Abstract
Background: Acute Respiratory Distress syndrome (ARDS) is a clinical syndrome of noncardiac pulmonary edema and inflammation leading to acute respiratory failure. We used the oleic acid infusion pig model of ARDS resembling human disease to explore cytokine changes in white blood cells (WBC) and plasma proteins, comparing baseline to ARDS values. Methods: Nineteen juvenile female swine were included in the study. ARDS defined by a PaO2/FiO2 ratio < 300 was induced by continuous oleic acid infusion. Arterial blood was drawn before and during oleic acid infusion, and when ARDS was established. Cytokine expression in WBC was analyzed by RT-qPCR and plasma protein expression by ELISA. Results: The median concentration of IFN-γ mRNA was estimated to be 59% (p = 0.006) and of IL-6 to be 44.4% (p = 0.003) of the baseline amount. No significant changes were detected for TNF-α, IL-17, and IL-10 mRNA expression. In contrast, the concentrations of plasma IFN-γ and IL-6 were significantly higher (p = 0.004 and p = 0.048 resp.), and TNF-α was significantly lower (p = 0.006) at ARDS compared to baseline. Conclusions: The change of proinflammatory cytokines IFN-γ and IL-6 expression is different comparing mRNA and plasma proteins at oleic acid-induced ARDS compared to baseline. The migration of cells to the lung may be the cause for this discrepancy.
1. Introduction
Acute Respiratory Distress Syndrome (ARDS) is a life-threatening condition characterized by rapid onset and acute hypoxemic respiratory failure associated with substantial morbidity and mortality.Citation1,Citation2 The severity of ARDS is defined based on the degree of hypoxemia assessed by the Horowitz quotient (PaO2:FiO2 ratio) or oxygenation index.Citation3,Citation4 Oleic acid infusion is often used to investigate the development of ARDS caused by pulmonary fat embolization that is attributed to long bone fracture, burns, acute pancreatitis, and heat exposure.Citation5,Citation6 In addition, oleic acid is the most common and abundant fatty acid transported in blood bound to albumin or as lipoproteins to prevent cytotoxicity. Released by injury, free fatty acids including oleic acid change membrane structure and cause vascular leakage. Six hours after intravenous oleic acid injection in mice or rats, the serum TNF-α and IL-6 levels are significantly increased.Citation7,Citation8 Thus, intravenous oleic acid infusion disrupting the integrity of the alveolar-capillary barrier is a great model for ARDS, causing severe alveolar degeneration, interstitial edema, inflammatory cell infiltration, and vascular congestion secondary to activated inflammatory pathways.Citation1,Citation9–14 The swine model of oleic acid infusion is often used because their lung resemble human lungs physiologically and immunologically.Citation14–18
However, cytokine expression during ARDS varies with study design and models, and no study reports early changes of inflammatory markers in blood before, at the time of clinical insult, and when ARDS criteria is met. This study aims to identify cytokine mRNA expression at the initiation of oleic acid infusion and at the time of achieving ARDS defined by a PaO2/FiO2 ratio <300 in a swine model of oleic acid infusion and compare it to the protein concentration at both time points.
2. Materials and methods
2.1. Study design
This observational study was approved by the University of Wisconsin Institutional Animal Care and Use Committee (IACUC) in accordance with the guidelines in the US Department of Health and Human Services and the National Institutes for Health. Female pigs of 20 ± 3 kg, corresponding to a human age of 6–7 years of age, were sedated with 4–7 mg/kg of Telazol and 2 mg/kg of Xylazine.Citation19 Intubation and mechanical ventilation with a tidal volume (Vt) of 8–10 ml/kg was initiated. Animals were kept under general anesthesia with 2% isoflurane, and ventilation was performed at a fraction of inspired oxygen (FiO2) of 0.50 and Positive End Expiratory Pressure (PEEP) of 7–8 cmH2O. The respiratory rate was initially set at 20 breaths per minute and then adjusted to maintain PaCO2 between 35–45 mmHg until induction of ARDS. An intravenous catheter was placed in each ear for drug delivery. Two additional central catheters were placed each in the right femoral vein and right femoral artery for pressure measurement and oleic acid infusion. After stabilization, approximately 4 mL blood samples were obtained through a femoral artery catheter at baseline immediately before oleic acid infusion. Continuous oleic acid (Methyl Oleate, TCI America, Portland, OR) infusion was initiated at a rate of 0.05 mL/kg and titrated up to 0.6 mL/kg every 15 min until ARDS was achieved. This results in an approximate total of 5.25 mL oleic acid infused after 90 min.
