Figures & data
Figure 1 Antibiotics discovery with the development of resistance, overview of 20th and 21st centuries.
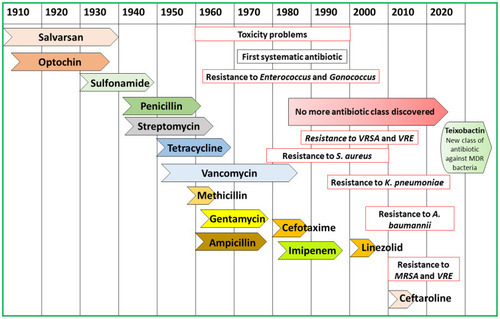
Figure 2 PubMed articles published on the topic of nanoparticles for antibacterial applications. The data was collected by using the keyword “antibacterial nanomaterials” in PubMed database about the articles published since 2010.
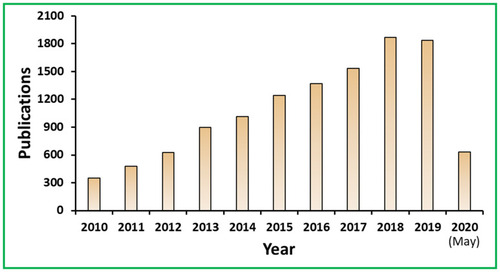
Figure 3 Schematic showing multifarious mechanisms of microbial resistance to develop MDR like decrease uptake of drug, an efflux of drug, target site changes, antibiotic modification, persister cells, swarming and biofilm formation.
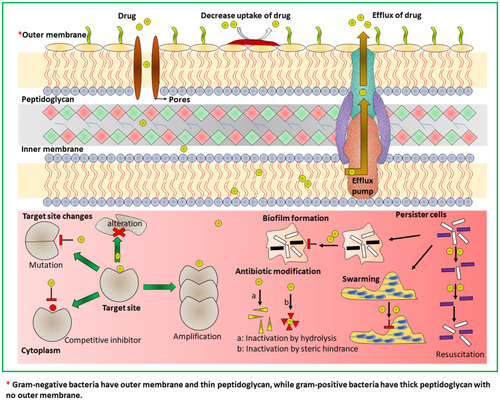
Figure 5 (A) Schematic approach for the preparation of gentamicin-NONOate nanoparticles via RAFT polymerization. (B and C) GEN-NO nanoparticles induced dispersal in P. aeruginosa biofilms. (B) Bacterial biofilms were grown in multi-well plates for 6 h in the absence of any treatment before being treated for a further 1 h with various concentrations (mM) of NO donor spermine NONOate (Sper-NO), free gentamicin or gentamicin-conjugated polymers (Poly-GEN) and GEN-NO nanoparticles (Poly-GENNO). Biofilm biomass was analyzed by crystal violet staining. Error bars represent standard error (n=2). (C) Stained biofilms treated with the indicated concentrations of GEN-NO nanoparticles. Note: concentration based on GEN, one mole of GEN-NO nanoparticles is equivalent to one mole of Sper-NO and gentamicin. (D) Representative confocal images showing P. aeruginosa biofilms stained with LIVE/DEAD kit. Biofilms were grown for 6 h and then treated with NO donor spermine NONOate (SperNO), free gentamicin, GEN-NO nanoparticles or left untreated for a further 1 h before staining. Viable and non-viable bacteria appear green and red, as well as those stained both green/red, respectively. Scale bar=50 mm. Note: concentration based on GEN, one mole of GEN-NO nanoparticles is equivalent to one mole of Sper-NO and gentamicin. Reproduced from Nguyen T-K, Selvanayagam R, Ho KKK, et al. Co-delivery of nitric oxide and antibiotic using polymeric nanoparticles. Chem Sci. 2016;7(2):1016–1027.Citation70 Creative Commons license and disclaimer available from: https://creativecommons.org/licenses/by/3.0/.
