Abstract
Effect of electromagnetic fields (EMF) on some growth parameters, protein content and genetic variations were investigated in early vegetative growth of Brassica napus L. (canola) and Zea mays L. (maize). The seeds of both plants were exposed to EMF treatments. Maize seeds were treated with 0, 3 and 10 mT for 4 h exposure time, and canola seeds were treated with 0, 1 mT for 1 h and 7 mT for 3 h, respectively. Results showed that seed germination increased significantly in maize, and decreased in canola seeds. Root length, shoot length, fresh weigh and dry weight increased significantly in maize and canola as compared to control. Total protein content in maize significantly increased at 3 mT and then decreased in 10 mT for 4 h, but in canola increased protein content under EMF treatments. RAPD analysis showed 104 total bands of 14 primers in maize, out of which 72 bands (about 69.2%) were polymorphic bands, and 65 polymorphic bands (51.6%) in canola were determined from a total of 126 bands. Primers showing maximum number of polymorphic bands were OPM-11 and OPI-07 in maize and OPA-10 in canola. It was found that seed pretreatment to low density of EMF could be used for improving growth and genetic variations in maize and canola plants.
Introduction
Germplasm diversity is of concern to breeders as extensive use of closely related cultivars results in vulnerability to pests and diseases. Electromagnetic fields (EMF) are an abiotic stress that can affect genetic diversity and resistance to environmental stress in plants. Mechanisms of EMF adaptation are not yet completely clear but can be explained to some extent by stress adaptation effectors such as increase in free radicals and antioxidant enzyme activities, delay of senescence, phosphoinositide breakdown, changing the mitosis control mechanisms and increase in the percentages of chromosomal aberrations (Gutzeit Citation2001; Ruiz-Gomez and Martinez-Morillo Citation2009; Focke et al. Citation2010; Blank and Goodman Citation2011).
Identification of the molecular mechanisms for plant tolerance to environmental stress is important. Molecular markers can show the direct effect of stress on genotypes at the DNA level. Random amplified polymorphic DNA (RAPD) may potentially form the basis of novel biomarker assays for the detection of DNA damage and mutational events in plants (Savva Citation1996). RAPD markers have been used to study genetic diversity and genotype assessment in several plant species (Bautista et al. Citation2003; Graciele et al. Citation2008). Majd et al. (Citation2013) showed that Satureja hortensis plants grown from wet and dry pretreated seeds with 4 mT for 120 min induced genetic variation compared to control plants.
Zea mays L. (maize) is the most important food crop belonging to the Poaceae family, and is used as a material for many industrial products such as starch and oil in Iran (Bray et al. Citation2000). Brassica napus L. (canola) is an important oil seed crop belonging to the Brassicaceae family. Canola has valuable fatty acids and amino acids required for the human body (Bybordi Citation2010). Our previous work showed that EMF treatment changed protein content, antioxidant activity, enzymes and genetic recombination in maize (Shabrangi et al. Citation2011). In this study, we try to identify the effects of EMF on physiological and molecular responses in two economically important plant species of canola and maize by using RAPD markers.
Materials and methods
Plant materials and electromagnetic field exposure
Seeds of Z. mays L. (genotype = single cross 704) and B. napus (Zarfam genotype) were obtained from the Seed and Plant Improvement Institute, Karaj, Iran, and were selected for a uniform size, shape and equal average weight. Three replicates were used in the experiment with 30 seeds in each treatment. The wet and dry seeds were spread on the moist filter papers in Petri dishes and then placed in the middle of a horizontally fixed coil (Figure ). Exposure to EMFs was performed using an EMF generator (Shabrangi et al. Citation2010). In preliminary experiments, the wet and dry seeds were exposed to EMFs by a magnitude of 1, 3, 5, 7 and 10 mT over 1–4 h exposure times. After seven days, seedlings were analyzed for growth parameters. In maize seedlings, the highest growth rate was obtained with 3 mT for 4 h for wet seeds, and the lowest growth was obtained with 10 mT for 4 h for wet seeds. On the other hand, seedlings of canola showed the highest and lowest growth with 1 mT for 1 h, and 7 mT for 3 h in wet seeds, respectively (Shabrangi and Majd Citation2009). Hence, these treatments were used for RAPD analysis and some growth parameters. Three replicates, with 30 seeds in each were used for repetition. Seeds exposed to different intensity of magnetic fields in maize (0, 3 and 10 mT for 4 h), and canola (0 and 1 mT for 1 h and 7 mT for 3 h). Then seeds were placed in a seed germination chamber at 23°C with a photo period of 14 h day/10 h dark, and watered daily at relative humidity of 20%. Two weeks after EMF treatments, four plants per treatment (four replicates) were collected for physiological and RAPD analyses.
