et al. Phylogenetic position of the genus Hy- drogenobacter. Int J Syst Bacteriol 44, 1994:620-626.
- Oshima K, Chiba Y, Igarashi Y, Arai H, Ishii M. Phylogenetic position of Aquificales based on the whole genome sequences of six Aquificales species. Int J Evol Biol 2012, 2012:859264-859264.
- Wurm R, Neusser T, Wagner R. 6S RNA-dependent inhibition of RNA polymerase is released by RNA-dependent synthesis of small de novo products. Biol Chem 391, 2010:187- 196. doi:10.1515/BC.2010.018
- Steuten B, et al. Regulation of transcription by 6S RNAs: Insights from the Escherichia coli and Bacillus subtilis model systems. RNA Biol 11, 2014.
- Mitschke J, et al. An experimentally anchored map of transcriptional start sites in the model cyanobacterium Syne-chocystis sp. PCC6803. Proc Natl Acad Sci U S A 108, 2011:2124-2129. doi:10.1073/pnas.1015154108
- Steuten B, Schneider S, Wagner R. 6S RNA: recent answers–future questions. Mol Microbiol 91, 2014:641-648. doi:10.1111/mmi.12484
- Beckmann BM, Burenina OY, Hoch PG, Kubareva EA, Sharma CM, Hartmann RK. In vivo and in vitro analysis of 6S RNA-templated short transcripts in Bacillus subtilis. RNA Biol 8, 2011:839-849.
- Meier C, Carter LG, Winter G, Owens RJ, Stuart DI, Esnouf RM. Structure of 5-formyltetrahydrofolate cyclo-ligase from Bacillus anthracis (BA4489). Acta Crystallogr Sect F Struct Biol Cryst Commun 63, 2007:168-172. doi:10.1107/S1744309107007221
- Stover P, Schirch V. 5-Formyltetrahydrofolate polyglutamates are slow tight binding inhibitors of serine hydroxymethyltransferase. J Biol Chem 266, 1991:1543-1550
- Bertrand R, Jolivet J. Methenyltetrahydrofolate synthetase prevents the inhibition of phosphoribosyl 5- aminoimidazole 4-carboxamide ribonucleotide formyltransferase by 5-formyltetrahydrofolate polyglutamates. J Biol Chem 264, 1989:8843-8846.
- Low HH, Moncrieffe MC, Lowe J. The crystal structure of ZapA and its modulation of FtsZ polymerisation. J Mol Biol 341, 2004:839-852. doi:10.1016/j.jmb.2004.05.031
- Huang KH, Durand-Heredia J, Janakiraman A. FtsZ ring stability: of bundles, tubules, crosslinks, and curves. J Bacteriol 195, 2013:1859-1868. doi:10.1128/JB.02157–12
- Ammelburg M, Frickey T, Lupas AN. Classification of AAA+ proteins. J Struct Biol 156, 2006:2-11. doi:10.1016/j.jsb.2006.05.002
- Yaron A, Naider F. Proline-dependent structural and biological properties of peptides and proteins. Crit Rev Biochem Mol Biol 28, 1993:31-81. doi:10.3109/10409239309082572
- Li H, Fast W, Benkovic SJ. Structural and functional modularity of proteins in the de novo purine biosynthetic pathway. Protein Sci 18, 2009:881-892. doi:10.1002/pro.95
- Neusser T, Polen T, Geissen R, Wagner R. Depletion of the non-coding regulatory 6S RNA in E. coli causes a surprising reduction in the expression of the translation machinery. BMC Genomics 11, 2010:165-165. doi:10.1186/1471–2164–11–165
- Itaya M, Tsuge K, Koizumi M, Fujita K. Combining two genomes in one cell: stable cloning of the Synechocystis PCC6803 genome in the Bacillus subtilis 168 genome. Proc Natl Acad Sci U S A 102, 2005:15971-15976. doi: 10.1073/pnas.0503868102
- Griffiths-Jones S, Bateman A, Marshall M, Khanna A, Eddy SR. Rfam: an RNA family database. Nucleic Acids Res 31, 2003:439-441.
- Altschul SF, Gish W, Miller W, Myers EW, Lipman DJ. Basic local alignment search tool. Journal of Molecular Biology 215, 1990:403-410. ISSN 0022–2836. doi:10.1006/jmbi.1990.9999
- Hertel J, et al. Non-coding RNA annotation of the genome of Trichoplax adhaerens. Nucleic Acids Res 37, 2009:1602-1615. doi:10.1093/nar/gkn1084
- Nawrocki EP, Eddy SR. Infernal 1.1: 100-fold faster RNA homology searches. Bioinformatics 29, 2013:2933-2935. doi: 10.1093/bioinformatics/btt509
- Eddy SR. RNABOB: a program to search for RNA secondary structure motifs in sequence databases, 1992-1996. http://selab.janelia.org/software.html
- Will S, Reiche K, Hofacker IL, Stadler PF, Backofen R. Inferring Non-Coding RNA Families and Classes by Means of Genome-Scale Structure-Based Clustering. PLOS Computational Biology 3, 2007:e65. doi:10.1371/journal.pcbi. 0030065
- Griffiths-Jones S. RALEE–RNA ALignment editor in Emacs. Bioinformatics 21, 2005:257-259. doi:10.1093/bioinformatics/bth489
- Larkin MA, et al. Clustal W and Clustal X version 2.0. Bioinformatics 23, 2007:2947-2948. doi:10.1093/bioinformatics/btm404
- Bryant D, Moulton V. Neighbor-net: an agglomerative method for the construction of phylogenetic networks. Mol Biol Evol 21, 2004:255-265. doi:10.1093/molbev/msh018
- Huson DH, Bryant D. Application of phylogenetic networks in evolutionary studies. Mol Biol Evol 23, 2006:254-267. doi: 10.1093/molbev/msj030
- Hofacker IL, Fontana W, Stadler PF, Bonhoeffer LS, Tacker M, Schuster P. Fast folding and comparison of RNA secondary structures. Monatshefte fur Chemie Chemical Monthly 125, 1994:167-188. ISSN 0026–9247. doi:10.1007/BF00818163
- Punta M, et al. The Pfam protein families database. Nucleic Acids Res 40, 2012:290-301. doi:10.1093/nar/gkr1065
- Mattatall NR, Sanderson KE. Salmonella typhimurium LT2 possesses three distinct 23S rRNA intervening sequences. J Bacteriol 178, 1996:2272-2278