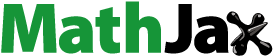
ABSTRACT
Efforts to combat the harmful effects of vehicle emissions on our environment and health include exhaust emissions and fuel efficiency regulations. One promising solution is direct fuel injection in supercritical conditions, where the fuel mixture is above critical temperature and pressure. This study presents a reduced kinetics mechanism (129 species and 1015 reactions) utilizing real gas equations to simulate the combustion of supercritical/transcritical gasoline surrogate/ethanol blends. It was developed by combining two reduced models – a Toluene reference fuel (TRF) with a Diisobutylene (DIB) and an ethanol model – and validated through simulations of ignition delay time (IDT). The Arrhenius parameters of key reactions for each fuel component were modified to improve accuracy. An adjusted Redlich-Kwong cubic state equation was employed to segregate each surrogate mixture tested as supercritical, transcritical, or subcritical. The new mechanism was then validated against experimental results using high-pressure conditions and the cubic Peng-Robinson and Redlich-Kwong equation of state parameters. Critical properties of each species were obtained through Joback’s Group Method and the Ambrose-Walton vapor pressure equation. The TRF/DIB/E mechanism results were consistent with shock tube experimental data at high pressure, indicating a potential for modeling combustion in ultra-high pressure direct injection engines.
Introduction
The growth of vehicle logistics and fossil fuel consumption has rapidly increased due to social development, particularly in large cities. The focus now is reducing the impact of air pollution and vehicle emissions on climate change and population health (Li, Williams, and Guo Citation2016). Harmful pollutants such as HC, NOx, CO, and Particulate Matter (PM) are studied in vehicle emissions, which permanently threaten human health and atmospheric quality (Elfasakhany Citation2014).
Clean and efficient combustion systems must comply with gas emission and fuel efficiency regulations. Improving internal combustion engines’ fuel economy, combustion, and emission performance remains vital.
An efficient way to enhance the performance of gasoline engines is by using gasoline direct injection (GDI) technology (Binder et al. Citation2015; Iwamoto et al. Citation1997). However, according to Zhang et al. (Citation2022), GDI has a shorter mixing time, which can cause partial uneven mixing and lead to severe particulate matter (PM) emission problems. Therefore, reducing PM emissions and enhancing the thermal efficiency of GDI engines remain the main challenges (Bokor et al. Citation2021; Piock et al. Citation2011; Zong et al. Citation2004). A proposed way to address these issues is using fuel or a mixture of fuels at a supercritical state in GDI engines (Boer et al. Citation2010, Citation2013; Song et al. Citation2020), also well-known and successfully used in liquid rockets, diesel, and aircraft engines (Mayer et al. Citation2000), as it has been recently applied in the study of Schmitt (Citation2020), where Large-Eddy Simulations were conducted on the Mascotte test cases operating at supercritical pressure.
A fuel mixture or fluid is considered supercritical when its pressure and temperature values exceed all the critical values of fuel species. If only the temperature exceeds the critical values of the fuel species, the fluid is known as transcritical. On the other hand, if neither temperature nor pressure exceeds the critical values of its species, a subcritical state occurs, as reported by Poling, Prausnitz, and O’Connell (Citation2001), Sako (Citation2002), and Kiran, Debenedetti, and Peters (Citation2012).
According to Liu et al. (Citation2017), supercritical fluids, such as gasoline/ethanol blends, are desirable mediums for chemical processes because of their characteristics. At constant supercritical pressure, temperature increases do not result in phase change but reduce the fluid density. Supercritical fluids have desirable flow properties because they often have lower viscosities and surface tension than liquids (by one order of magnitude). Moreover, supercritical fluids have rapid diffusion rates (typically an order of magnitude higher than liquids). Supercritical combustion occurs without phase change, surface tension, and latent heat (Foster and Miller Citation2010; Schmitt Citation2020). Due to the above qualities, when supercritical fuel is injected into a cylinder, it promotes homogenized mixture distribution. It can effectively decrease engine particle emissions and improve thermal efficiency, as reported by Song et al. (Citation2020).
Concerning the reduction of particulate emission, Boer, Chang, and Shetty (Citation2010) and Boer et al. (Citation2013) studied the emission of pollutants of subcritical and supercritical gasoline GDI injectors at distinct engine operating conditions. Their results indicated that supercritical gasoline presents a lower and soot emission than subcritical gasoline. Further, bio-fuels in the mixture can be used to control emissions. For example, to analyze the alcohol fuels for spark-ignition engines regarding performance, efficiency, and emission effects. In this way, Lee et al. (Citation2009), Szybist et al. (Citation2011) and Turner et al. (Citation2020) explored the beneficial use of methanol or ethanol as an alternative fuel in high concentrations to control emissions from GDIs. Their study’s main finding is that using a high proportion of ethanol E85 reduces emissions relative to GDI with gasoline and E20 by 1–2 orders of magnitude.
Another recent study developed by Yoshimura et al. (Citation2023) investigated the potentiality of utilizing ethanol-based fuel blends to improve the thermal efficiency of spark-ignition (SI) engines and examining its effects under pre-spark heat release (PSHR) conditions. Their findings indicate that non-ethanol fuel has a higher PSHR and shorter combustion duration than ethanol-blended fuel, about 5%, particularly under high engine loads. Also, as revealed by their chemical simulation, the ethanol-blended fuel laminar burning velocity was increased by the partially oxidized reactants caused by PSHR. However, according to the authors, the chemical effects of the ethanol-blended fuel under PSHR conditions were exceeded by the thermodynamic ones, leading to a lower turbulent burning velocity at these conditions.
From this point of view, gasoline and flex-fuel engines and their optimizing thermal efficiency while reducing particle emissions depending on the quality of fuel mixture/air, which are closely linked to fuel breakup, diffusion, atomization, and vaporization of the fuel injecting conditions, as claimed by Yamaguchi et al. (Citation2019). Research has recently focused on enhancing flex-fuel engine performance by developing and applying high and ultra-high-pressure injection (UHPDI) technology. For example, Kaminaga et al. (Citation2019) found that a high-compression ratio SI engine with high-pressure GDI improves the turbulence, promoting a better air-fuel mixture formation and flame speed through a high-speed fuel spray jet. According to the authors, this new combustion concept enables fuel injection delay and SI timing up to near the top dead center (TDC), effectively preventing irregular combustion in a high compression ratio SI engine. Their result indicated that GDI at high pressure maintains the high-load performance and could improve partial load thermal efficiency. It also significantly increases the heat release rate while improving the small-scale turbulent intensity and fuel evaporation. Yamaguchi et al. (Citation2020) states that improving maximum injection pressure and reducing PM emissions have been an increasing trend for new-generation GDI engines up to 100 MPa. Similarly, Yamaguchi et al. (Citation2021) have extensively researched gasoline direct injection engine performance and spray behaviors using ultrahigh injection pressures of up to 1500 Bar, employing various data collection techniques in optical, metal engines, and spray chambers. Their study reveals that high-pressure sprays create more air entrainment, provide better atomization, and accelerate evaporation and mixing. Furthermore, their findings suggest that high-pressure sprays can enable increased flexibility for injection timing and significantly reduce particulate matter (PM) and hydrocarbons (HC) emissions without sacrificing engine performance or increasing other emissions. Also, the impact of fuel consumption improvement is more significant when the injection pressure is 1000 bar.
Other related works, such as those by Li et al. (Citation2018) and Li et al. (Citation2023), have also conducted comparative investigations of impingement sprays from GDI injectors fueled with gasoline and ethanol under injection pressures up to 50 MPa. Their analyses reveal increased injection pressure enhances the vortex scale, improving air/fuel mixing quality. They also identify UHPDI as a potential method to improve air/fuel mixture homogeneity for GDI injectors fueled with ethanol or gasoline/ethanol blends.
When simulating and analyzing high-pressure combustion, it is crucial to account for non-idealities in thermodynamic properties, especially when significant species undergo transcritical and supercritical states. In addition, critical properties such as critical temperature and pressure are essential. presents the critical properties of hydrocarbon and alcohol fluids of interest in this work.
Table 1. Hydrocarbon and alcohol fuels, oxygen, and nitrogen critical properties (Poling, Prausnitz, and O’Connell Citation2001). and
are the critical pressure and temperature.