Protein extraction and assay
For estimation of total protein content, 0.5 g of fresh aerial part (leaf and shoot) was homogenized at 4°C with a mortar in 1 M Tris–HCl (pH 6.8) and PVPP (1%). The homogenates were centrifuged at 13,000 g for 30 min twice at 4°C using a Heraeus 400R microfuge. Supernatant was kept at −70°C and used for protein determination and enzyme assay. Protein concentration was measured according to Bradford (Citation1976), using bovine serum albumin (BSA) as standard. Five milliliters of the Bradford reagent and 100 μl of the each protein extract were mixed and then reaction mixtures were incubated at room temperature for 20 min. The absorbance values were measured at 595 nm using UV-visible spectrophotometer (UV-160, Shimadzu, Tokyo, Japan).
RAPD analysis
For RAPD analysis, fresh leaves of 14-day-old seedlings were selected randomly from the control plants and plants generated from treated seeds, and DNA extraction was done using a NucleoSpin Plant kit (Macherey-Nagel, Duren, Germany). The PCR reaction mixture consisted of template DNA, 1× PCR buffer (10 mM Tris-HCJ pH 8.8, 250 mM KC12), 200 μM dNTPs, 0.80 μM 10-base random primers (Table ) and 1 unit of Taq polymerase, in a total volume of 25 μl. DNA amplification was performed on a palm cycler GP-001 (Corbett Research, Calamvale, QLD, Australia).
Table 1. Results of RAPD analysis of maize and canola plants under electromagnetic field treatment.
Template DNA was initially denatured at 92°C for 3 min, followed by 35 cycles of PCR amplification under the following parameters: denaturation for 1 min at 92°C, primer annealing for 1 min at 36°C and primer extension for 2 min at 72°C. A final incubation for 10 min at 72°C was performed to ensure that the primer extension reaction proceeded to completion. The PCR amplified products were separated by electrophoresis on a 2% agarose gels using 0.5× TBE buffer (44.5 mM Tris/Borate, 0.5 mM EDTA, pH = 8) or 12% polyacrylamide gels. The gels were stained with ethidium bromide and visualized under UV light or silver stained for added sensitivity. Thirty-one random primers supplied by Operon technology (Alameda, CA, USA) were used.
Statistical analysis
The growth parameters and protein content were analyzed by one-way analysis of variance (ANOVA) using SPSS, version 18 (Chicago, IL, USA) with four replications for every treatment at the level of p ≤ 0.05. The mean differences were compared by the lowest standard deviations (LSD) test. RAPD bands obtained were treated as binary characters and coded accordingly (presence = 1, absence = 0). Simple matching coefficient was determined (Podani Citation2000), and grouping of the genotypes was done by clustering methods based on principal coordinate analysis (PCO) from NTSYSpc (Numerical Taxonomy and Multivariate Analysis System), version 2.02 (Exeter Biological Software, Setauket, NY, USA) program (Rohlf, Citation1998).
Results
Growth and protein content
Seed germination significantly increased under EMF treatment in maize (Table ). The increase of 68.4 and 43.1% of seed germination were observed with 3 mT and 10 mT for 4 h in maize as compare to control, respectively. Growth parameters increased significantly under EMF treatments in maize (Figure ). The highest growth parameters were observed with the 3 mT treatment, and an increase of 168.5, 48.3, 35.8 and 42.3% were identified for root length, shoot length, fresh and dry weight, respectively, as compared to control. In contrast to maize, EMF treatments decreased seed germination in canola. With 1 mT, the fresh and dry weight significantly increased (Figure ), but these parameters decreased slightly with 7 mT for 3 h as compared to control. Total protein content in maize showed a 22.7% increase at 3 mT and 12.9% decrease at 10 mT as compared to control. In canola, the protein content showed 75.5% and 46.03% increases at 3 and 7 mT, respectively (Table ).
