Abstract
Thermal dose and absorbed radiation dose have historically been difficult to compare because different biological mechanisms are at work. Thermal dose denatures proteins and the radiation dose causes DNA damage in order to achieve ablation. The purpose of this paper is to use the proportion of cell survival as a potential common unit by which to measure the biological effect of each procedure.
Survival curves for both thermal and radiation doses have been extracted from previously published data for three different cell types. Fits of these curves were used to convert both thermal and radiation dose into the same quantified biological effect: fraction of surviving cells. They have also been used to generate and compare survival profiles from the only indication for which clinical data are available for both focused ultrasound (FUS) thermal ablation and radiation ablation: essential tremor thalamotomy.
All cell types could be fitted with coefficients of determination greater than 0.992. As an illustration, survival profiles of clinical thalamotomies performed by radiosurgery and FUS are plotted on a same graph for the same metric: fraction of surviving cells.
FUS and Gamma Knife have the potential to be used in combination to deliver a more effective treatment (for example, FUS may be used to debulk the main tumour mass, and radiation to treat the surrounding tumour bed). In this case, a model which compares thermal and radiation treatments is valuable in order to adjust the dose between the two.
Introduction
Therapies involving the use of ionising radiation and/or thermal energy have a long history in the treatment of disease, including cancer. Evidence from an Egyptian papyryus suggests that as early as 3500 years ago heat was applied in an attempt to treat breast cancer [Citation1,Citation2]. Therapy involving radiation had to wait until the discovery of X-rays in 1895, however within several years of this discovery, radiation therapy based on radionuclides and low-energy X-ray generating equipment was used for the treatment of cancer [Citation3]. More recently, there have been parallel developments in the use of heat and ionising radiation for both diffuse and focal disease, as well as attempts to combine the benefit of the two modalities. A number of techniques have been developed to allow the focal destruction of malignant tissue in humans, including ablation by radiofrequency [Citation4], microwaves [Citation5–12], lasers [Citation13], magnetic nanoparticles [Citation14,Citation15] and high-intensity focused ultrasound [Citation16–18]. Recent advancements in the focal treatment of cancer using ionising radiation include the development of stereotractic radiosurgery (SRS) [Citation19], stereotactic body radiotherapy (SBRT) [Citation20–22] and intraoperative radiotherapy (IORT) [Citation23,Citation24].
From an early date and continuing to the present, investigation of the synergies between heat and ionising radiation has been a natural avenue for research including the basic biology of hyperthermia [Citation25], the most effective sequencing of heating and radiation [Citation26,Citation27], biological interactions between heat and radiation [Citation28] and determination of thermal enhancement ratios and predictors of response [Citation28–30]. Significant evidence in the form of several randomised control trials exists to demonstrate that hyperthermia followed by radiation can significantly improve outcomes in head and neck cancer [Citation31,Citation32], malignant melanoma [Citation33], breast cancer [Citation34], glioblastoma multiforme [Citation35], pelvic tumours [Citation36], cervical carcinoma [Citation37], superficial tumours [Citation38], cervical cancer [Citation39], non-small-cell lung cancer [Citation40], rectal cancer [Citation41] among others [Citation2,Citation42]. The recent progress in focal ablative therapies such as HIFU and SRS/SBRT may also benefit from combined approaches. However,direct comparison of absorbed dose in ionising radiation and thermal dose for heating have historically been difficult because of the widely different physical and biological mechanisms in play [Citation1].
In this work, we compare thermal and ionising radiation modalities in terms of biological damage to tissue by using equivalent historical in vitro cell survival data. By doing so, we aim to create a method for translation between measures of thermal and radiation dose. Essential tremor thalamotomy is currently the only indication for which quantitative clinical data are available for both radiation and FUS treatments. In order to illustrate this approach, we thus took into account the beam-shaping capabilities of the stereotactic radiosurgery (SRS) and FUS devices available for treatment of essential tremor, in order to allow more direct comparison between FUS and SRS treatments. Our approach could facilitate dosimetry planning in the setting of combined radiosurgery and FUS.