The experimental ignition delay studies are crucial in validating the chemical kinetics models applied in combustion simulations at high-pressure conditions. However, in most cases, the experimental data fail to reach the supercritical region state. The chemical kinetics models also consider the ideal gas state equation for reacting mixtures at high pressure. Regarding research on supercritical combustion, Roy and Askari (Citation2020) investigated the combustion of ethanol under engine-applicable conditions. Zhang et al. (Citation2021) examined the spray features of diesel surrogates fuel comprised of six components at different pressure injections considering super/trans/subcritical conditions. Furthermore, Harman-Thomas, Hughes, and Pourkashanian (Citation2022) conducted a study about carbon dioxide combustion at supercritical conditions. However, only some studies have considered some real gas equations of state (Eos) (Liang, Li, and Law Citation2019; Mardani and Barani Citation2018). Research of long-chain hydrocarbon fuels, considering real gas, is scarce in the literature. For instance, Kogekar et al. (Citation2018) considers the real gas effects (i.e., a multi-component Redlich-Kwong (R-K) formulation) by comparing high-pressure experimentally measured shock tube (ST) ignition delay data and combustion simulations for n-dodecane/air mixtures. The mechanism used there was developed by Wang et al. (Citation2014) and comprises 100 species and 432 reactions. Their results show that the predicted IDTs are affected by the non-ideal behavior by 50–100 s in the negative temperature coefficient (NTC) region.
The present paper focuses on a new reduced mechanism that contains TRF (aromatic (toluene), n-paraffin (n-heptane), iso-paraffin (iso-octane)), an olefin (diisobutylene, DIB), and ethanol (oxygenated hydrocarbon) for calculating the transcritical/supercritical gasoline surrogate/ethanol mixtures ignition delay times (IDT) using real gas state equations. The final gasoline surrogate/ethanol kinetics mechanism comprised 129 species and 1015 reactions. The simulation used a 0D constant-volume autoignition delay IDT, calculated by Cantera developed version (Goodwin et al. Citation2021). The validation was done using available ST experimental results under supercritical, transcritical, or even subcritical conditions of IDT for different equivalence ratios (), high-pressure range (10–80 atm), and temperatures between 690 and 1250 K. The IDT validation simulations used three EoS: the ideal, the cubic R-K, and the cubic Peng-Robinson (p-R) equation of state. The critical properties of each species in the merged mechanism necessary to obtain the parameters used in the cubic R-K EoS and the cubic p-R EoS were obtained by applying Joback’s Group Method (Poling, Prausnitz, and O’Connell Citation2001; Thomson et al. Citation2008).
Kinetics modeling
Reduction process
The TRF/DIB and the ethanol-reduced mechanisms were obtained using a reducing process comprised of sensitivity analysis (SA) (Rabitz, Kramer, and Dacol Citation1983; Turányi Citation1990) and the directed relation graph with error propagation (DRGEP) (Pepiot-Desjardins and Pitsch Citation2008). All these features are available in Pymars code (1.1.0) from Mestas, Clayton, and Niemeyer (Citation2019).
DRGEP creates smaller mechanisms than the Directed Relation Graph (DRG) by considering the propagation of errors during species removal, as noted in Alviso et al. (Citation2020). So, we applied DRGEP to eliminate multiple species and then evaluated the resulting model by applying temperature sensitivity analysis. To learn more about DRG and DRGEP methodology, please see Pepiot-Desjardins and Pitsch (Citation2008) and Lu and Law (Citation2006a, Citation2006b).
We aimed to produce a skeletal kinetics model containing TRF/DIB as a gasoline surrogate by reducing the Andrae and Kovács (Citation2016) kinetics model, consisting of 159 species and 734 reactions.
We chose the Andrae and Kovács (Citation2016) model because it was validated considering IDT experiments using gasoline surrogates in rapid compression machines, HCCI (Homogeneous charge compression ignition) engines, and ST at high-pressure conditions. This validation was carried out utilizing TRF and TRF/DIB mixtures with octane numbers and H/C ratios similar to commercial gasoline. The TRFDIB exhibited superior agreement with the data, mainly at high pressures (above 30 bar) and temperatures (above 800 K). This range is notably valuable for featuring supercritical and transcritical conditions for the fuel species referenced in .
The reduced ethanol model used in this study is a simplified version of the C1-C3 () mechanism (Burke et al. Citation2014; Kéromnès et al. Citation2013; Metcalfe et al. Citation2013) which originally consisted of 114 species and 1999 reactions. The
model was selected because it was validated using IDT for various mixtures such as (H2+CO)/O2/N2/Ar, small hydrocarbon, and oxygenated hydrocarbon species/air mixtures at pressures ranging of 1 to 70 bar, temperatures from 914 to 2220 K, and equivalence ratios from 0.1 to 4.0. The validation process was critical in accurately describing the combustion features of practical fuels, including IDT, LFS, and emissions. Interestingly, their mechanism was validated for high-pressure and intermediate to high-temperature conditions, crucial for internal combustion engines and gas turbines.
Gasoline surrogate and ethanol models were reduced using ignition delay time (0D constant-volume reactor) at two distinct equivalence ratios ()- lean (=0.50) and stoichiometric (=1.0). The process was carried out within a temperature range of 690 K to 1280 K, with pressures of 10, 30, 50, and 55 (TRF/DIB model) and 10, 30, 50, and 75 atm (ethanol model). The DRGEP reduction process interactively removes species and reactions until a defined threshold error of IDT of 1
is computed for all operating conditions. The DRGEP process targets species OH and CO for TRF/DIB reduction and hydrogen peroxide (
), hydroperoxy radical (
), 1-hydroxyethyl radical
(named “SC2H4OH” in
mechanism), and acetaldehyde (
=
=
) for ethanol reduction due to their highest sensitivity pressure, as reported in Cancino et al. (Citation2010) for the ethanol high-pressure path reaction. The target combustion products for both reduction processes were
,
, and
. The reduced kinetics model of TRF/DIB consists of 121 species and 592 reactions, and the ethanol reduced kinetics model consists of 37 species and 564 reactions.
The IDT simulations of the mixture of gasoline surrogate and air (TRF/DIB/air) and ethanol/air as a function of temperature for stoichiometric combustion conditions ( = 1), pressures of 1, 10, 50, and 100 atm and initial temperatures in the range between 690 and 1250 K are presented in .
Figure 1. Numerical stoichiometric IDT at different pressure points (a) the TRF/DIB reduced mechanism against the original TRF/DIB mechanism Andrae and Kovács (Citation2016) (1, 10, 50, 100 atm) for a mixture of toluene, n-heptane, iso-octane, diisobutylene (surrogate D) (Fikri et al. Citation2008), (b) the ethanol reduced mechanism versus the original one (Burke et al. Citation2014; Kéromnès et al. Citation2013; Metcalfe et al. Citation2013) (1, 10, 100 atm).
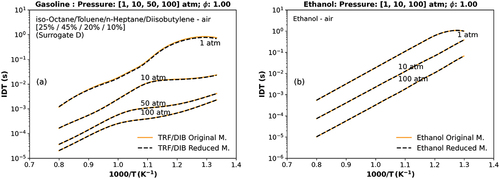
show that the TRF/DIB gasoline surrogate and the ethanol-reduced mechanism results are in good agreement with their original detailed mechanism in all the selected conditions (1, 10, 50, and 100 atm). The highest difference of IDT simulation results between reduced and original mechanisms were 1.0%. Surrogate D, from Fikri et al. (Citation2008), was used in this IDT simulation, and it comprises isooctane, toluene, n-heptane, and diisobutylene proportionately: 25%, 45%, 20%, and 10% by liquid volume.
Merging the reduced mechanisms
A multi-component chemical mechanism was created by combining a reduced kinetics model for TRF/DIB with one for pure ethanol. Despite these fuels having independent combustion mechanisms, they part numerous frequent reactions and species. However, the shared reactions have different Arrhenius and thermochemical parameters, as well as distinct properties of transport. The challenge in merging the models lies in determining the most suitable properties, rates, and coefficients when duplicate species or reactions are present. The reduced ethanol and gasoline surrogate kinetics models shared 29 species and 141 reactions. Following the methodology outlined in Alviso et al. (Citation2020), the reduced mechanisms were merged in two ways to ascertain the most precise intermediate IDT kinetics model.