Table 2. Effect of EMF treatment on some growth parameters and protein content in maize (M1–M3) and canola (C1–C3) seedlings.
RAPD analysis
Of 31 RAPD primers, 14 produced some bands. In maize, 104 bands were obtained, of which 72 were polymorph (69.2%) and 32 bands were monomorph (29.7%, Table ). In canola, 126 bands were obtained of which 65 bands were polymorph (51.6%) and 61 bands were monomorph (48.04%, Table ).
Primer OPR-02 produced the highest number of bands in both canola (14 bands) and maize (13 bands), while primer OPC-12 (four bands) in canola, and primer OPM-10 (three bands) in maize produced the lowest number of bands (Table ). The average number of polymorphic bands per primer varied from one to nine in maize and one to 10 in canola. The primers OPI-07 and OPM-11(9 bands) in maize and OPA-10 (10 bands) in canola showed the highest number of polymorphic bands (Table ). The highest percentage of polymorphic bands was recognized for primers OPI-07(90%) and OPM-11 (100%) in maize and OPA-10 (90.9%) in canola, while the lowest percentage in maize and canola was recognized for primers OPH-07 (12.5%) and OPM-11 (9.09%), respectively (Table ).
In the three treatments of maize, there were bands occurring in two treatments and missing in one. For example, bands of 150–500 bp in OPA-10, and bands of 500–1000 bp in primer OPA-13, were absent in plants grown from the seeds exposed to 10 mT treatment and existed in other treatments. Primers OPC-01 (Figure B), OPC-05 and OPC-06 also showed missing bands only in the 10 mT treatment and primer OPR-02 showed two bands of 400, 450 bp with 10 mT (Figure A). Some specific bands were produced with a 3 mT treatment of maize for 4 h, for example bands of 900, 950, 1200, 1400, 1700 bp in OPC-08 (Figure A), and five bands of 300, 400, 550, 1400 and 1500 bp in OPR-08 (Figure B). Few specific bands occurred in control plants of maize, for example, a 1500 bp band in primer OPC-04, and bands with 250, 500 and 1500 bp in OPI-07 primer. Unweighted pair group method with arithmetic averages (UPGMA) clustering and PCO plot revealed a genetic difference between control and seedlings generated from treated seeds with 3 and 10 mT for 4 h (Figures ).
Figure 3. Effect of different EMF treatments on RAPD profiles of maize and canola seedlings by (A) OPC-08 and (B) OPC-01 primers, respectively. Control (M1), 3 mT (M2) and 10 mT (M3) for 4 h in maize, control (C1), 1 mT for 1 h (C2) and 7 mT for 3 h (C3) in canola.
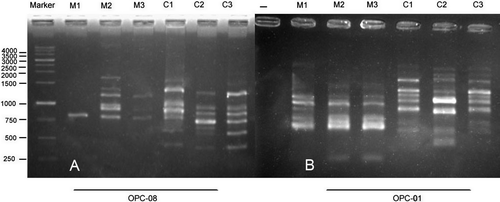
Figure 4. Effect of different EMF treatments on RAPD profiles of maize and canola seedlings by (A) OPR-02 and (B) OPR-08 primers, respectively. Control (M1), 3 mT (M2) and 10 mT (M3) for 4 h in maize, control (C1), 1 mT for 1 h (C2) and 7 mT for 3 h (C3) in canola.
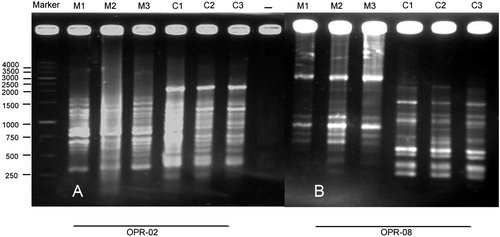
Figure 5. Dendrogram resulting from UPGMA cluster and POC plot based on RAPD data from control, 3 and 10 mT for 4 h in maize.