Biological effect of ionising radiation and definition of radiation dose
Photon-based ionising radiation (X-rays and γ-rays) are indirectly ionising; they deposit energy in tissue in a two-step process. In the first step, photolectric and Compton interactions between photons and atoms in tissue result in the transfer of energy to fast electrons ejected from the target atoms. In a second step, atoms in the targeted tissue are ionised as these fast electrons undergo Coulomb interactions with other atoms in the targeted tissue, transferring a fraction of their energy during each interaction [Citation43]. In traditional radiobiology theory, the biological target of ionising radition is damage to DNA. In a minority of cases (∼33%), DNA is directly ionised, leading to strand breaks. In the majority of cases (∼66%), the ions created in irradiated tissue result in the creation of free radicals (most significantly hydroxyl radicals) which subsequently react with and create strand breaks in DNA. The ultimate biological effect of the DNA damage is mitotic death of the cells in the irradiated tissue [Citation1]. At higher, ablative doses there may additional biological mechanisms at play as well, including microvascular damage [Citation44].
Radiation dose is described in physical terms: the amount of energy absorbed per mass of tissue. This is the definition of “absorbed dose”, and is most commonly assigned units of Gray (Gy), where 1 Gy = 1 J/kg of energy absorbed in tissue [Citation43]. The amount of biological damage caused can be related directly to the physical quantity of absorbed dose, and this has become the standard method of prescribing the appropriate amount of radiation to be delivered in any given therapeutic setting.
Biological effect of thermal energy and definition of thermal dose
The response of cells to thermal insult at sub-ablative temperatures (such as temperatures employed in traditional hyperthermia) can be quite complex, involving the development of a combination of thermotolerance, cytotoxicity and radiosensitisation most likely mediated by protein denaturation within cells which increases proportionately to the thermal damage. Lower level of protein denaturation can trigger protective mechanisms such as increased expression of heat shock proteins which help to stabilise the cell against further thermal damage. Higher levels of protein denaturation can cause the inactivation of protein synthesis and DNA repair mechanisms resulting in cytotoxity and increased sensitivity to ionising radiation [Citation45]. When delivered at sufficient power to result in ablation, thermal energy ultimately results in coagulative necrosis [Citation46]. As with ionising radiation, the extent of biological changes in tissue resulting from thermal exposure is correlated with the amount of energy absorbed in tissue. However, for thermal energy, it is the temperature to which the tissue is raised, and the duration of the heating that seem to play the predominent biological role. Sapareto and Dewey have defined a “thermal isoeffective dose” [Citation47]. This has units of cumulative equivalent minutes at 43 °C (CEM 43 °C), and allows conversion of any temperature/time (T/t) combination to the equivalent time for which the reference temperature of 43 °C must be applied to obtain the same level of thermal damage:
The definition of the thermal isoeffective dose formalises the idea that two different temperatures applied over different time intervals can have the same biological effect in a given tissue.
Cell survival curves
Traditionally, the most common method for assessing cell survival in thermo- and radiobiology uses survival curves. The principle of survival curve analysis is the same, irrespective of the source of cell damage. The curve depicts the relationship between the damage to which a cell has been subjected and the cell’s subsequent ability to divide to form a colony. For the studies considered here, in vitro assays have been used as the source data. The development of survival curves involve plating a known number of cells (determined using a standard counting method) out into tissue culture dishes following exposure to heat or ionising radiation, and analysing their response at a later time point. The surviving fraction is calculated by normalising the number of cells or colonies seen in treated samples to that seen in controls. This is then plotted on a logarithmic scale against the thermal or radiation dose delivered [Citation1]. A minimum colony size (usually 50 cells) is used as a cut-off for minimal critical mass of cells to survive as a colony. It should be noted, that while this type of assay is frequently assumed to measure individual cell survival directly, the reduction in number after treatment may also be due in part to cells going into a “dormant” state, and not dividing [Citation1].Typical survival curves are shown in for radiotherapy and thermal doses. For thermal treatments, the cells were subjected to a variety of temperatures as shown in the figure legend. However, when both temperature and time are taken into account by calculating thermal dose in CEM, the data fall along a common curve.
Figure 1. An example of a survival curve for radiotherapy exposure (A), extracted from [Citation97], and for heated cells (B), extracted from previously published results [Citation98], with thermal isoeffective dose calculated from the combination of heating duration and temperature.