Arterial blood samples were obtained immediately following intubation, prior to initiating oleic acid, then every 15 min, while infusing oleic acid, to monitor acid-base and gas exchange, including PaO2. ARDS was defined per Berlin criteria including presence of acute hypoxemic respiratory failure defined by P/F ratio < 300 mmHg, bilateral opacities on chest x-ray, a minimum of 5 cm H2O of positive end-expiratory pressure (PEEP) and chest x-ray imagingCitation3). In addition, and given that his was a pediatric model, we insured that all animals met the PALICC definition of ARDS including oxygenation index (OI).Citation20 Once ARDS was achieved, a final blood sample was drawn through the femoral artery catheter and oleic acid infusion was stopped at that time. Arterial blood samples were analyzed using a pHOx Ultra blood gas analyzer (Nova Biomedical, Waltham, MA).
2.2. Sample preparation
Blood samples were taken prior to oleic-acid injection and after ARDS was apparent, as described earlier. Blood was collected in EDTA blood collection tubes (BD Vacutainer; Becton Dickinson, NJ), centrifuged, and plasma was frozen at −80 °C. Red blood cells were lysed with ammonium chloride solution (155 mM NH4Cl, 10 mM KHCO3, 0.1 mM EDTA, pH 7.3) and total RNA was extracted from white blood cells (WBC) using Spin column extraction (SV Total RNA Isolation (Promega, Madison, WI). Briefly, the cell pellet was lysed in RNA lysis buffer, loaded onto spin columns, and purified by a series of centrifugations. Total bound RNA was eluted with H2O. Purity and concentration were determined using NanoDrop Spectrophotometer (Thermo Fisher Scientific; Waltham, MA) at wavelengths 230, 260, and 280 nm. cDNA was synthesized by reverse transcription (GoScript™ Reverse Transcription System by Promega, Madison, WI). cDNA purity and concentration were determined and samples were used for PCR or kept frozen at −80 °C.
2.3. qPCR
Primers were selected using Primer-BLAST from the National Library of Medicine. Swine (Sus Scrofa) gene sequences used for primer design were taken from the National Center for Biotechnology Information and CDS was used for primer design. Primer sequences are shown in () and were purchased from Integrated DNA Technologies (Integrated DNA Technologies, Coralville, IA). qPCR was prepared following the protocol from Promega and run in duplicates (GoTaq qPCR, Promega, WI). qPCR data were analyzed by averaging the duplicates and using the ΔΔCt method with RING finger protein 7 (RNF7) as the reference gene to determine the fold change compared to baseline expression.
Table 1. Primer sequences.
2.4. ELISA
Plasma cytokines were determined by a pig specific ELISA following the protocols provided (IL-1β was purchased from Abcam®, Cambridge, MA, and IFN-γ, IL-6, TNF-α, and IL-10 were purchased from Invitrogen, Thermo Fisher, Waltham, MA). IL-1β, IL-6, IL-10, and TNF-α ELISA were 5 step procedures with antigen binding, biotin conjugate binding, Streptavidin-HRP binding, chromagen incubation, and stop solution addition. The IFN-γ ELISA was a 4 step procedure with antigen and biotin binding combined in one step. All plates were read within 15 min at 450 nm and the cytokine concentration was determined based on the concentration of the standards.
2.5. Statistics
For each cytokine, the signed-rank test was used to assess whether the distribution of relative concentrations (i.e., fold changes relative to baseline) was shifted away from 1. Spearman’s rank correlation coefficient (rs) was used to describe the association between each cytokine’s relative concentration and either time to ARDS or the P/F ratio at baseline. All analyses were performed using R (v 4.2.0).
3. Results
Inflammatory cytokine mRNA expression in WBCs was determined in 17 female pigs except for IL-17 mRNA, which was analyzed in 16 animals. Plasma IL-1β protein concentration was determined in 19 female pigs with 14 being included in the cytokine mRNA analysis. All animals showed a significant drop in pH, PaO2, and a significant increase in PaCO2 comparing baseline with ARDS values (). Median (IQR) time to achieve ARDS was 107 (92–139) minutes with a median volume of 7.4 (5.3–9.1) mL oleic acid infused. Laboratory and physiologic data prior and following ARDS induction are summarized in and . On average, the P/F ratio, once ARDS was achieved, dropped from 594 (95% CI; 575–615) to 270 (95% CI; 256–291). The oxygenation index once ARDS was achieved increased significantly from 1.71 at baseline to 4.48 mL/cmH2O by 2.77 (95% CI: 2.51–3.02; p < 0.001), indicating mild ARDS per PALICC definition. Similarly, the average Vd/Vt ratio, once ARDS was achieved was 0.43 mL/cmH2O compared to 0.28 mL/cmH2O at baseline, a significant increase by 0.15 (95% CI: 0.12–0.19; p < 0.001) mL/cmH2O) translating to the ARDS response being 169% (95% CI: 146–192%) greater than the baseline response. Further supporting the development of ARDS is the decline of compliance with the average response, once ARDS achieved, being 4.21 (95% CI: 3.07–5.34; p < 0.001) mL/cmH2O lower than the response at baseline (7.16 vs 11.4 mL/cmH2O), which roughly translates to the compliance at ARDS being 35% (95% CI: 28–42%) less than the baseline response.