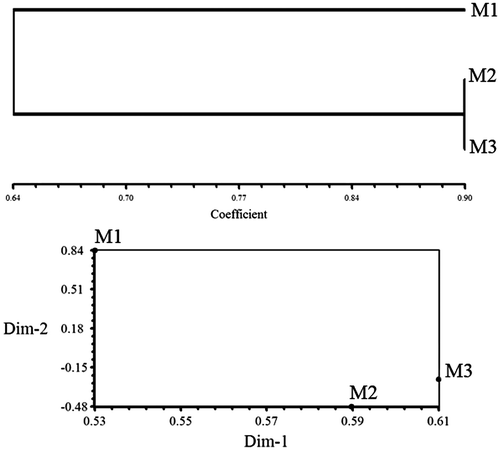
In canola, bands with molecular weights of 500, 750, 900 and 1000 bp in OPA-10, three bands with 450, 1700 and 2000 bp in OPC-01 (Figure B) and a 250 bp band in OPC-05 primer were specific for plants grown from the seeds exposed to 1 mT for 1 h treatment. Some specific bands also occurred in seedlings grown from the seeds exposed to 7 mT for 3 h treatment, for example four bands with 250, 1000, 1500 and 2000 bp in OPC-05, a 1000 bp band in OPR-02 (Figure A) primer.
Few bands were specific for the control plants: three bands with 1500, 2000 and 5000 bp in OPC-12, a 750 bp band in OPC-05 and 1600 bp band in OPC-08 primer. UPGMA clustering and PCO ordination plot separated the plants generated from treated seeds far from control plants due to their molecular difference (Figure ).
Discussion
Seed germination and growth parameters showed a considerable improvement in maize, and a significant decrease of seed germination in canola under EMF treatments. Root and shoot length also increased in both maize and canola seedlings (Table ). The low intensity of electromagnetic field (20 mT) showed a significant increase in the fresh and dry weight of Helianthus annuus seedling (Fischer et al. Citation2004). Seeds exposed to 100 and 200 mT for 2 h showed a significant increase in growth, photosynthesis and chlorophyll content in Indian maize (Anand et al. Citation2012). Electromagnetic fields can induce changes at a cellular level leading to increase in cell viability, organization and differentiation, and cell metabolism (Vizcaino Citation2003; Valiron et al. Citation2005). EMF treatment also induced protein synthesis in the root system (Goodman et al. Citation1993). It seems that the increase in root and shoot length may be related to cell metabolism and protein induction under EMF treatment in canola and maize seedlings.
The protein content significantly increased at 1 and 7 mT in canola and 3 mT in maize after 14 days planting (Table ). Similar results were observed in wheat (Triticum aestivum L.) with a 0.3 T magnetic field after 21 days of planting (Elbeshehy and Almaghrabi Citation2013). Moon and Chunge (Citation2000) reported that magnetic field treatments can induce reactive oxygen species and influence the biochemical process by stimulating protein synthesis and enzyme activity. Our previous work showed the protein content of maize decreased at 3 and 10 mT after seven days (Shabrangi et al. Citation2011), but in this work, protein content increased at 3 mT after 14 days. It seems that protein accumulation after 14 days may be related to the synthesis of stress tolerance proteins and alleviation of stress conditions.
The presence of polymorphic bands, specific bands and missing bands in both maize (72 bands) and canola (65 bands) under EMF treatments indicates the presence of genetic polymorphism (Table ). Several studies showed that EMF treatments can induce antioxidant enzyme activity and reactive oxygen species in plant cells (Gupta et al. Citation1993; Tkalec et al. Citation2005; Shabrangi et al. Citation2011). EMF is a physical mutagen which dissociates the atoms of water molecules and creates hydroxyl radicals (Leibovitz and Siegel Citation1980). They interact with biomolecules including DNA and protein, and then scavenge electrons from them. Biomolecule oxidation by the radicals would damage their structure and biological activity. Our previous work showed that EMF treatment with the magnitude of 3 and 10 mT for 4 h increased significantly catalase and ascorbate peroxidaes activities and induced oxidative stress after seven days in maize (Shabrangi et al. Citation2011). Oxidative DNA damage can affect the DNA methylation patterns (Gutzeit Citation2001; Franco et al. Citation2008; Campisi et al. Citation2010).