![Figure 1. An example of a survival curve for radiotherapy exposure (A), extracted from [Citation97], and for heated cells (B), extracted from previously published results [Citation98], with thermal isoeffective dose calculated from the combination of heating duration and temperature.](/cms/asset/4f1c88ff-c232-42f2-9f2c-c66002b6f1db/ihyt_a_1278281_f0001_b.jpg)
Materials and methods
Literature review
An extensive review of the literature was conducted to identify studies reporting cell survival for thermal exposure, with the goal of identifying cell subtypes for which there were matching published reports on cell survival for ionising radiation. Basically a literature review for both thermal and radiation cell survival was performed and then data were cross referenced. The literature review was conduced using Cornell University’s online library during June and July of 2013. Keywords used in this literature review included: cell survival, radiation treatment, thermal treatment, thermal dose, radiation dose, tissue damage and hyperthermia. Some exclusion criteria included: articles not written in English, studies that only used thermal and radiation treatment in combination and studies that did not measure cell survival. lists the publications selected for thermal and ionising radiation modalities. The list is not exhaustive and the literature search was stopped when three different cell-types were found for which survival data were available: Chinese hamster ovary cells (CHO), Chinese hamster lung cells (CHL) and human glioblastoma tumour cells will thus be used in the rest of the article to illustrate our approach.
Table 1. Review of papers reporting survival of cells exposed to thermal rise or ionising radiations, matched for cell subtype and dose range.
Data extraction and cell survival modelling
Each point of the survival data, as displayed in the reports selected for thermal and ionising radiation experiments, was manually transferred into Matlab version R2013a (Mathworks, Inc., Natick, MA) for each cell type: CHO, CHL and human glioblastoma astrocytoma (GBA). The radiation dose survival profiles for each of the three cell types were modelled using the Universal Survival Curve (USC) developed by Park et al. [Citation48]. The USC is one of several derivatives of the linear quadratic model that attempt to take into account low and high doses survival regimes [Citation44,Citation49–53]:
where, S is the cell survival, defined as the ratio between the remaining number of undamaged cells at time t indicated by N(t) to the number of undamaged cells N(0) present prior to the start of the treatment, Dr is the radiation dose in Gray, n is the number of fractionations and α (units of loge of the cells killed per Gy) and β (units loge of cells killed per Gy2) are constants that describe the linear and quadratic portions of the curve, respectively. DT is the transition dose, where the curve changes from using the low-dose model to the high dose. Do is a measure of the slope of the linear portion of the curve at high doses and Dq is the x-intercept of the survival line valid for Dr ≤ DT . β and DT are calculated from the parameters α, Do, and Dq to ensure continuity and differentiability at DT [Citation48]:
In traditional radiobiology, the α and β terms above are also often represented in ratio form (the α/β ratio, units of Gy), which is the dose at which the linear and quadratic components are equal. Tumours and tissue which show an early response to radiation damage tend to have a large α/β ratio, while tissue which shows a late response to radiation damage have a small α/β ratio [Citation1,Citation53,Citation54]. Thermal dose survival data were fitted with a linear-quadratic equation for all three cell types [Citation55,Citation56]:
where, S is the cell survival, Dt is the thermal dose in equivalent minutes and a and b are constants.
Damage index Ω is widely used for RF and laser ablation. It is the logarithm of the ratio between the number of undamaged cells N(0) present prior to the start of the treatment to the remaining number of undamaged cells at time t indicated by N(t) [Citation57]:
Ω can thus be expressed as a function of the thermal dose with the same fit coefficients a and b calculated above:
Evaluation of clinical treatment spot sizes
For sake of illustration, clinical data from both radiation surgery and thermal ablation have been processed. The only indication which the authors could get access to data sets for both is essential tremor. Thalamotomy data for both FUS and SRS were thus analysed for predicted cell survival. The clinical data used for analysis are anonymised data from a pilot study for essential tremor patients conducted at the University of Virginia [Citation58] under oversight and approval of the university’s Institutional Review Board (IRB). The anonymised Gamma Knife SRS dose distributions for the three cases used in the analysis have been determined by the IRB to be not subject to IRB review.
focused ultrasound
The clinical FUS study consisted of 15 patients with essential tremor whose condition did not improve with medication. The patients were treated with MR-guided FUS, targeting the ventral intermediate (VIM) nucleus of the thalamus. A series of low-power sonications were delivered to the intended target to validate the geometric accuracy of the set-up.
Temperature maps were recorded during treatment using MR thermometry [Citation59]. In this study, the temperature for each voxel was read from the raw data files for each timepoint of the sonication and used to calculate the thermal dose. The thermal dose profile was determined by normalising the data to the maximum thermal dose in the volume of interest.
Using the calculated thermal profiles and the cell survival-thermal dose relationship for all cell lines (CHO, CHL and GBA), cell survival was calculated for each voxel in the region of interest.