The first option was to utilize the reduced TRF/DIB model as the foundation mechanism, maintaining vital characteristics such as Arrhenius parameters, thermochemical properties, and transport properties in the intermediate kinetics mechanism. Furthermore, the reduced ethanol kinetics model can be employed as a supplement, incorporating only the necessary species and reactions into the intermediate kinetics mechanism. Alternatively, the second option involves starting with the reduced ethanol model as the foundation mechanism and integrating the TRF/DIB model as a supplement, following the same process.
The merging options generated an intermediary mechanism with 129 species and 1015 elementary reactions. The differences are in the Arrhenius parameters, thermochemical, and transport properties from duplicate reactions and duplicated species. The intermediary model with the reduced TRF/DIB model as a base presented better accuracy with the experimental data. All the mechanisms used in the reduced process are summarized in .
Table 2. Gasoline surrogate (TRF/DIB) and ethanol mechanisms.
OM, Original; RM, Reduced; MM, Merged mechanism; TRF/DIB, toluene reference fuel and diisobutylene; TFRE/DIB, toluene…, ethanol and diisobutylene
Modification of Arrhenius parameters of key reactions using sensitivity analysis
IDT improvement: gasoline surrogates
The original (Andrae and Kovács Citation2016), reduced, and merged models are tested against literature experimental IDT data of single components at high-pressure conditions (50–55 atm), such as iso-octane and toluene from Davidson, Gauthier, and Hanson (Citation2005), and n-heptane from Gauthier, Davidson, and Hanson (Citation2004), as shown in . This was performed to measure better the merged process’s effect in the IDT at high temperature and pressure for each component.
Figure 2. Numerical original, reduced and merged (1st and 2nd) IDT of individual components against experimental data: (a) iso-octane (Davidson, Gauthier, and Hanson Citation2005), (b) n-heptane (Gauthier, Davidson, and Hanson Citation2004), and (c) toluene (Davidson, Gauthier, and Hanson Citation2005). The symbol represents experiments from the literature.
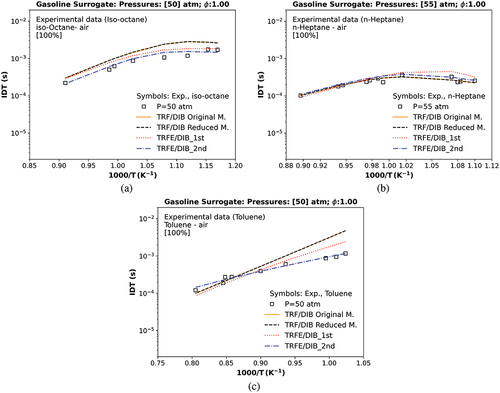
In , the numerical IDT of iso-octane of the original and reduced model presents a significant deviation in temperatures lower than 1000 K concerning the iso-octane experimental data of Davidson, Gauthier, and Hanson (Citation2005).
In , the numerical IDT of toluene of the original (Andrae and Kovács Citation2016) and reduced TRF/DIB model exhibits excellent agreement with experimental data of Davidson, Gauthier, and Hanson (Citation2005) at high temperatures, but a significant over-prediction in temperatures lower than 1000 K. Contrasting with the other single components, the original (Andrae and Kovács Citation2016) and reduced TRF/DIB numerical IDT of n-heptane in show pretty good accuracy with the experimental data of Gauthier, Davidson, and Hanson (Citation2004) at all ranges of temperature experimented. The numerical (TRFE/DIB_1st) IDT over-predicted the experimental data in temperatures lower than 1000 K.
As shown in , although the merged model, called TRFE/DIB_1st, over-predicted/under-predicted the IDT experimental results of each individual component of gasoline surrogates (iso-octane, toluene, n-heptane), it presents better IDT results than the original and reduced TRF/DIB model for toluene and iso-octane but struggles to simulate the n-heptane, especially in low-temperature region. Thus, a sensitivity analysis was conducted to select the main sensitive reactions (key reactions) and to correct these key reactions of every single component of gasoline surrogate (n-heptane, toluene, or iso-octane), as in .
Figure 3. Temperature sensitivity analysis of TRFE/DIB_1st model for single components: (a) iso-octane, (b) n-heptane, and (c) toluene at temperatures equal and lower than 1000 K.
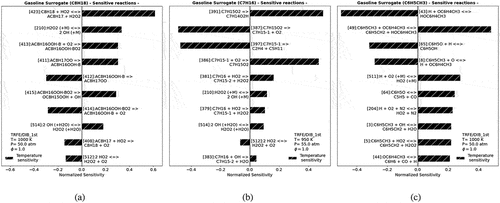
The reaction rate of each selected key reaction of a specific gasoline surrogate was improved by replacing its constant reaction rate (k) with another one from the literature. Increasing or decreasing the reactivity of each key reaction was done by changing its pre-exponential (A), activation energy (Ea), and temperature exponent (b), as summarized in . The k constant improvement methodology is common in the literature, as done by Mittal et al. (Citation2014), and Roy and Askari (Citation2020).
Table 3. Modifying gasoline surrogates (iso-octane, n-heptane, toluene) key reactions constant rate (k).
The resultant merged model, called TRFE/DIB_2nd, present also in , exhibits better agreement with the experimental data of individual components of gasoline surrogates(iso-octane and toluene) as indicated by the lowest boldface root mean square error (RMSE) in and an excellent agreement concerning the n-heptane data in comparison with the original, the reduced and the TRFE/DIB_1st mechanisms exhibited in , also as shown with the RMSE in boldface in .
Table 4. The IDT RMSE for various kinetics models were compared to experimental data of single components of gasoline surrogates (toluene, n-heptane, or iso-octane). The bolded data in each row indicates the model with the lowest values of RMSE compared with the same row experimental data.
IDT improvement: ethanol
The original (Burke et al. Citation2014; Kéromnès et al. Citation2013; Metcalfe et al. Citation2013), reduced, and merged models are tested against experimental IDT of ethanol at high-pressure conditions (40 atm), as shown in . This was performed to measure better the merged process’s effect in the IDT at high temperature and pressure for ethanol.
Figure 4. Temperature sensitivity analysis of TRFE/DIB_1st model for ethanol at a temperature equal to 1100 K (a). Numerical IDT of ethanol of distinct models against experimental data. The symbol represents experiments (Heufer et al. Citation2011).
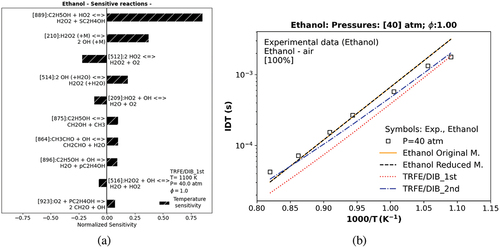
Concerning the TRFE/DIB_1st model, a temperature sensitivity was also conducted to identify main sensitive reactions (key reactions) and to correct these key reactions of ethanol at 40 atm and 1100 K, as shown in .
The reaction rate of each selected key reaction of ethanol was improved by replacing its constant reaction rate (k) with another one from the literature, as summarized in . The merged model TRFE/DIB_2nd in , presents an excellent agreement with ethanol experimental data of Heufer et al. (Citation2011) in comparison with the original, the reduced, and the TRFE/DIB_1st mechanisms, as indicated in the bold in .
Table 5. Modifying ethanol key reactions constant rate (k).
Table 6. RMSE of IDT for various kinetics models compared to ethanol experimental data. Bold data represents the mechanisms with the lowest RMSE values in each row.
The accuracy of the mechanisms in matching ethanol IDT experimental results at 40 atm pressure conditions is determined by the RMSE shown in .
Estimation of critical properties (
) for mixtures
The state of a fuel mixture at ultra-high pressure when inserted into a cylinder is estimated by its critical properties such as temperature, volume, and pressure ( [K],
[
], and
[bar], respectively). According to Green and Southard (Citation2019), the pseudocritical method is the most common way to estimate these properties. This method employs a linear model that accounts for each pure component’s contributions to a given reaction. Still, they may differ substantially from the actual critical properties of mixtures, which is a non-linear phenomenon.