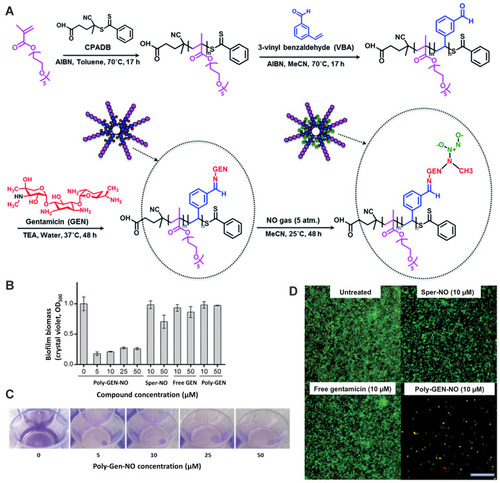
Figure 6 Effect of pH on the particle size (A) and zeta potential (B) of C-NPs and on the particle size (C) and zeta potential (D) of C/RL-NPs. Scheme of C-NPs and C/RL-NPs synthesis (E). Data collected from DLS. Reprinted with permission from Marangon CA, Martins VCA, Ling MH, et al. Combination of rhamnolipid and chitosan in nanoparticles boosts their antimicrobial efficacy. ACS Appl Mater Interfaces. 2020;12(5):5488–5499. Copyright (2020) American Chemical Society.Citation84
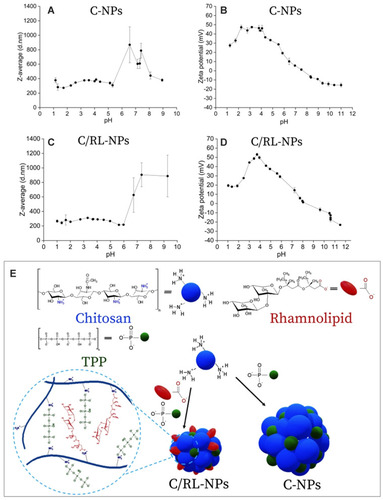
Figure 7 (A) Schematic diagram of chemical mechanism of Ag-NP toxicity to human monocytes (THP-1). Ag-NPs are internalized by cells and trafficked from engulfed vesicles to the lysosomes. Due to the acidic environment in the lysosome, Ag-NPs in the form of (Ag0)n are dissolved into Ag+ ions and then changed into Ag-O- form possibly due to the binding of organic acid molecules. Dissolved silver increases LMPs that leads to the release of both dissolved silver and lysosomal contents to the cytoplasm. Then, both the increased LMPs and the released silver decrease the mitochondrial membrane potentials, which results in ROS generation and cell apoptosis. Meanwhile, the dissolved silver (Ag-O-) will interact with cysteine-contained proteins like metallothioneins, enzymes, etc. to become Ag-S- speciation, which may trigger mitochondrion-involved apoptosis. However, a part of Ag-S- form may be exported by the membrane transporters to reduce cytotoxicity. To understand the chemical origin of Ag-NP cytotoxicity, two advanced techniques are powerful to illustrate the dynamic processes of intracellular Ag-NPs in time and space. SR-TXM can in situ study the intracellular accumulation and exocytosis of Ag-NPs, while SRXANES is capable of revealing the chemical transformation of silver from the oxidation and degradation to the Ag-S- form. (B) TEM image of Tween-20 dispersed Ag-NPs. (C) Hydrodynamic size distributions of 10 μg mL-1 Ag-NPs during 24 h in ddH2O and 10% FBS-supplemented RPMI 1640 medium. (D and E) Cytotoxicity of Ag-NPs and Ag+ ions. (D) Impacts of Ag-NPs, Ag+ ions, and the dispersant (Tween-20) on the mitochondrial dehydrogenase activity shown as cell viability vs the dose- and time-dependence. Data are shown as mean and standard deviation (n = 4). (E) Influence of Ag-NPs, Ag+ ions and Tween-20 on the percentage of live cells determined by Live-Dead assay. Reprinted with permission from Wang L, Zhang T, Li P, et al. Use of synchrotron radiation-analytical techniques to reveal chemical origin of silver-nanoparticle cytotoxicity. ACS Nano. 2015;9(6):6532–6547. Copyright (2015) American Chemical Society.Citation99
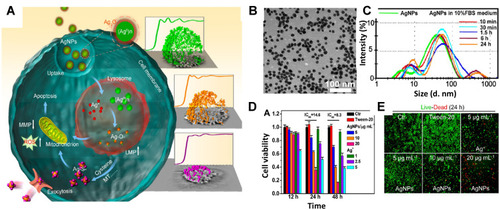
Figure 8 Schematic illustration of the fabrication of Ag@MSNs@LEVO nanoplatform and its application for a synergistic therapy of drug-resistant infections in vitro and in vivo. Reprinted from Biomaterials, Vol 101, Wang Y, Ding X, Chen Y, et al, Antibiotic-loaded, silver core-embedded mesoporous silica nanovehicles as a synergistic antibacterial agent for the treatment of drug-resistant infections, Pages No.207–216, Copyright (2016), with permission from Elsevier.Citation103