Gamma knife radiosurgery
The clinical radiosurgery data are derived from treatment plans for three radiosurgical thalamotomies performed on the Gamma Knife at University of Virginia for individuals with essential tremor that have failed to improve with other treatments and are unwilling, or unable, to undergo an invasive procedure. Radiosurgical thalamotomy was achieved using the Leksell Gamma Knife to deliver a maximum dose of 130–140 Gy with a 4 mm isocenter to the VIM nucleus on the opposite side of the brain from the more severe tremor. The technique employed at the University of Virginia is similar to techniques published in the literature [Citation60].
SRS dose distributions were created using the Gamma Knife treatment planning software (Leksell GammaPlan versions 8.0–10.1, Elekta AB, Stockholm). The resulting dose distributions were exported in anonymised DICOM-RT format. The radiotherapy dose profiles in x and y directions were averaged and then normalised to the maximum administered dose and converted to a percentage. Using the cell survival-radiotherapy dose relationship for CHO, CHL and GBA, the fraction of cells surviving was determined as a function of distance.
Results
Cell survival fitting
The resulting cell survival curves, extracted from previous studies, [Citation61–66] are shown in for radiotherapy and thermal dose.
Figure 2. Fraction of cells surviving as a function of the radiation (A) or thermal (B) dose for (•) CHO, (♦) CHL and (▪) GBA. Data have been extracted from previously published results: (CHO),[Citation61] (CHL),[Citation62] (GBA) [Citation65] (A) and (CHO), [Citation63] (CHL), [Citation66] (GBA) [Citation64] (B).
![Figure 2. Fraction of cells surviving as a function of the radiation (A) or thermal (B) dose for (•) CHO, (♦) CHL and (▪) GBA. Data have been extracted from previously published results: (CHO),[Citation61] (CHL),[Citation62] (GBA) [Citation65] (A) and (CHO), [Citation63] (CHL), [Citation66] (GBA) [Citation64] (B).](/cms/asset/e6dd2321-c10b-4cfd-8354-4d398c97f486/ihyt_a_1278281_f0002_c.jpg)
Results are summarised in . The α/β values for the radiation treatment on the 3 types of cells are close to previously published values [Citation54,Citation67,Citation68]. The linear quadratic fit of the curves resulted in an average coefficient of determination of R2 = 0.995, a maximum of 0.998, and a minimum of 0.992. All R2 values are displayed in . In order to achieve a cell survival fraction of 10 − 5 (i.e. a survival of 0.001%), a radiotherapy dose of 17 Gy must be applied to CHO cells, 20.5 Gy to CHL cells, and 14 Gy to GBA cells. To achieve the same percentage of surviving cells a thermal dose of 148.5CEM must be administered to the targeted CHO cells, 178CEM to CHL cells, and 249.5CEM to GBA cells.
Table 2. Parameters for the Universal Survival Curve (USC) for radiation therapy doses and linear quadratic exponential fit for thermal doses.
Estimated cell survival from clinical MRgFUS and Gamma Knife essential tremor treatments
The dose profiles from the clinical essential tremor data for FUS and SRS are shown in , normalised to the point of maximum delivered dose (3160 CEM for thermal and 130 Gy for SRS). About 10% of the maximum dose is delivered to cells at 1.6 mm for thermal dose and at 6.9 mm for radiotherapy exposures. As seen in the simulated profiles, the thermal dose profile drop off is sharp and by 3 mm the dose is at zero. For the radiotherapy dose, the drop off is much more gradual and reaches an average of 4% of the maximum dose at 11 mm.
Figure 3. Average dose profiles (A) and corresponding survival curves (B) for essential tremor clinical data. The average profiles amongst patients are shown in four directions from the centre and the average is highlighted in bold (A). Survival curves for essential tremor clinical data for FUS (solid) and SRS (dashed) are plotted for each cell type available.
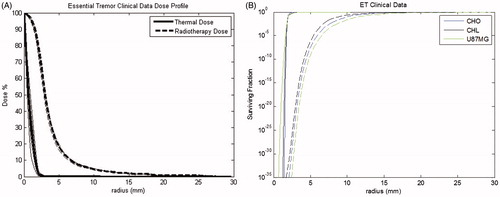
The corresponding simulated cell survival curves for the essential tremor clinical data are displayed in . As an example, for GBA cells, the survival curve for the radiotherapy dose clinical data reaches a level of 90% at 28.9 mm and 99.5% at 27.78 mm. For the thermal dose, 90% survival occurs at a distance of 3.71 mm and reaches 99.5% survival at a distance of 6.5 mm. The survival curve for radiotherapy dose drops off more gradually than the thermal dose survival curve ().