The main difference between critical and pseudocritical properties is that the first is the real critical properties of a system that are determined thermodynamically (by a state equation), and the latter present a nonphysical sense (Takacs Citation2015). According to Poling, Prausnitz, and O’Connell (Citation2001), empirical methods are the more straightforward manner to conjecture critical properties of mixtures. Consequently, when studying single supercritical fuel/air, such as hydrogen/air (Mardani and Barani Citation2018) and methane/air (Liang, Li, and Law Citation2019), researchers use an empirical rules mixture approach correlated with a non-ideal EoS.
Chueh and Prausnitz (Citation1967b) developed an acceptable empirical method to estimate hydrocarbon binary mixtures’ critical temperature and pressure, considering a cubic modified R-K EoS. This empirical method can be expanded for ternary, quaternary, or more complex surrogate fuels, as reported by He et al. (Citation2018) and Lan et al. (Citation2022) for binary and ternary experimental mixtures for performing prediction of the critical properties of n – alkanes, other hydrocarbons, nitrogen, and oxygen- and halogen-containing compounds for fuel mixtures. Thus, in this work, the empirical method of Chueh and Prausnitz (Citation1967b) was utilized to feature the state of the most common gasoline surrogate mixtures (TRF, TRF/DIB, TRF/DIB/ethanol) from the literature.
To classify fuel mixture states as supercritical, transcritical, or subcritical, we used IDT experimental data from the literature () to determine whether the temperature and pressure exceeded the critical properties values.
Table 7. Composition of selected experimental gasoline surrogate and ethanol (by liquid vol.%).
IDT, Ignition delay times; Surr., Surrogate; ST, Shock tube.
The empirical technique of Chueh and Prausnitz (Citation1967a, Citation1967b) was applied considering a cubic modified R-K EoS, as indicated in EquationEquation (1)(1)
(1) .
The values for [K] and
[
] are estimated using the methods proposed by Schick and Prausnitz (Citation1968). The universal gas constant (83.145 [
]) is denoted as R. The hydrocarbon mixtures parameters, such as
and
(binary interaction parameters) are used to obtain
and are delineate in EquationEquations (2)
(2)
(2) –(Equation8
(8)
(8) ).
Each component critical properties in a mixture fuel are expressed by these parameters, including the acentric factor , critical pressure
[bar], critical volume
[
], critical temperature
[K],
[
] (a constant that corrects for the attractive potential of molecules) for component i, and
[
] (a constant that corrects for volume) for component j. Additionally, the species mole fraction of each component in the surrogate mixture is represented by
or
.
is a dimensionless constant that depends on the acentric factor of component j, while
and
are dimensionless constants that depends on the critical properties of component j or i, respectively.
The subscripts or
denote single component j, while
or
represents a single component i.
is a critical property, and
signifies the interaction between i and j. Additionally,
indicates mixture. Typically, the parameter of binary interaction
falls within the range of 0.1 to 0.01. For reference, Chueh and Prausnitz (Citation1967a) has tabulated numerous binary systems.
, critical pressure;
, critical temperature; Surr., Surrogate;
, critical volume;
It is worth noting that ’s first section (Gasoline Surrogate) lists the properties of fuel injection without any air mixture. On the other hand, the second section (Gasoline Surrogate + Air) contains the critical properties of fuel + air mixtures for combustion with a stoichiometric ratio.
Table 8. Predicted critical properties of stoichiometric gasoline surrogate mixtures ( = 1) without and with air concerning the literature mixtures available in .
As cited in the introduction section, the critical properties contribution of each species is not a linear phenomenon, and a supercritical state is achieved when the temperature and pressure surpass critical property values. Concerning the mixtures’ critical properties presented in , the critical temperature of all mixtures is easily achieved in experimental conditions. However, to achieve supercritical combustion, the pressure must be over the selected mixture’s critical value, which is hard to achieve as reported in experimental conditions in . Therefore, the critical pressure property of the mixture is the key to achieving supercritical combustion.
Real equations of state
The ideal equation of state (IES) was used here. However, to better understand the real gas effects on IDT combustion at high pressure, it is necessary to consider an accurate gas EoS in the simulations. This goal is achieved using two cubic multi-component EoS in CANTERA developed version (Goodwin et al. Citation2021): R-K EoS, and p-R Eos. The R-K EoS implementation provides accurate results in various non-ideal states, but its accuracy is known to suffer in the equilibrium of the vapor-liquid region. p-R modified the attractive term definition of R-K EoS to address this issue. The p-R state equation expanded the application range of the cubic equation because it improved the calculation accuracy of liquid density. As a result, it has become one of the most widely used methods for chemical engineering calculations, as highlighted by Green and Perry Green (Citation2007). This study uses a mixture-averaged multi-component definition of the R-K EoS and p-R EoS to predict real gas behavior.
For a pure species,
The Redlich-Kwong state Equation (R-K) is
The Peng-Robinson (P – R) EoS is
Here, the influence of molecular interactions is determined by the specific species Van der Waals repulsive volume correction (b) and the attraction parameter (a). The pressure (P) is measured in Pascals (Pa), while the molar volume (V) is measured in cubic meters per mole (). The gas constant (R) is 8.314 Joules per mole-Kelvin (
), and the absolute temperature (T) is measured in Kelvin (K). The critical pressure (
) and critical temperature (
) are specific to the component of interest and are measured in Pascals (Pa) and Kelvin (K), respectively. The temperature correlation is represented by the symbol alpha (
), while the parameter kappa (
) is generalized with omega (
). The acentric factor (
) is specific to the interest component. It measures its non-sphericity, with values close to zero for nearly spherical molecules (such as the noble gases argon, krypton, and xenon).
For mixtures, EquationEquations (9)(9)
(9) and (Equation11
(11)
(11) ) assume the following form:
where the coefficients ,
, and
are dependent on species i and j, as indicated in EquationEquations (17)
(17)
(17) –(Equation19
(19)
(19) ).
The (a) and (b) in the R-K EoS (EquationEquations 9(9)
(9) and (Equation10
(10)
(10) )) as well as the (a), (b), and (
) in the p-R EoS (EquationEquations 11
(11)
(11) –Equation14
(14)
(14) ), need to be estimated or known for all species present in the chemical kinetics mechanism. However, these parameters rely on the critical properties of the species, such as pressure and temperature, usually accessible for almost all stable species but not for many radicals and intermediate species present in most kinetics mechanisms.
Critical properties estimation for chemical species
This study employs the Joback group contribution method Joback (Citation1984) for estimating the critical pressure (), critical temperature (
), and boiling point (
) of each species contained in the developed reduced mechanism whose values are absent in the literature. The Joback method is a widely used approach in chemistry that considers the fundamental structural properties of each chemical group within an individual species. This method calculates various compound properties by summing up the recurrence in the molecule of each group multiplied by its contribution, which in turn depends on structurally dependent parameters. It assumes that group interactions are imperceptible and work for non-polar and polar species. In Owczarek and Blazej (Citation2003, Citation2004), (
) for various gases were tabulated using several methodologies, and the Joback method was found to limit errors to less than 8.0% for both unbranched and branched hydrocarbons. However, according to Stein and Brown (Citation1994), the Joback method guides to high deviations for small and large molecules and a satisfactory estimation for mid-sized components (Mol Wt of 55 up to 225 g/mol).
In this work, the results of the Joback method present a maximum error of about 9.1% when we compare the experimental critical properties data for stable species from the literature with the estimated one. In addition, for ethanol (Mol Wt = 46 g/mol), iso-octane (Mol. Wt = 114.23 g/mol), and toluene (Mol. Wt = 92.14 g/mol) the temperature of the boiling point () presents a maximum deviation of 9.1%, 2.3%, and 1.10%, respectively.
It is important to point out that the critical properties of most of the intermediate and radical species in the reduced TRFE/DIB mechanism are not available in the literature, so there is no way to calculate an error directly. Nonetheless, they present a molecular weight between 70 and 170 g/mol, which is a good range to use the Joback method for calculating the critical properties.