Figure 1. Average response of oxygenation index, Vd/Vt ratio, and compliance comparing baseline with ARDS.
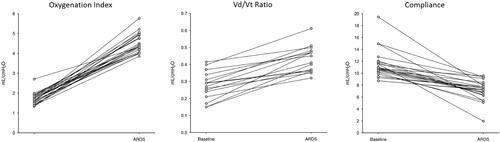
Table 2. Laboratory and physiology data.
We tested WBC mRNA expression of the cytokines IFN-γ, TNF-α, IL-6, IL-10, and IL-17. Two of these cytokines, IFN-γ (p = 0.006) and IL-6 (p = 0.003), showed a significantly reduced mRNA expression at ARDS compared to baseline. The median concentration of IFN-γ mRNA was estimated to be 59% (95% CI: 40.9–85.6%) of the baseline amount, while the median mRNA expression of IL-6 was 44.4% (95% CI: 25.6–77.1%) of its baseline level (). Other cytokines (TNF-α, IL-17, IL-10) had relative concentrations that did not systematically deviate from 1 (p > 0.15 for each). Relative cytokine concentrations were not associated with time to ARDS (p ≥ 0.07) (). Neither was there any association between the P/F ratio at baseline and relative cytokine concentration (p ≥ 0.10).
Figure 2. Fold changes (ARDS relative to baseline) for cytokines measured using RT-qPCR. During ARDS, INF-γ and IL-6 decreased to 59% and 44% of its baseline value, respectively. TNF-α, IL-17, and IL-10 had non-significant fold changes.
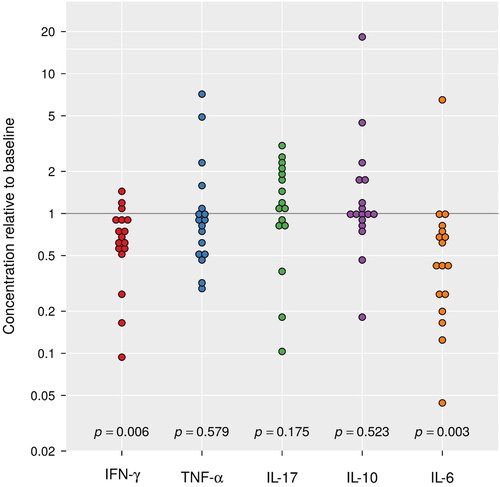
Figure 3. No significance between time to ARDS and cytokine levels. Relative concentrations of mRNA of cytokines had no significant correlation with time it induces ARDS.
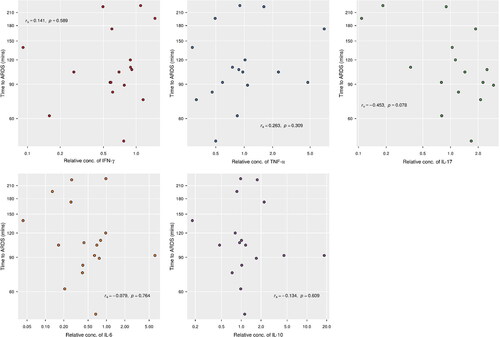
Plasma cytokine concentration was measured by ELISA in n = 19 pigs. (Of these 19, 14 were among the 17 used in the earlier analysis and five were specific to just this one cytokine. Likewise, 3 of the original 17 animals were particular to that earlier data set and had no protein data.) The full collection of 19 animals was used for analysis of relative IL-1β concentrations while the two correlational analyses used data from 18 of the animals (time to ARDS and P/F ratio at baseline missing for one pig).
The relative plasma concentration of IL-1β and IL-10 at ARDS did not significantly differ (p = 0.332 and 0.113 respectively) from baseline (). Neither was there any noted association between relative concentrations of IL-1β and the P/F ratio at baseline (rs = 0.18, p = 0.47). There was some evidence (p = 0.026) of a direct association between time to ARDS and the relative concentration of IL-1β (rs = 0.52) such that increases in one variable are associated with an increase in the other ().