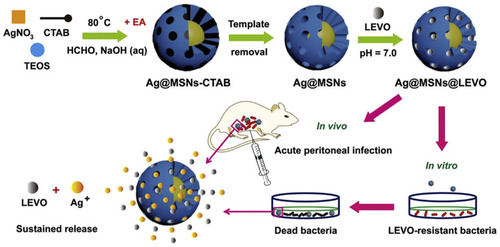
Figure 9 (A) Survival of S. aureus in ZnO-NP treated mice. Mice were infected intradermally with S. aureus and treated with ZnO-NPs simultaneously (S. aureus + ZnO NP) or 1 day after infection (S. aureus + 1d ZnO-NP). Mice injected with PBS and ZnO-NP alone were used as control. Seven days after infection, skin lesions were cut, homogenized and bacterial count was determined by CFU assay. (B) Histological appearance of mice skin biopsy; PBS treated, S. aureus infected, S. aureus infected and ZnONP treated and only ZnO-NP treated. On day 6, biopsy specimens were taken immediately after the termination of the experiment, fixed in 4% neutral buffered formalin and embedded in parafilm. The biopsy specimens were stained with hematoxylin and eosin. Numbered arrows indicate the following: 1, epidermis; 2, dermis; 3, sebaceous gland; 4, bacteria; 5, disrupted epidermal layer; 6, polymorphous infiltrate, consisting of mononuclear cells including lymphocytes and neutrophils. Experiments were performed in triplicates, results are shown mean ± SD; ***P ≤ 0.001. Adapted from Nanomedicine: Nanotechnology, Biology and Medicine, Vol 10/ Edition 6, Pati R, Mehta RK, Mohanty S, et al, Topical application of zinc oxide nanoparticles reduces bacterial skin infection in mice and exhibits antibacterial activity by inducing oxidative stress response and cell membrane disintegration in macrophages, Pages No.1195–1208, Copyright (2014), with permission from Elsevier.Citation112 (C) {101}-{001} surface heterojunction-promoted electron-hole spatial separation. The conduction band and valence band edges of {001} facet are higher than those of {101} facets, facilitating electrons to transfer from {001} to {101} facets and holes from {101} to {001} facets under sunlight irradiation. The {101}/{001} ratio can be adjusted to optimize the electron-hole separation efficiency, maximizing the electron and hole accumulation on {101} and {001} facets, respectively. (D–H) Oxidative stress responses of E. coli and S. aureus bacteria treated or untreated with various TiO2 nanocrystals under simulated sunlight irradiation. (D) Fluorescence images of DCF-stained bacteria showing intracellular ROS production; Cellular GSH levels in E. coli (E) or S. aureus (F) determined by the GSH-Glo assay; Lipid peroxidation assessment of E. coli (G) or S. aureus (H) based on a MDA method. Bacterial suspensions (1×106 CFU/mL) were incubated with 200 µg/mL of TiO2 nanocrystals for 2 h under simulated sunlight irradiation, followed by 6 h incubation at 37 °C on a rotary platform at a 180 rpm. Adapted with permission from Liu N, Chang Y, Feng Y, et al. {101}–{001} Surface heterojunction-enhanced antibacterial activity of titanium dioxide nanocrystals under sunlight irradiation. ACS Appl Mater Interfaces. 2017;9(7):5907–5915. Copyright (2017) American Chemical Society.Citation124
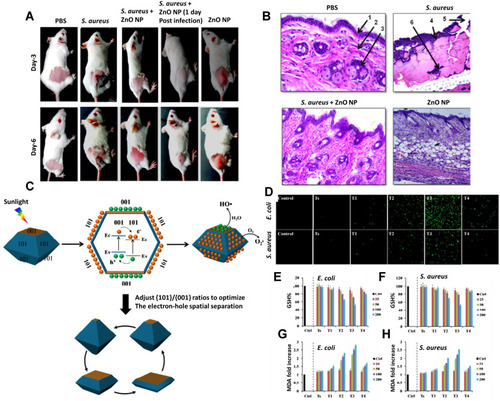