Minasbekyan and Abovyan (Citation2013) showed that electromagnetic irradiation changed DNA methylation and induced nucleotide variations and appearance of new bands in wheat seedlings. The occurrence of genetic changes in the genome of plants can show with the loss or rearrangement of some nucleotides (Sushir et al. Citation2008). Our previous work showed a significant difference of meiotic characters in EMF pretreated plants as compared to control. Cytological abnormalities such as chromosome stickiness and micronuclei formation were observed in EMF pretreated canola plants (Shabrangi et al. Citation2010). On the other hand, the significant DNA fragmentation (damage) could be due to leaks in the membranes surrounding lysosomes which release digestive enzymes such as DNAase and may explain the damage done to DNA after exposing to electromagnetic field (Diem et al. Citation2005; Panagopoulous et al. Citation2007). Although in this work we did not investigate DNA methylation and DNAase content, it can be assumed that the presence of polymorphic bands may be related to nucleotide variations and genetic polymorphism under EMF treatment.
Results of clustering in both maize and canola genotypes indicate that plants generated from treated seeds differ extensively in their genetic content as they stand far from control plants in UPGMA dendrograms (Figures and ). This shows that the effect of EMFs on genetic structure of plants may be of great use in crop breeding and introducing genetic variations in the available germplasm.
Conclusion
Our study showed that pretreatment of seeds with EMF induced changes in shoot and root length, fresh and dry weight and protein content in maize and canola. Polymorphic bands and genetic variation were induced significantly under EMF treatments in both maize and canola. Treatments of 1 mT in canola and 3 mT in maize induced both growth parameters and genetic variation. This suggests that these intensities of EMF can be used as a tool for environmental stress alleviation in these plants. Further work on the effect of EMF treatments in the second generation is required to gain more information about these genetic variations and the growth of these plants.
Disclosure statement
No potential conflict of interest was reported by the authors.
Additional information
Funding
References
- Anand A, Nagarajan S, Verma AP, Jshi DK, Pathak PC, Bhardwaj J. 2012. Pre-treatment of seeds with static magnetic field ameliorates soil water stress in seedlings of maize (Zea mays L.). Indian J Biochem Biophys. 49(1):63–70.
- Bautista R, Canovas FM, Claros MG. 2003. Genomic evidence for a repetitive nature of the RAPD polymorphisms in Olea europaea (olive-tree). Euphytica. 130(2):185–190.
- Blank M, Goodman R. 2011. DNA is a fractal antenna in electromagnetic fields source. Int J Rad Biol. 87(4):409–415.
- Bradford MM. 1976. A rapid and sensitive method for the quantitation of microgram quantities of protein utilizing the principles of protein-dye binding. J Anal Biochem. 72(7):248–254.
- Bray EA, Bailey-Serres J, Weretilnyk E. 2000. Responses to abiotic stresses. In: Gruissem W, Buchannan B, Jones R, editors. Biochemistry and molecular biology of plants. Rockville, MD: American Society of Plant Physiology; p. 1158–1249.
- Bybordi A. 2010. Effects of salinity on yield and component characters in canola (Brassica napus L.) cultivars. Notulae Sci Biol. 2(1):81–83.
- Campisi A, Gulino M, Acquaviva R. 2010. Reactive oxygen species levels and DNA fragmentation on astrocytes in primary culture after acute exposure to low intensity microwave electromagnetic field. Neuroscience Lett. 473(1):52–55.
- Diem E, Schwarz C, Adlkofer F, Jahn O, Rüdiger H. 2005. Non-thermal DNA breakage by mobilephone radiation (1800 MHz) in human fibroblasts and in transformed GFSH-R17 rat granulosa cells in vitro. Mutat Res. 583(2):178–183.
- Elbeshehy EKF, Almaghrabi OA. 2013. Effect of weak electro-magnetic treatments on seedling growth and protein properties of different wheat (Triticum aestivum L.) cultivars. J Biotech Sci. 1(1):1–14.
- Fischer G, Tausz M, Kock M, Grill D. 2004. Effects of weak 16 2/3 Hz magnetic fields on growth parameters of young sunflower and wheat seedlings. Bioelectromagnetics. 25(8):638–641.
- Focke F, Schuermann D, Kuster N, Schar P. 2010. DNA fragmentation in human fibroblasts under extremely low frequency electromagnetic field exposure. Mutat Res Fundament Mol Mech Mut. 683(1–2):74–83.