4. Discussion
ARDS is a heterogeneous disease and animal models are instrumental in untangling pathophysiology and disease progression. Although every model has limitations compared to human disease, knowing the onset time and type of insult enables tracking pathological changes over time.Citation21,Citation22 Oleic acid infusion is an established model of lung injury and ARDS, and often used to study inflammatory changes and potential treatments.Citation23–25 However, early changes of inflammatory markers before reaching ARDS are not frequently captured in clinical studies.Citation9 In the present study, we analyzed changes in inflammatory mRNA expression in blood leukocytes before and immediately after intravenous oleic acid infusion. In addition, we analyzed the plasma protein concentration of the same inflammation markers. Our data show decreased IFN-γ and IL-6 mRNA expression in WBC compared to baseline values. In contrast, the plasma IL-6 and IFN-γ concentrations were increased when reaching ARDS compared to baseline. This finding holds paramount importance as recognizing early changes in inflammatory markers may contribute to a better understanding of disease subtypes and mechanisms leading to ARDS. Furthermore, our findings hold promise in aiding clinicians better to comprehend the role of inflammatory markers during ARDS progression, enabling them to navigate the limitations and opportunities associated with using these markers in the management and monitoring of ARDS
Oleic acid, a common fatty acid in the human body, is transported bound to albumin or as lipoproteins, preventing cytotoxicity. Free fatty acids can change membrane structure and cause vascular leakage and therefore oleic acid infusion was used as a model to reproduce ARDS to investigate ventilation strategies, pathophysiology, or interventions.Citation12,Citation14,Citation17,Citation18,Citation23,Citation24,Citation26–30 This model was established for acute lung injury in sheep, dogs, and rats and used in many other species.Citation31–36 The injection procedure varies substantially between protocols. Single injections, multiple injections, and continuous infusion over a given time are the most common methods.Citation37–39 Single bolus injection caused the animals to be unstable and unsuitable for the experiment, which was also reported by Borges et al., who described high mortality and limited reproducibility using a single dose.Citation40 Therefore, we chose a continuous infusion initiated at a rate of 0.05 mL/kg/minute and titrated up to 0.6 mL/kg/minute every 15 min resulting in 5.25 ml oleic acid after 90 min. This protocol was adopted from Sum-Ping et al.Citation41 In our experiment the mortality was very low with 3 animals dying within the first 50 animals.
Effective treatment of ARDS is challenging due to ARDS heterogeneity and timing of treatment. Using subtype, phenotype, and biomarkers therefore could be very beneficial to classify ARDS and optimize therapy.Citation42–44 Accordingly, two ARDS sub-phenotypes, hyperinflammation and hypoinflammation, determined by plasma biomarkers, react differently to treatment.Citation45–47 Hyperinflammation is characterized by elevated IL-6 and IL-8 plasma levels, which in contrast are reduced in hypoinflammation.Citation46,Citation48,Citation49 A recent study of adult ARDS patients showed that bronchoalveolar lavage fluid (BALF)-derived classification was associated with clinical severity of pulmonary disease differed from plasma-derived inflammation-based grouping.Citation50 Patients selected for this study were on invasive mechanical ventilation within 48 h of ARDS onset, hence severe disease. IL-6 and IL-8 protein concentration in plasma were not different after a Bonferroni correction for multiple hypothesis tests but highly significantly different in BALF.Citation50 Moreover, serum high mobility group box 1 (HMGB1) protein levels in pediatric living donor liver transplantation (LDLT) recipients at 30 min after reperfusion were positively associated with early PARDS.Citation51 Sepsis patients who would develop ARDS later had increased expression of Bactericidal Permeability-Increasing Protein (BPI), Olfactomedin 4 (OLFM4), Lipocalin2 (LCN2), CD24, matrix metalloprotease-8 (MMP8) and decreased expression of MME at an early time compared to patients who did not develop ARDS.Citation52 All these gene products are related to pathways of the innate immune system. This indicates that timing of sample acquisition, sample source, and mechanism of injury are important variables to identify subtypes and phenotypes in ARDS.
The analysis of our samples included the comparison of pre- to post-injury inflammatory markers in white blood cells and plasma. The median time of 107 (92–139) minutes to develop ARDS may explain why our data are differ from most published studies. The noted reduction in IFN-γ and IL-6 mRNA expression and increase in plasma IFN-γ and IL-6 may therefore be a transient event. The correlation between the duration of the oleic acid infusion and volume with IL-1 protein plasma concentration supports this notion. Most published data were obtained at some time past ARDS onset. In addition, the control in our study was taken from the same pig prior to oleic acid injection, rather than a separate control animal or group. We compared cytokine protein or mRNA levels at ARDS with baseline levels in the same animal before OA infusion, while in most human studies, scientists measure cytokines or biomarkers at ARDS or at admission into the ICU/PICU comparing them to post-treatment data or control patients without ARDS. However, the difference between mRNA and protein data demonstrate that the interpretation of biomarker analysis depends on the specimen and kind of component, mRNA or protein. Fine-tuning of transcription, translation, and post-translational regulation enables the correct function of complex systems.Citation53,Citation54 It also shows the importance of sample acquisition time.