The temperature of the boiling point () of each species can be evaluated employing the Joback method:
Subsequently, the critical pressure () and temperature (
) are estimated as
Here, denotes the bond-chain type of group, and
denotes the total
groups in the species of interest. It is worth noting that each group type
contributes to the critical temperature (
), boiling-point temperature (
), and critical pressure (
). The term
refers to the number of atoms in the species of interest. All group contributions (
,
,
) are obtained from Poling, Prausnitz, and O’Connell (Citation2001). However, it is important to note that group contribution data for radicals and short-lived species are not readily accessible. Nonetheless, the parameters of unstable species can usually be considered equivalent to those of similar stable species, as reported by Kogekar et al. (Citation2018).
To obtain the values of required for using the Peng Robinson (p-R) EoS, vapor pressure data (
) can be utilized for any fluid (Green Citation2007). Vapor pressure is the equilibrium pressure between coexisting vapor and liquid phases at a certain temperature. The vapor pressure curve is a monotonically increasing function of temperature, ranging from the triple point pressure, its minimum value at the triple point temperature
, to its maximum value (
), at critical temperature
. In this study, the vapor pressure equations of Ambrose-Walton modified by Lee-Kesler Poling, Prausnitz, and O’Connell (Citation2001) were used, as shown in EquationEquation (23)
(23)
(23) .
where ; calculated from auxiliary quantities
=
, and assuming
= 1.01325 [bar]. Then, the vapor pressure in EquationEquation (23)
(23)
(23) is inverted to obtain the (
) from an experimental or estimated vapor pressure single point (e.g., the boiling point (
), for example (
) from Joback (Citation1984) for each species). A comparison between the literature values for n-heptane (Mol. Wt = 100.20 g/mol) (Poling, Prausnitz, and O’Connell Citation2001) and the estimated properties by Joback’s Method is presented in .
Table 9. Literature values for n-heptane properties (Poling, Prausnitz, and O’Connell Citation2001) versus Joback’s heptane estimated properties.
The n-heptane chemical structure is shown in , based on its bond-chain group type () and the total number
of
groups in the n-heptane species, the contribution to the temperature of boiling point (
), the critical pressure (
), and temperature (
) were calculated using EquationEquations (20)
(20)
(20) –(Equation23
(23)
(23) ).
Validation for IDT at transcritical and supercritical conditions using real gas state equations
To compare the developed mechanism (TRFE/DIB_2nd, available in the Supplementary material) at more comprehensive pressure and temperature conditions, various experimental conditions of ST data are collected and summarized in . The critical properties of each mixture summarized before are shown in . It is necessary to highlight that in , only the pure ethanol/air mixture ST experimental data (-) presents a supercritical state because the maximum experimental pressure range (75 atm) in exceeds the estimated critical pressure of the ethanol/air mixture ( 72 atm) in . This value was obtained by the modified cubic R-K equation, mixture rule, and the mixture critical properties methodology of Chueh and Prausnitz (Citation1967b).
shows an excellent agreement between the TRFE/DIB_2nd model and the ethanol ST experimental measurements of IDT at 20 atm (transcritical) and at 75 atm (supercritical) (Heufer and Olivier Citation2010), as indicated in boldface RMSE in considering all three equations of state (ideal, R-K and p-R Eos). Also, shows transcritical combustion at 13 and 40 atm (Heufer et al. Citation2011). At both transcritical and supercritical conditions, a relative deviation around 3% at 40 and 4% at 75 atm is observed between p-R EoS and the ideal EoS as inserted in the last column in . Concerning R-K EoS, the relative deviations were 2% and 3% in relation to the ideal EoS at the same experimental pressure data.
Figure 6. IDT simulations compared with (a) a transcritical data at 20 atm and a supercritical ST data at 75 atm (Heufer and Olivier Citation2010); (b) a transcritical data at 13, and 40 atm (Heufer et al. Citation2011) of a stoichiometric mixture of ethanol, , and
; symbols represent experiments by Heufer and Olivier (Citation2010); Heufer et al. (Citation2011); solid and dashed lines are the TRFE/DIB_2nd mechanism, respectively, using the ideal (IES) and the real cubic gas R-K and the p-R EoS.
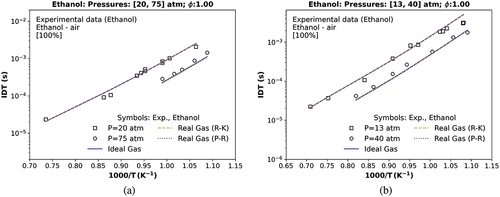
Table 10. IDT RMSE of the TRFE/DIB_2nd model against quinary, quaternary, and ternary mixtures of gasoline surrogates and ethanol experimental data. In addition, the relative deviations (%) of IDT simulations were analyzed between the same kinetics model that used distinct equations of states (redlich-kwong and peng-robinson), compared to the IDT simulations that relied on the ideal equation of state.
Figure 7. IDT simulations compared with (a) a transcritical data at 25 atm and a transcritical data at 55 atm testing a TRF, , and
stoichiometric mixture (Gauthier, Davidson, and Hanson Citation2004); (b) a transcritical data at 10 and 30 atm using TRF/ethanol (Cancino et al. Citation2009). Symbols represent experiments by Cancino et al. (Citation2009); Gauthier, Davidson, and Hanson (Citation2004); solid and dashed lines are the TRFE/DIB_2nd mechanism, respectively, using the ideal (IES) and the real cubic gas RK and the p-R EoS.
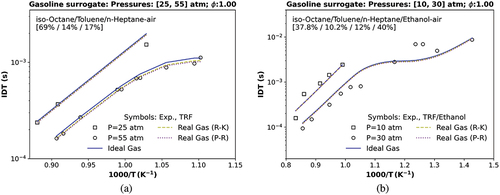
Figure 8. IDT simulations compared with (a) a transcritical ST data at 10, 30, and 50 atm utilizing a stoichiometric mixture of TRF/DIB, , and
(Fikri et al. Citation2008); (b) a transcritical data at 10 and 30 atm with a stoichiometric mixture of TRF/DIB and ethanol (Cancino et al. Citation2011); c) a numerical transcritical IDT condition at 80 and a numerical supercritical IDT condition at 150 atm. Symbols represent experiments by (Cancino et al. Citation2011; Fikri et al. Citation2008); solid and dashed lines are the TRFE/DIB_2nd mechanism, respectively, using the ideal (IES), the real cubic gas R-K and the p-R EoS.
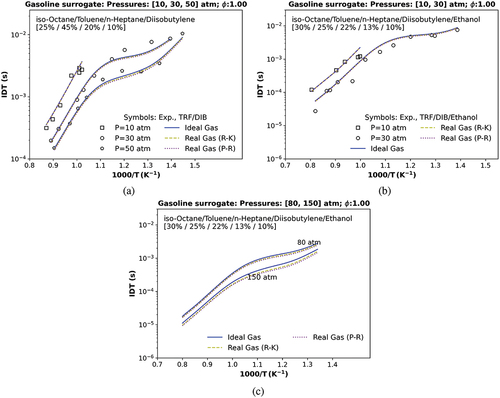
The TRFE/DIB_2nd mechanism was also compared with the remaining ST experimental data, exhibited in , at different pressure conditions using distinct stoichiometric gasoline surrogates mixtures or gasoline surrogates/ethanol blends. Unfortunately, none of these data experimental pressure conditions in exceeds their respective mixture critical pressure calculated in , which means that all those ST experimental gasoline surrogate/air combustion occurs at a transcritical state because only their experimental temperature overpass the critical conditions.