- Franco R, Schoneveld O, Georgakilas AG, Panayiotidis M. 2008. Oxidative stress. DNA methylation and carcinogenesis. Cancer Lett. 266(1):6–11.
- Goodman EM, Greenebaum B, Marron MT. 1993. Altered protein synthesis in a cell-free system exposed to a sinusoidal magnetic field. Biochimica Biophys Act. 1202(1):107–112.
- Graciele S, Pipolo VC, Ruas CF, Carvalho VP. 2008. Comparative analysis of genetic diversity among the inbreed lines (Zea mays L.) obtained by RAPD and SSR Markers. Brazil Arch Biol Technol. 51(1):183–192.
- Gupta AS, Webb RP, Holaday AS, Allen RD. 1993. Overexpression of superoxide dismutase protects plants from oxidative stress. Plant Physiol. 103(4):1067–1073.
- Gutzeit HO. 2001. Biological effects of ELF-EMF enhanced stress response: New insights and new questions. Electro Magnet. 20(1):15–26.
- Leibovitz B, Siegel BV. 1980. Aspects of free radical reactions in biological systems. Aging J Gerontol. 35(1):45–56.
- Majd A, Singh C, Arbabiyan S, Salimpour F, Angaji SA. 2013. Effects of extremely low frequency electromagnetic fields on growth and genetic diversity of (Satureja hortensis L.). Ann. Biol Res. 4(6):41–45.
- Minasbekyan LA, Abovyan MS. 2013. Changes in DNA methylation wheat seedlings in first and second generations under influence of EHF EMI. Prog Electromagn Res Symp Proc. 12–15.
- Moon JD, Chung HS. 2000. Acceleration of germination of tomato seed by applying AC electric and magnetic fields. Electrostatics. 48(2):103–114.
- Panagopoulos DJ, Chavdoula ED, Nezis IP, Margaritis LH. 2007. Cell death induced by GSM 900-MHz and DCS 1800-MHz mobile telephony radiation. Mutat Res. 628(1–2):69–78.
- Podani J. 2000. Introduction to exploration of multivariate biological data. English translation. Leiden: Backhuys.
- Rohlf FJ. 1998. NTSYSpc: numerical taxonomy and multivariate analysis system. Version 2.02. Setauket (NY): Exeter Software.
- Ruiz-Gomez MJ, Martinez-Morillo M. 2009. Electromagnetic fields and the induction of DNA strand breaks. Electromagnetic Biol Med. 28(2):201–214.
- Savva D. 1996. DNA fingerprinting as a biomarker assay in ecotoxicology. Toxicol and Ecotoxicol News Rev. 3:110–114.
- Shabrangi A, Majd A. 2009. Comparing effects of electromagnetic fields (60 Hz) on seed germination and seedling development in monocotyledons and dicotyledons. Proc Prog Electromagn Res Symp. 704–709.
- Shabrangi A, Majd A, Sheidai M. 2011. Effects of extremely low frequency electromagnetic fields on growth, cytogenetic, protein content and antioxidant system of Zea mays L. African J Biotech. 10(46):9362–9369.
- Shabrangi A, Sheidai M, Majd A, Nabiuni M, Dorranian D. 2010. Cytogenetic abnormalities caused by extremely low frequency electromagnetic fields in canola. Science Asia. 36:292–296.
- Sushir KV, Mehetre SS, Patil SC, Kamdi SR. 2008. RAPD and cytogenetic study in F1 and F2 of inter specific cross between Gossypium arboretum and Gossypium anomalum. Cytologia. 73(3):213–219.
- Tkalec M, Malarić K, Pevalek-Kozlina B. 2005. Influence of 400, 900 and 1900 MHz electromagnetic fields on Lemna minor growth and peroxidase activity. Bioelectromagnetics. 26(3):185–193.
- Valiron O, Peris L, Rikken G, Schweitzer A, Saoudi Y, Remy C, Job D. 2005. Cellular disorders induced by high magnetic fields. J MRI. 22(3):334–340.
- Vizcaino V. 2003. Biological effects of low frequency electromagnetic fields. Radiobiologia. 3:44–46.