Finally, to incorporate the clinical significance of our findings, it is imperative to underline the pivotal role of early identification of inflammatory markers in the progression of ARDS. The dynamics of cytokine expression, particularly IFN-γ, and IL-6, as elucidated in our study, could potentially provide a critical window for early recognition of ARDS, as the early detection of these markers could potentially herald the onset of ARDS, allowing for preemptive therapeutic strategies to ameliorate or even prevent the progression of this life-threatening condition. As highlighted above, human studies on ARDS face inherent limitations due to the rarity of capturing patients at the onset of the syndrome, thus restricting the ability to understand the early pathophysiological events fully. In this context, our study gains significant relevance by offering insights into the early inflammatory response within a controlled translational experimental setup, thereby helping with addressing the challenges faced in clinical settings. As our understanding of the molecular underpinnings of ARDS deepens, so too does the potential for developing targeted therapies that can mitigate the severity or even prevent the onset of ARDS in at-risk populations. In conclusion, the early identification of inflammatory markers could represent a critical juncture in the quest for improving ARDS outcomes. Our study provides a rationale for the investigation and potential inclusion of these biomarkers in the diagnostic and therapeutic approaches to ARDS, underscoring the need for further research in this domain.
Our study has many limitations: first, we were not able to obtain bronchoalveolar lavage fluid due to hemodynamic instability associated with our model and the defined endpoints of the experiments. Second, we did not obtain protein data on IFN-γ or IL-6. Further studies are required to better characterize the short-term changes in cytokine expression and the consequences for the progression of lung injury. Third, unanswered in this study is the question about the reason for the reduced mRNA expression in blood at the analyzed time point. Is it a downregulation of this mRNA expression or are cells expressing this mRNA migrating to the injured tissue, spleen, or lymph nodes? And forth, we did not analyze lymphocyte subpopulations and expression of cytokine mRNA and protein.
In conclusion, our study showed that the oleic-acid swine model of pediatric ARDS is a good model to study early inflammatory changes during lung injury simultaneously with heart and lung physiology. IFN-γ and IL-6 mRNA expression was significantly reduced in WBCs in less than 2 h at the time when ARDS was attained whereas IFN-γ and IL-6 protein concentration increased. These changes do not indicate the presence of ARDS, which needs to be determined by clinical criteria. However, they show that interpretation of data from a single class of biological component may not be enough for clinical interpretation. Further studies are required to define this reduction, which may be caused by cell migration as it has been observed in COVID-19 patients, change in mRNA expression, or both.Citation55 Future studies to identify subphenotypes of ARDS should also include the analysis of non-coding RNA including micro-RNA (miRNAs), circular RNA (circRNA), and long non-coding RNA (lncRNA). All these RNAs are involved in the regulation of inflammation and are differentially expressed between subjects who did and did not develop ARDS.Citation56–58
5. Impact
The onset of acute respiratory distress (ARDS) is reflected by reduced IFN-γ and IL-6 mRNA expression in WBC and simultaneous increased IFN-γ and IL-6 plasma concentration. For clinical use as biomarkers the time of sample acquisition and analysis method is of great importance.
Identifying early inflammatory changes may be important to characterize ARDS and initiate treatments to ameliorate disease progression.
Acknowledgments
We thank Jennifer Frank for her diligent work in collecting data during experiments. We thank Everett J Temme for helping handling the animals and performing CPR.
Declaration of interest
No potential conflict of interest was reported by the author(s).
Additional information
Funding
References
- Huppert LA, Matthay MA, Ware LB. Pathogenesis of acute respiratory distress syndrome. Semin Respir Crit Care Med. 2019;40(1):31–39. doi:10.1055/s-0039-1683996.
- Matthay MA, Zemans RL, Zimmerman GA, et al. Acute respiratory distress syndrome. Nat Rev. Dis Primers. 2019;5:18.
- Ranieri VM, Rubenfeld GD, Thompson BT, et al. Acute respiratory distress syndrome: the Berlin definition. JAMA. 2012;307:2526–2533.
- Yehya N, Smith L, Thomas NJ, et al. Definition, incidence, and epidemiology of pediatric acute respiratory distress syndrome: from the second pediatric acute lung injury consensus conference. Pediatr Crit Care Med. 2023;24(12 Suppl 2):S87–s98. doi:10.1097/PCC.0000000000003161.