In , a comparison between the transcritical numerical IDT with all used EoS and the experimental data of Gauthier, Davidson, and Hanson (Citation2004) at 55 atm using a ternary surrogate mixture (iso-octane/toluene/n-heptane, 69%/14%/17% by liquid vol.), reveals that TRFE/DIB_2nd kinetics mechanism is slightly closer to the experimental measurement in this condition, although it presents a 6.00% of deviation between the ideal (IES) and the real gas R-K Eos and 8.38% concerning p-R EoS as shown in the two last column of . The transcritical numerical results at 25 atm present some deviation from experimental measurement near 1000 K. However, the cubic equations of (R-K) and (p-R) do not strongly diverge from ideal gas simulation IDT results at these temperatures and reduced pressure conditions, as expected, because they are far from the NTC region.
shows that the TRFE/DIB_2nd mechanism also exhibits an excellent agreement when comparing its numerical transcritical IDT of a quaternary mixture (iso-octane/toluene/n-heptane/ethanol, 37.8%/10.2%/12%/40% by liquid vol.) and the experimental data of Cancino et al. (Citation2009) at 10 atm with RMSE around 1.6e-04 s and 30 atm with the lowest RMSE around 1.7e-03 s concerning all three equation of state (ideal, R-K and p-R EoS), as shown in .
shows the numerical IDT of a quaternary mixture (iso-octane/toluene/n-heptane/diisobutylene, 25%/45%/20%10% by liquid vol.) against the experimental data from Fikri et al. (Citation2008), respectively, at 10, 30, and 50 atm (transcritical). At all pressure conditions, a slight deviation is observed at low temperatures for this gasoline surrogate (TRF/DIB) concerning the experimental data, whereas an improved agreement can be observed at high temperatures. |The RMSE of all three EoS was quite similar, with values between and
s. In , the same gasoline surrogate compounds are blended with ethanol in Cancino et al. (Citation2011) in a different proportion, and the mixture of TRF/DIB/ethanol/air achieves supercritical pressure values over 85 atm when the experimental pressure was performed at only 30 atm in . Thus, at 30 atm, the simulations using the surrogate TRF/DIB/ethanol/air are not considered at supercritical conditions.
In , to observe the effect of the cubic EoS (R-K and p-R), two more IDT numerical simulations were performed without considering experimental data, one at transcritical conditions (80 atm) and another at supercritical conditions (150 atm). It is possible to notice that an increase in pressure (80 to 150 atm) strongly promotes a deviation of simulations using the ideal gas (IES) against the real cubic gas (R-K) and the (p-R) EoS (17.60% and 24.09%, respectively), as indicated in the last two columns of . This shows that the most significant deviation occurs against the p-R EoS. It is important to comment that , at 10 atm, do not present a NTC because the NTC usually occurs at low temperatures (500 K < T < 900 K), which differs from the numerical data at 10 atm that shows a temperature range between 920 and 1240 K.
estimates the agreement of the mechanisms with the gasoline surrogate and the ethanol IDT measurements under different pressure ranges based on the RMSE. The relative deviations (%) of IDT simulations for the same kinetics models, using distinct equations of states (Redlich-Kwong and Peng-Robinson), compared to the same conditions simulations using ideal EoS are also presented in the last two columns of .
Rel. D., Relative deviation about the IDT simulations adopting the ideal EoS;
Conclusions
This paper presents a reduced model for simulating the combustion of TRF/DIB/ethanol blends. The model consists of 129 species and 1015 reactions, including ethanol, diisobutylene, n-heptane, iso-octane, and toluene.
To develop this kinetics model, a TRF/DIB reduced mechanism and an ethanol-reduced model were merged to simulate supercritical/transcritical TRF/DIB/ethanol blends. The reduced model was validated for ethanol at supercritical conditions by comparing it with available experimental data of HPST, and it was validated for TRF/DIB/ethanol blends at transcritical conditions using shock tube experimental data. Both reduced mechanisms were obtained, considering high-pressure conditions, using a combination of sensitivity analysis and directed relation graph with error propagation method.
The developed mechanism was simulated using two cubic multi-component equations of state, the Peng-Robinson (p-R) and Redlich-Kwong (R-K), to predict real gas behavior effects on IDT high-pressure combustion. Empirical methods were used to determine the influence of molecular interaction parameters required for both cubic equations, such as the specific species repulsive volume correction (b), the attraction parameter (a), and the non-sphericity (acentric factor ()) of each component molecule using the critical properties of each species in the new mechanism. However, not all the critical properties of all species mechanisms are available in the literature. Thus, estimating critical properties was obtained by applying a sequential process using Joback’s Group Method and the Ambrose-Walton vapor pressure equation, exhibiting deviation a maximum within 8% compared to critical literature properties. For some radicals and short-lived species not readily accessible, their critical properties can be estimated by considering that they are equivalent to those of similar stable species.
We estimated the critical properties of the most prevalent fuel/air experimental IDT compositions through empirical methods complementary with an adjusted cubic Redlich-Kwong state equation. These data were gathered from the literature and utilized for IDT validations. Based on these estimated properties findings, we can categorize the physical conditions of combustion as supercritical, transcritical, or subcritical.
The developed mechanism was validated using experimental data for several mixtures of fuel species (TRF/DIB/ethanol, TRF/DIB, TRF, and PRF) and pure ethanol. The study’s operating conditions, including non-idealities, resulted in a decrease of approximately 5.91% in predicted IDT when comparing ideal gas against R-K EoS and an 8.01% decrease when comparing against (p-R) EoS simulations at 55 atm. The non-ideal effects are insignificant at 50 atm for the fuel mixtures studied here. However, as the pressure increases, these effects become increasingly significant. For instance, at 150 atm, the difference between the predicted IDT of ideal and real gases (R-K EoS and p-R EoS) was almost 17.61% and 24% in the NTC region.
In conclusion, the developed reduced mechanism extensively reproduces the results of IDT at transcritical for TRF/DIB/ethanol blends and at supercritical conditions when tested against the available experimental data for ethanol.
Supplemental Material
Download Zip (5 MB)Disclosure statement
No potential conflict of interest was reported by the author(s).
Supplementary material
Supplemental data for this article can be accessed online at https://doi.org/10.1080/00102202.2024.2347615
Additional information
Funding
References
- Alviso, D., M. Weyl Costa, L. Backer, P. Pepiot, N. Darabiha, and R. Gonçalves dos Santos. 2020. Chemical kinetic mechanism for diesel/biodiesel/ethanol surrogates using n-decane/methyl-decanoate/ethanol blends. J. Braz. Soc. Mech. Sci. Eng. 42 (2):1–14. doi:10.1007/s40430-020-2186-9.
- Andrae, J. C. 2008. Development of a detailed kinetic model for gasoline surrogate fuels. Fuel 87 (10–11):2013–22. doi:10.1016/j.fuel.2007.09.010.
- Andrae, J., and T. Kovács. 2016. Evaluation of adding an olefin to mixtures of primary reference fuels and toluene to model the oxidation of a fully blended gasoline. Energy Fuels 30 (9):7721–30. doi:10.1021/acs.energyfuels.6b01193.
- Binder, A., R. Ecker, A. Glaser, and K. Müller. 2015. Gasoline direct injection. In Gasoline Engine Management, ed. R. Konrad, 110–21. Wiesbaden: Springer Vieweg.
- Boer, C., G. Bonar, S. Sasaki, and S. Shetty (2013). Application of supercritical gasoline injection to a direct injection spark ignition engine for particulate reduction. Technical report, SAE Technical Paper.
- Boer, C., J. Chang, and S. Shetty (2010). Transonic combustion-a novel injection-ignition system for improved gasoline engine efficiency. Technical report, SAE Technical Paper.
- Bokor, C., B. Rohani, C. Humphries, D. Morrey, and F. Bonatesta. 2021. Investigating the impact of gasoline composition on pn in gdi engines using an improved measurement method. Int. J. Engine Res. 22 (11):3391–406. doi:10.1177/1468087420970374.
- Burke, S. M., W. Metcalfe, O. Herbinet, F. Battin-Leclerc, F. M. Haas, J. Santner, F. L. Dryer, and H. J. Curran. 2014. An experimental and modeling study of propene oxidation. part 1: Speciation measurements in jet-stirred and flow reactors. Combust. Flame 161 (11):2765–84. doi:10.1016/j.combustflame.2014.05.010.
- Cancino, L., M. Fikri, A. Oliveira, and C. Schulz. 2009. Autoignition of gasoline surrogate mixtures at intermediate temperatures and high pressures: Experimental and numerical approaches. Proc. Combust. Inst. 32 (1):501–08. doi:10.1016/j.proci.2008.06.180.
- Cancino, L., M. Fikri, A. Oliveira, and C. Schulz. 2010. Measurement and chemical kinetics modeling of shock-induced ignition of ethanol- air mixtures. Energy Fuels 24 (5):2830–40. doi:10.1021/ef100076w.