Figure 10 Schematic illustration of the contact killing of bacteria on a Cu-NP incorporated MI-dPG surface coating via the “attract-kill-release” route. Reprinted with permission from Li M, Gao L, Schlaich C, et al. Construction of functional coatings with durable and broad-spectrum antibacterial potential based on mussel-inspired dendritic polyglycerol and in situ-formed copper nanoparticles. ACS Appl Mater Interfaces. 2017;9(40):35411–35418. Copyright (2017) American Chemical Society.Citation118
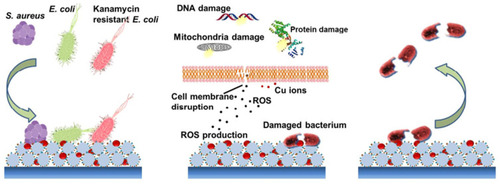
Figure 11 Illustration of a possible multivalent interaction between a Van-capped Au nanoparticle (2) and a VanA genotype VRE strain (hexagons: glycosides; ellipses represent the amino acid residues of the glycanpeptidyl precursor with different colors: L-Ala (yellow), D-Glu (orange), L-Lys (green), D-Ala (blue), and D-Lac (purple)). Reprinted with permission from Gu H, Ho PL, Tong E, Wang L, Xu B. Presenting vancomycin on nanoparticles to enhance antimicrobial activities. Nano Lett. 2003;3(9):1261–1263. Copyright (2003) American Chemical Society.Citation141
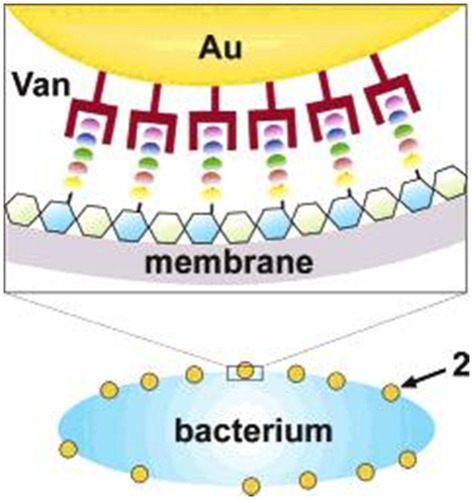
Figure 12 (A) Schematic principle of bacterial toxin-triggered antibiotic release from gold nanoparticle stabilized liposomes to treat toxin-secreting bacteria. Vancomycin-loaded liposomes are protected by absorbing chitosan-coated gold nanoparticles (AuCHT) onto their surface to prevent them from fusing with one another or with bacterial membranes. Once the AuCHT-stabilized liposomes (AuCHT-liposome) encounter bacterial toxins, the toxins will form pores in the liposome membranes and thus release the encapsulated antibiotics, which subsequently kill or inhibit the growth of the bacteria that secrete the toxins. (B) The surface ζ potential (mV) of bare liposome (without AuCHT) and AuCHT-liposome with a liposome/AuCHT molar ratio of 1:300. Adapted with permission from Pornpattananangkul D, Zhang L, Olson S, et al. Bacterial toxin-triggered drug release from gold nanoparticle-stabilized liposomes for the treatment of bacterial infection. J Am Chem Soc. 2011;133(11):4132–4139. Copyright (2011) American Chemical Society.Citation142 (C) Illustration of the synthesis of mixed-charge TMA/MUA nanoparticles. The relative compositions of these thiols in solution used for NP functionalization and on the resulting NPs are generally different, which is why the latter have to be determined independently by methods such as core etching followed by NMR. (D) Quantification of the NP charge polarities plotted against the composition of the mixed on-particle SAMs (composition expressed as XTMA:XMUA ratios as determined by core-etching/NMR analyses). The blue curve is for pH 11 and fully deprotonated MUAs; the red curve is for pH 7.4 (PBS buffer) under which conditions a small fraction of the MUAs is protonated (and hence the curve shifts slightly upwards compared to the one for pH 11). Error bars are based on three separate measurements. (E) Microscopic studies of bacteria treated with different types of mixed-charge