- ten Duis HJ. The fat embolism syndrome. Injury. 1997;28(2):77–85. doi:10.1016/s0020-1383(96)00085-x.
- Inoue H, Nakagawa Y, Ikemura M, Usugi E, Nata M. Molecular-biological analysis of acute lung injury (Ali) induced by heat exposure and/or intravenous administration of oleic acid. Leg Med (Tokyo). 2012;14(6):304–308. doi:10.1016/j.legalmed.2012.06.003.
- Xu GL, Yao L, Rao SY, Gong ZN, Zhang SQ, Yu SQ. Attenuation of acute lung injury in mice by oxymatrine is associated with inhibition of phosphorylated P38 mitogen-activated protein kinase. J Ethnopharmacol. 2005;98(1-2):177–183. doi:10.1016/j.jep.2005.01.026.
- Chen HI, Hsieh NK, Kao SJ, Su CF. Protective effects of propofol on acute lung injury induced by oleic acid in conscious rats. Crit Care Med. 2008;36(4):1214–1221. doi:10.1097/CCM.0b013e31816a0607.
- Ballard-Croft C, Wang D, Sumpter LR, Zhou X, Zwischenberger JB. Large-animal models of acute respiratory distress syndrome. Ann Thorac Surg. 2012;93(4):1331–1339. doi:10.1016/j.athoracsur.2011.06.107.
- Wong JJ-M, Jit M, Sultana R, et al. Mortality in pediatric acute respiratory distress syndrome: a systematic review and meta-analysis. J Intensive Care Med. 2019;34(7):563–571. doi:10.1177/0885066617705109.
- Zhou L, Yang R, Xue C, et al. Biphasic positive airway pressure spontaneous breathing attenuates lung injury in an animal model of severe acute respiratory distress syndrome. BMC Anesthesiol. 2022;22(1):228. doi:10.1186/s12871-022-01763-w.
- Puuvuori E, Chiodaroli E, Estrada S, et al. Pet imaging of neutrophil elastase with (11)C-Gw457427 in acute respiratory distress syndrome in pigs. J Nucl Med. 2022;64(3):423–429. doi:10.2967/jnumed.122.264306.
- Rissel R, Renz M, Mohnke K, et al. Comparison of two porcine acute lung injury models: a post-hoc analysis. Intensive Care Med Exp. 2022;10:37.
- Rohrs EC, Bassi TG, Nicholas M, et al. Negative-pressure-assisted ventilation lowers driving pressure and mechanical power in an ards model. J Appl Physiol (1985). 2022;133(6):1237–1249. doi:10.1152/japplphysiol.00486.2022.
- Dickson RP, Hotchkin DL, Lamm WJE, et al. A porcine model for initial surge mechanical ventilator assessment and evaluation of two limited-function ventilators. Crit Care Med. 2011;39(3):527–532. doi:10.1097/CCM.0b013e318206b99b.
- Judge EP, Hughes JM, Egan JJ, Maguire M, Molloy EL, O’Dea S. Anatomy and bronchoscopy of the porcine lung. a model for translational respiratory medicine. Am J Respir Cell Mol Biol. 2014;51(3):334–343. doi:10.1165/rcmb.2013-0453TR.
- Abram J, Martini J, Spraider P, et al. Individualised flow-controlled versus pressure-controlled ventilation in a porcine oleic acid-induced acute respiratory distress syndrome model. Eur J Anaesthesiol. 2023;40(7):511–520. doi:10.1097/EJA.0000000000001807.
- Brusatori S, Zinnato C, Busana M, et al. High- versus low-flow extracorporeal respiratory support in experimental hypoxemic acute lung injury. Am J Respir Crit Care Med. 2023;207(9):1183–1193. doi:10.1164/rccm.202212-2194OC.
- Tohyama S, Kobayashi E. Age-appropriateness of porcine models used for cell transplantation. Cell Transplant. 2019;28(2):224–228. doi:10.1177/0963689718817477.
- Pediatric Acute Respiratory Distress Syndrome. Consensus recommendations from the pediatric acute lung injury consensus conference. Pediatr Crit Care Med. 2015;16:428–439.
- Matthay MA, Ware LB, Zimmerman GA. The acute respiratory distress syndrome. J Clin Invest. 2012;122(8):2731–2740. doi:10.1172/JCI60331.
- Matute-Bello G, Downey G, Moore BB, et al. An official American thoracic society workshop report: features and measurements of experimental acute lung injury in animals. Am J Respir Cell Mol Biol. 2011;44(5):725–738. doi:10.1165/rcmb.2009-0210ST.