- Cancino, L., M. Fikri, A. Oliveira, and C. Schulz. 2011. Ignition delay times of ethanol-containing multi-component gasoline surrogates: Shock-tube experiments and detailed modeling. Fuel 90 (3):1238–44. doi:10.1016/j.fuel.2010.11.003.
- Chueh, P., and J. Prausnitz. 1967a. Vapor-liquid equilibria at high pressures: Calculation of critical temperatures, volumes, and pressures of nonpolar mixtures. AichE J. 13 (6):1107–13. doi:10.1002/aic.690130613.
- Chueh, P., and J. Prausnitz. 1967b. Vapor-liquid equilibria at high pressures: Calculation of partial molar volumes in nonpolar liquid mixtures. AichE J. 13 (6):1099–107. doi:10.1002/aic.690130612.
- Davidson, D., B. Gauthier, and R. Hanson. 2005. Shock tube ignition measurements of iso-octane/air and toluene/air at high pressures. Proc. Combust. Inst. 30 (1):1175–82. doi:10.1016/j.proci.2004.08.004.
- Elfasakhany, A. 2014. Experimental study on emissions and performance of an internal combustion engine fueled with gasoline and gasoline/n-butanol blends. Energy Convers. Manage. 88:277–83. doi:10.1016/j.enconman.2014.08.031.
- Fikri, M., J. Herzler, R. Starke, C. Schulz, P. Roth, and G. Kalghatgi. 2008. Autoignition of gasoline surrogates mixtures at intermediate temperatures and high pressures. Combust. Flame 152 (1–2):276–81. doi:10.1016/j.combustflame.2007.07.010.
- Foster, J., and R. S. Miller. 2010. Fundamentals of high pressure combustion. In High Pressure Processes in Chemical Engineering, ed. M. Lackner, 53–72. ProcessEng Engineering GmbH.
- Gauthier, B., D. F. Davidson, and R. K. Hanson. 2004. Shock tube determination of ignition delay times in full-blend and surrogate fuel mixtures. Combust. Flame 139 (4):300–11. doi:10.1016/j.combustflame.2004.08.015.
- Goodwin, D. G., R. L. Speth, H. K. Moffat, and B. W. Weber. 2021. Cantera: An object-oriented software toolkit for chemical kinetics, thermodynamics, and transport processes. Version 3.0.0. doi:10.5281/zenodo.8137090.
- Green, D. 2007. Perry’s chemical engineer’s handbook 8/e section 2 physical & chem data (pod). New York: McGraw-Hill Education.
- Green, D. W., and M. Z. Southard. 2019. Perry’s chemical engineers’ handbook. New York: McGraw-Hill Education.
- Harman-Thomas, J. M., K. J. Hughes, and M. Pourkashanian. 2022. The development of a chemical kinetic mechanism for combustion in supercritical carbon dioxide. Energy 255:124490. doi:10.1016/j.energy.2022.124490.
- Heufer, K., and H. Olivier. 2010. Determination of ignition delay times of different hydrocarbons in a new high pressure shock tube. Shock. Waves 20 (4):307–16. doi:10.1007/s00193-010-0262-2.
- Heufer, K. A., S. M. Sarathy, H. J. Curran, A. C. Davis, C. K. Westbrook, and W. J. Pitz. 2012. Detailed kinetic modeling study of n-pentanol oxidation. Energy Fuels 26 (11):6678–85. doi:10.1021/ef3012596.
- Heufer, K., Y. Uygun, H. Olivier, S. Vranckx, C. Lee, and R. Fernandes (2011). Experimental study of the high-pressure ignition of alcohol based biofuels. In Proc. European Combust Meeting, Cardiff, U.K., June 29–July 1, 2011.
- He, M., C. Wang, J. Chen, and X. Liu. 2018. Prediction of the critical properties of mixtures based on group contribution theory. J. Mol. Liq. 271:313–18. doi:10.1016/j.molliq.2018.08.048.
- Iwamoto, Y., K. Noma, O. Nakayama, T. Yamauchi, and H. Ando. 1997. Development of gasoline direct injection engine. SAE Trans. 106:777–93.
- Joback, K. G. (1984). A unified approach to physical property estimation using multivariate statistical techniques. Ph. D. thesis, Massachusetts Institute of Technology.
- Kaminaga, T., K. Yamaguchi, S. Ratnak, J. Kusaka, T. Youso, T. Fujikawa, and M. Yamakawa (2019, September). A study on combustion characteristics of a high compression ratio si engine with high pressure gasoline injection. SAE Technical Papers 2019-September (September). SAE 14th International Conference on Engines and Vehicles, Capri, Napoli (Italy), ICE; 15-09-2019 Through 19-09-2019.
- Kéromnès, A., W. K. Metcalfe, K. A. Heufer, N. Donohoe, A. K. Das, C.-J. Sung, J. Herzler, C. Naumann, P. Griebel, O. Mathieu, et al. 2013. An experimental and detailed chemical kinetic modeling study of hydrogen and syngas mixture oxidation at elevated pressures. Combust. Flame 160(6):995–1011. doi:10.1016/j.combustflame.2013.01.001.
- Kiran, E., P. G. Debenedetti, and C. J. Peters. 2012. Supercritical fluids: Fundamentals and applications, Vol. 366. Springer Dordrecht: Springer Science & Business Media.
- Kogekar, G., C. Karakaya, G. J. Liskovich, M. A. Oehlschlaeger, S. C. DeCaluwe, and R. J. Kee. 2018. Impact of non-ideal behavior on ignition delay and chemical kinetics in high-pressure shock tube reactors. Combust. Flame 189:1–11. doi:10.1016/j.combustflame.2017.10.014.
- Lan, T., Y. Wang, R. Ali, H. Liu, X. Liu, and M. He. 2022. Prediction and measurement of critical properties of gasoline surrogate fuels and biofuels. Fuel Process. Technol. 228:107156. doi:10.1016/j.fuproc.2021.107156.
- Lee, J., R. Patel, A. Schonborn, N. Ladommatos, and C. Bae. 2009. Effect of biofuels on nanoparticle emissions from spark-and compression-ignited single-cylinder engines with same exhaust displacement volume. Energy Fuels 23 (9):4363–69. doi:10.1021/ef9004708.
- Liang, W., W. Li, and C. K. Law. 2019. Laminar flame propagation in supercritical hydrogen/air and methane/air mixtures. Proc. Combust. Inst. 37 (2):1733–39. doi:10.1016/j.proci.2018.06.070.
- Li, X., D. Li, P. Dimitriou, T. Ajmal, A. Aitouche, R. Mobasheri, O. Rybdylova, Y. Pei, and Z. Peng. 2023. Comparative investigation on macroscopic and microscopic characteristics of impingement spray of gasoline and ethanol from a gdi injector under injection pressure up to 50 mpa. Energy Rep. 9:1910–18. doi:10.1016/j.egyr.2023.01.024.
- Li, X., Y. Qiang Pei, J. Qin, D. Zhang, K. Wang, and B. Xu. 2018. Effect of ultra-high injection pressure up to 50 mpa on macroscopic spray characteristics of a multi-hole gasoline direct injection injector fueled with ethanol. Proc. Inst. Mech. Eng. Part D: J. Automob. Eng. 232 (8):1092–104. doi:10.1177/0954407017726720.
- Liu, Y., M. Jia, M. Xie, and B. Pang. 2013. Improvement on a skeletal chemical kinetic model of iso-octane for internal combustion engine by using a practical methodology. Fuel 103:884–91. doi:10.1016/j.fuel.2012.07.046.
- Liu, Y., Y. Pei, Z. Peng, J. Qin, Y. Zhang, Y. Ren, and M. Zhang. 2017. Spray development and droplet characteristics of high temperature single-hole gasoline spray. Fuel 191:97–105. doi:10.1016/j.fuel.2016.11.068.
- Li, S., G. Williams, and Y. Guo. 2016. Health benefits from improved outdoor air quality and intervention in china. Environ. Pollut. 214:17–25. doi:10.1016/j.envpol.2016.03.066.