NPs. Scanning electron microscopy (SEM) images of E. coli incubated with i) pure-MUA and ii) pure-TMA NPs. Inset in (B) shows TMA NPs adsorbing on the bacteria. For TEM images resolving the individual NPs. iii) Kelvin force microscopy (KFM) image of an E. coli bacterium illustrating its net negative surface potential of about 50 mV (blue horizontal line). iv) SEM, v) TEM, and vi) AFM images of E. coli after incubation with cTMA:cMUA=80:20 AuNPs evidencing the rupture of the bacterial cell wall. vii) TEM image showing cTMA:cMUA=80:20 AuNPs (small dark dots) associated with the intracellular material leaked from the bacterium upon lysis. Adapted from Angewandte Chemie International Edition, Vol 55/ Issue 30, Pillai PP, Kowalczyk B, Kandere-Grzybowska K, Borkowska M, Grzybowski BA, Engineering gram selectivity of mixed-charge gold nanoparticles by tuning the balance of surface charges, Pages No.8610–8614, Copyright (2016), with permission from John Wiley and Sons. © 2016 WILEY‐VCH Verlag GmbH & Co. KGaA, Weinheim.Citation143
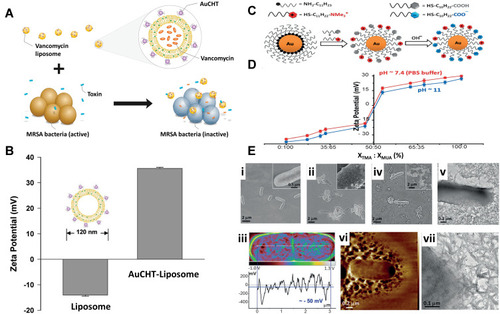
Figure 13 (A) Schematic illustration of inhalable particles as carriers for delivery of drug loaded nanoparticles to the deep lung. (B) Scanning microscopic images of microparticles made from mannitol and leucine using a spray-drying process. The microparticles were loaded with nanoparticles made from glyceryl monostearate and soybean phosphatidylcholine with a double emulsion process. (C) A zoomed-in image of (B). (D) Fluorescence images of lungs from untreated rat (E) and rat after intrapulmonary delivery of microparticles fluorescein-labeled nanoparticles. (F) Schematic illustration of a nanoparticle-hydrogel hybrid (NP-gel) system with tissue adhesive properties for localized antibiotic delivery under flow conditions. In this design, dopamine methacrylamide (DMA) containing catechol functional group was conjugated into gel matrix for adhesion. (G) NP-gel was tested for adhesion under a flow (shear stress = 3.2 Pa) on E. coli bacterial film (green: nanoparticles in the gel; bacteria: red), HEK 293T cell monolayer (blue: cell nuclei; green: nanoparticles in the gel), and shaved mouse skin. (H) E. coli biofilm formation when the bacteria were treated with PBS, blank gel (gel without nanoparticles or ciprofloxacin), free ciprofloxacin, ciprofloxacin loaded nanoparticles (without hydrogel), and ciprofloxacin-loaded NP-gel (scale bar = 5 mm). Reprinted from Advanced Drug Delivery Reviews, Vol 127, Gao W, Chen Y, Zhang Y, Zhang Q, Zhang L, Nanoparticle-based local antimicrobial drug delivery, Pages No.46–57, Copyright (2018), with permission from Elsevier.Citation184
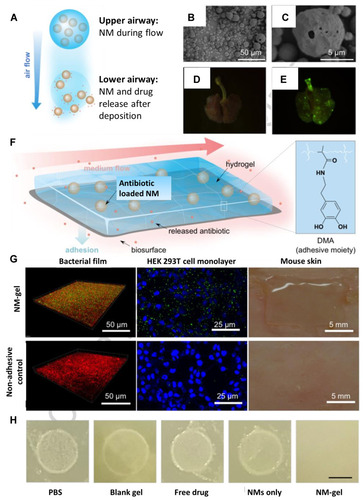
Figure 14 Antimicrobial materials potential to combat different infections in several organs of the human body.
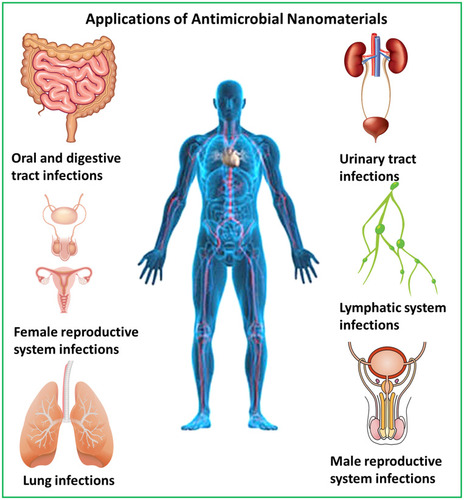
Figure 15 Methods to improve the biological action of metal nanomaterials. (A) Nanomaterials surface modification by biological substances. (B) Green synthesis of metal nanomaterials.
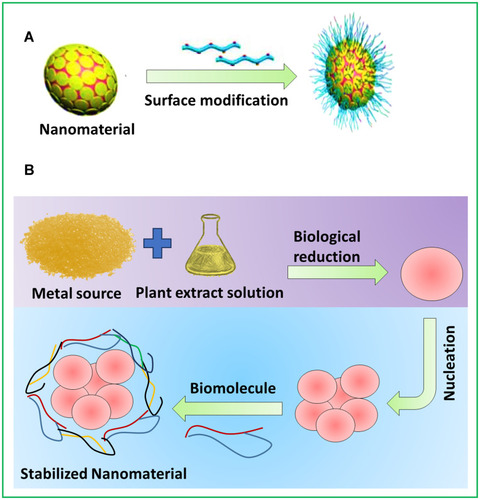