- Abedi F, Hayes AW, Reiter R, Karimi G. Acute lung injury: the therapeutic role of rho kinase inhibitors. Pharmacol Res. 2020;155:104736. doi:10.1016/j.phrs.2020.104736.
- Millar JE, Bartnikowski N, von Bahr V, et al. Extracorporeal membrane oxygenation (Ecmo) and the acute respiratory distress syndrome (Ards): a systematic review of pre-clinical models. Intensive Care Med Exp. 2019;7:18.
- Gonçalves-de-Albuquerque CF, Silva AR, Burth P, Castro-Faria MV, Castro-Faria-Neto HC. Acute respiratory distress syndrome: role of oleic acid-triggered lung injury and inflammation. Mediators Inflamm. 2015;2015:260465–260469. doi:10.1155/2015/260465.
- Bassi TG, Rohrs EC, Fernandez MKC, et al. Diaphragm neurostimulation mitigates ventilation-associated brain injury in a preclinical acute respiratory distress syndrome model. Crit Care Explor. 2022;4(12):e0820. doi:10.1097/CCE.0000000000000820.
- Rissel R, Gosling M, Ruemmler R, Ziebart A, Hartmann EK, Kamuf J. Bronchoalveolar lavage and oleic acid-injection in pigs as a double-hit model for acute respiratory distress syndrome (Ards). J Vis Exp. 2020.
- Rissel R, Kirchner L, Renz M, et al. Resveratrol influences pulmonary mechanics and inflammatory response in a porcine Ards model. Life Sci. 2023;319:121410. doi:10.1016/j.lfs.2023.121410.
- Gonçalves-de-Albuquerque CF, Silva AR, Burth P, Castro-Faria MV, Castro-Faria-Neto HC. In Handbook of Lipids in Human Function. (Watson, R. R. & De Meester, F. eds.) p. 605–634. Champaign, Illinois: AOCS Press, 2016.
- Yang R, Zhou L, Chen Z, et al. Effect and mechanical mechanism of spontaneous breathing on oxygenation and lung injury in mild or moderate animal ards. BMC Pulm Med. 2023;23(1):428. doi:10.1186/s12890-023-02730-y.
- Osher AB, Gothe B, Simmons DH, Waymost B, Green H. Effects of continuous positive airway pressure after oleic acid-induced lung injury in dogs. Pediatr Res. 1978;12(9):923–926. doi:10.1203/00006450-197809000-00008.
- Griffith BP, Carroll RG, Hardesty RL, Peel RL, Borovetz HS. Selected lobar injury after infusion of oleic acid. J Appl Physiol Respir Environ Exerc Physiol. 1979;47(4):706–711. doi:10.1152/jappl.1979.47.4.706.
- Dickey BF, Thrall RS, McCormick JR, Ward PA. Oleic-acid-induced lung injury in the rat. failure of indomethacin treatment or complement depletion to ablate lung injury. Am J Pathol. 1981;103(3):376–383.
- Zwissler B, Forst H, Ishii K, Messmer K. A new experimental model of ards and pulmonary hypertension in the dog. Res Exp Med (Berl). 1989;189(6):427–438. doi:10.1007/BF01855010.
- Coalson JJ, King RJ, Winter VT, et al. O2- and pneumonia-induced lung injury. I. Pathological and morphometric studies. J Appl Physiol (1985). 1989;67(1):346–356. doi:10.1152/jappl.1989.67.1.346.
- Smith RA, Johnson M, Venus B. Does continuous positive airway pressure compensate for loss of glottic function during tracheal intubation? Crit Care Med. 1990;18(8):848–850. doi:10.1097/00003246-199008000-00011.
- Brown BH, Flewelling R, Griffiths H, et al. Eits changes following oleic acid induced lung water. Physiol Meas. 1996;17 Suppl 4A:A117–130. doi:10.1088/0967-3334/17/4a/016.
- Overbeck MC, Pranikoff T, Yadao CM, Hirschl RB. Efficacy of perfluorocarbon partial liquid ventilation in a large animal model of acute respiratory failure. Crit Care Med. 1996;24(7):1208–1214. doi:10.1097/00003246-199607000-00024.
- Mutch WA, Harms S, Lefevre GR, Graham MR, Girling LG, Kowalski SE. Biologically variable ventilation increases arterial oxygenation over that seen with positive end-expiratory pressure alone in a porcine model of acute respiratory distress syndrome. Crit Care Med. 2000;28(7):2457–2464. doi:10.1097/00003246-200007000-00045.
- Borges AM, Ferrari RS, Thomaz LDGR, et al. Challenges and perspectives in porcine model of acute lung injury using oleic acid. Pulm Pharmacol Ther. 2019;59:101837. doi:10.1016/j.pupt.2019.101837.