- Lu, T., and C. K. Law. 2006a. Linear time reduction of large kinetic mechanisms with directed relation graph: N-heptane and iso-octane. Combust. Flame 144 (1–2):24–36. doi:10.1016/j.combustflame.2005.02.015.
- Lu, T., and C. K. Law. 2006b. On the applicability of directed relation graphs to the reduction of reaction mechanisms. Combust. Flame 146 (3):472–83. doi:10.1016/j.combustflame.2006.04.017.
- Mardani, A., and E. Barani. 2018. Numerical investigation of supercritical combustion of h2–o2. Energy Fuels 32 (3):3851–68. doi:10.1021/acs.energyfuels.7b03025.
- Mayer, W., A. Schik, M. Schaf Fler, and H. Tamura. 2000. Injection and mixing processes in high-pressure liquid oxygen/gaseous hydrogen rocket combustors. J. Propul. Power 16 (5):823–28. doi:10.2514/2.5647.
- Mestas, P., P. Clayton, and K. Niemeyer. 2019. Pymars: Automatically reducing chemical kinetic models in python. J. Open Source Softw. 4 (41):1543. doi:10.21105/joss.01543.
- Metcalfe, W. K., S. M. Burke, S. S. Ahmed, and H. J. Curran. 2013. A hierarchical and comparative kinetic modeling study of c1–c2 hydrocarbon and oxygenated fuels. Int. J. Chem. Kinet. 45 (10):638–75. doi:10.1002/kin.20802.
- Mittal, G., S. M. Burke, V. A. Davies, B. Parajuli, W. K. Metcalfe, and H. J. Curran. 2014. Autoignition of ethanol in a rapid compression machine. Combust. Flame 161 (5):1164–71. doi:10.1016/j.combustflame.2013.11.005.
- Owczarek, I., and K. Blazej. 2003. Recommended critical temperatures. part i. aliphatic hydrocarbons. J. Phys. Chem. Ref. Data 32 (4):1411–27. doi:10.1063/1.1556431.
- Owczarek, I., and K. Blazej. 2004. Recommended critical temperatures. part ii. aromatic and cyclic hydrocarbons. J. Phys. Chem. Ref. Data 33 (2):541–48. doi:10.1063/1.1647147.
- Pepiot-Desjardins, P., and H. Pitsch. 2008. An efficient error-propagation-based reduction method for large chemical kinetic mechanisms. Combust. Flame 154 (1–2):67–81. doi:10.1016/j.combustflame.2007.10.020.
- Piock, W., G. Hoffmann, A. Berndorfer, P. Salemi, and B. Fusshoeller. 2011. Strategies towards meeting future particulate matter emission requirements in homogeneous gasoline direct injection engines. SAE Int. J. Engines 4 (1):1455–68. doi:10.4271/2011-01-1212.
- Poling, B., J. Prausnitz, and J. O’Connell. 2001. The properties of gases and liquids. 5th ed. New York: McGraw-Hill.
- Rabitz, H., M. Kramer, and D. Dacol. 1983. Sensitivity analysis in chemical kinetics. Annu. Rev. Phys. Chem. 34 (1):419–61. doi:10.1146/annurev.pc.34.100183.002223.
- Roy, S., and O. Askari. 2020. A new detailed ethanol kinetic mechanism at engine-relevant conditions. Energy Fuels 34 (3):3691–708. doi:10.1021/acs.energyfuels.9b03314.
- Sako, T. 2002. Supercritical fluids: Molecular interactions, physical properties, and new applications. Springer Berlin Heidelberg: Springer Science & Business Media.
- Schick, L., and J. Prausnitz. 1968. On the correlation of critical properties of mixtures. AichE J. 14 (4):673–673. doi:10.1002/aic.690140432.
- Schmitt, T. 2020. Large-eddy simulations of the mascotte test cases operating at supercritical pressure. Flow Turbul. Combust. 105 (1):159–89. doi:10.1007/s10494-019-00096-y.
- Song, Y., Z. Zheng, T. Peng, Z. Yang, W. Xiong, and Y. Pei. 2020. Numerical investigation of the combustion characteristics of an internal combustion engine with subcritical and supercritical fuel. Appl. Sci. 10 (3):862. doi:10.3390/app10030862.
- Stein, S. E., and R. L. Brown. 1994. Estimation of normal boiling points from group contributions. J. Chem. Inf. Comp. Sci. 34 (3):581–87. doi:10.1021/ci00019a016.
- Szybist, J. P., A. D. Youngquist, T. L. Barone, J. M. Storey, W. R. Moore, M. Foster, and K. Confer. 2011. Ethanol blends and engine operating strategy effects on light-duty spark-ignition engine particle emissions. Energy Fuels 25 (11):4977–85. doi:10.1021/ef201127y.
- Takacs, G. 2015. Chapter 2 - a review of production engineering fundamentals. In Sucker-rod pumping handbook, ed. G. Takacs, 13–56. Boston: Gulf Professional Publishing.
- Thomson, G. H., D. G. Friend, R. L. Rowley, W. V. Wilding, and B. E. Poling. 2008. Physical and chemical data section 2. Perry’s Chem Eng Handbook. McGraw-Hill, New York. 1:2–48.
- Turányi, T. 1990. Sensitivity analysis of complex kinetic systems. tools and applications. J Math Chem 5 (3):203–48. doi:10.1007/BF01166355.
- Turner, J. W. G., A. G. J. Lewis, S. Akehurst, C. J. Brace, S. Verhelst, J. Vancoillie, L. Sileghem, F. C. P. Leach, and P. P. Edwards. 2020. Alcohol fuels for spark-ignition engines: Performance, efficiency, and emission effects at mid to high blend rates for ternary mixtures. Energies 13 (23):6390. doi:10.3390/en13236390.
- Wang, H., Y. Ra, M. Jia, and R. D. Reitz. 2014. Development of a reduced n-dodecane-pah mechanism and its application for n-dodecane soot predictions. Fuel 136:25–36. doi:10.1016/j.fuel.2014.07.028.
- Yamaguchi, A., L. Koopmans, A. Helmantel, J. Dillner, and P. Dahlander. 2020. Air motion induced by ultra-high injection pressure sprays for gasoline direct injection engines. SAE Int. J. Fuels Lubr. 13 (3):223–36. doi:10.4271/04-13-03-0014.
- Yamaguchi, A., L. Koopmans, A. Helmantel, J. Dillner, and P. Dahlander. 2021. Spray behaviors and gasoline direct injection engine performance using ultrahigh injection pressures up to 1500 bar. SAE Int. J. Engines 15 (3–15–01–0007):167–83. doi:10.4271/03-15-01-0007.
- Yamaguchi, A., L. Koopmans, A. Helmantel, F. P. Karrholm, and P. Dahlander (2019). Spray characterization of gasoline direct injection sprays under fuel injection pressures up to 150 mpa with different nozzle geometries. Technical report, SAE Technical Paper.
- Yoshimura, K., K. Isobe, M. Kawashima, K. Yamaguchi, R. Sok, S. Tokuhara, and J. Kusaka. 2023. Effects of pre-spark heat release of ethanol-blended gasoline surrogate fuels on engine combustion behavior. SAE Int. J. Fuels Lubr. 17 (1). Publisher Copyright: 2023 SAE International. All rights reserved doi:10.4271/04-17-01-0003.
- Zhang, Y., Q. Wang, R. Yang, Y. Yan, F. Jiahong, and Z. Liu. 2022 03. Numerical investigation of the effect of injection timing on the in-cylinder activity of a gasoline direct injection engine. Adv. Mech. Eng. 14 (3):168781322210828. doi:10.1177/16878132221082873.
- Zhang, Q., J. Xia, Z. He, J. Wang, R. Liu, L. Zheng, Y. Qian, D. Ju, and X. Lu. 2021. Experimental study on spray characteristics of six-component diesel surrogate fuel under sub/trans/supercritical conditions with different injection pressures. Energy 218:119474. doi:10.1016/j.energy.2020.119474.
- Zong, N., H. Meng, S.-Y. Hsieh, and V. Yang. 2004. A numerical study of cryogenic fluid injection and mixing under supercritical conditions. Phys. Fluids 16 (12):4248–61. doi:10.1063/1.1795011.