- Sum-Ping ST, Symreng T, Jebson P, Kamal GD. Stable and reproducible porcine model of acute lung injury induced by oleic acid. Crit Care Med. 1991;19(3):405–408. doi:10.1097/00003246-199103000-00021.
- Battaglini D, Fazzini B, Silva PL, et al. Challenges in ards definition, management, and identification of effective personalized therapies. J Clin Med. 2023;12(4):1381. doi:10.3390/jcm12041381.
- Calfee CS, Delucchi K, Parsons PE, Thompson BT, Ware LB, Matthay MA, NHLBI ARDS Network. Subphenotypes in acute respiratory distress syndrome: latent class analysis of data from two randomised controlled trials. Lancet Respir Med. 2014;2(8):611–620. doi:10.1016/S2213-2600(14)70097-9.
- Calfee CS, Delucchi KL, Sinha P, et al. Acute respiratory distress syndrome subphenotypes and differential response to simvastatin: secondary analysis of a randomised controlled trial. Lancet Respir Med. 2018;6(9):691–698. doi:10.1016/S2213-2600(18)30177-2.
- Hendrickson KW, Peltan ID, Brown SM. The epidemiology of acute respiratory distress syndrome before and after coronavirus disease 2019. Crit Care Clin. 2021;37(4):703–716. doi:10.1016/j.ccc.2021.05.001.
- Spadaro S, Park M, Turrini C, et al. Biomarkers for Acute Respiratory Distress Syndrome And Prospects For Personalised Medicine. J Inflamm (Lond). 2019;16(1):1. doi:10.1186/s12950-018-0202-y.
- Carlton EF, Flori HR. Biomarkers in pediatric acute respiratory distress syndrome. Ann Transl Med. 2019;7(19):505–505. doi:10.21037/atm.2019.09.29.
- Calfee CS, Janz DR, Bernard GR, et al. Distinct molecular phenotypes of direct vs indirect ards in single-center and multicenter studies. Chest. 2015;147(6):1539–1548. doi:10.1378/chest.14-2454.
- Sinha P, Calfee CS. Phenotypes in acute respiratory distress syndrome: moving towards precision medicine. Curr Opin Crit Care. 2019;25(1):12–20. doi:10.1097/MCC.0000000000000571.
- Sathe NA, Morrell ED, Bhatraju PK, et al. Alveolar biomarker profiles in subphenotypes of the acute respiratory distress syndrome. Crit Care Med. 2023;51(1):e13–e18. doi:10.1097/CCM.0000000000005704.
- Cao Y, Zhi J, Ren H, et al. Association between serum hmgb1 elevation and early pediatric acute respiratory distress syndrome: a retrospective study of pediatric living donor liver transplant recipients with biliary atresia in China. BMC Anesthesiol. 2023;23(1):87. doi:10.1186/s12871-023-02040-0.
- Yao R-Q, Shen Z, Ma Q-M, et al. Combination of transcriptional biomarkers and clinical parameters for early prediction of sepsis indued acute respiratory distress syndrome. Front Immunol. 2022;13:1084568. doi:10.3389/fimmu.2022.1084568.
- Perl K, Ushakov K, Pozniak Y, et al. Reduced changes in protein compared to mRNA levels across non-proliferating tissues. BMC Genomics. 2017;18(1):305. doi:10.1186/s12864-017-3683-9.
- Koussounadis A, Langdon SP, Um IH, Harrison DJ, Smith VA. Relationship between differentially expressed mRNA and mRNA-protein correlations in a xenograft model system. Sci Rep. 2015;5(1):10775. doi:10.1038/srep10775.
- Nasrollahi H, Talepoor AG, Saleh Z, et al. Immune responses in mildly versus critically ill Covid-19 patients. Front Immunol. 2023;14:1077236. doi:10.3389/fimmu.2023.1077236.
- Zhang C, Ji Y, Wang Q, Ruan L. Mir-338-3p is a biomarker in neonatal acute respiratory distress syndrome (Ards) and has roles in the inflammatory response of Ards cell models. Acta Med Okayama. 2022;76:635–643.
- Li K, Huang Z, Tian S, et al. Microrna-877-5p alleviates Ards via enhancing Pi3k/Akt path by targeting Cdkn1b both in vivo and in vitro. Int Immunopharmacol. 2021;95:107530. doi:10.1016/j.intimp.2021.107530.
- Wang Q, Feng Q, Zhang Y, Zhou S, Chen H. Decreased microrna 103 and microrna 107 predict increased risks of acute respiratory distress syndrome and 28-day mortality in sepsis patients. Medicine (Baltimore). 2020;99(25):e20729. doi:10.1097/MD.0000000000020729.