Discussion
Potential clinical applications of FUS overlap significantly with SRS. FUS is being considered as a substitute modality for radiotherapy for indications such as essential tremor, neuropathic pain and others. However, the techniques do not need to compete. A more common scenario may be to use FUS in combination with radiotherapy, especially in the treatment of malignant disease. In this setting, FUS may be used to debulk the main tumour mass, allowing for significant and immediate symptomatic relief. This may be followed by radiation to the surrounding tumour bed with the goal of reducing local failure and regional recurrences. Investigators are also looking at the reverse approach, where the tumour and the surrounding tumour bed are first irradiated to damage the ability of the cells to reproduce. This is then followed by FUS to debulk the main tumour.
In either situation, a method of quantifying the biological damage inflicted on both normal and diseased tissue from both modalities, like the one introduced in this paper, would be valuable, and in particular for areas of tissue receiving sub-lethal doses from either modality on its own.
Historically, creating a direct comparison between thermal dose and radiation dose has been considered impractical [Citation1]. This is in part because the concept of absorbed dose for ionising radiation describes the physics of the situation; i.e. the energy absorbed by a mass of tissue from exposure to ionising radiation [Citation43]. Conversely, the formulation of the thermal iso-effective dose is based on empirically observed effects in heated cells.
However, SRS [Citation19] and FUS surgery [Citation16,Citation69] attempt to achieve a similar biological effect: the ablation of a volume of tissue. SRS, especially when delivered in a single fraction, delivers a dose to tissue that can be considered “ablative” in the sense that the expected surviving fraction of cells is low [Citation70]. Likewise, FUS achieves ablation of a desired region of tissue by heating to a thermal isoeffect dose known to cause coagulative necrosis with a similarly negligible surviving cell fraction [Citation71,Citation72]. A threshold for damage of 240CEM was determined in vivo in dog prostate [Citation73] and in muscle [Citation74] and corresponds to the threshold used in most clinical systems [Citation75]. Normalisation to a similar biological endpoint makes feasible a direct comparison of radiation dose and thermal isoeffect. The threshold thermal or radiation dose required to “just” achieve ablation gives us a sort of “calibration” point in the spectrum of biological damage at which to equate the two dose formulations.
Equating the dose fomulations might benefit the planning of combination therapies which take advantage of the synergistic effect of radiotherapy and thermal ablation. Compared to RF ablation alone, RF ablation combined with radiation therapy has been shown to increase the ablation volume in rat tumours [Citation76] and improve survival [Citation77], has shown a low rate of complications in patients with unresectable lung cancer [Citation78–80] and has increased the relapse-free survival rate in prostate adenocarcinoma [Citation81]. This pioneering work could be revisited in an optimal way by taking advantage of the non-invasive and conformal treatment capabilities of focused ultrasound and external beam therapy for inducing thermal and radiation effects with 3D planning based on the cell survival formulation proposed here.
The thermal energy resulting in cytotoxicity drops off faster than for radiation therapy (). In part, this is related to the physics and technical details of the devices. Gamma Knife, used as a platform for the radiosurgery dose distibutions in our study, achieves a steep dose profile through the application of a large number (192 in the Perfexion model Gamma Knife used in this study) cross-firing beams that are collimated so they intersect with high precision at a focal point. A single irradiation location or “isocenter” creates an approximately spherical dose distribution. Irradiation of irregularly shaped targets is achieved by using multiple isocenters and collimator settings at different positions. The dose falloff on a Gamma Knife is fundamentally dependent on the attenuation of each beam (which is at 60Co energy), the penumbra of each beam and the total number of beams, and the isodose level in the distribution placed at the treatment margin of the target [Citation82]. The sharper spatial distribution falloff of the thermal dose is linked to the size of each individual heated region (focal spot) and thus to the ultrasonic transducer geometry, and the frequency used. This is not unexpected as thermal conduction is a very inefficient method of heating tissue [Citation83]: thermal conduction is an inherent limitation for large treatment volumes with focused ultrasound as the focal spot is small. The whole volume needs to be treated spot by spot. Nevertheless, limited diffusion can also be seen as an advantage as it contributes to a sharper boundary and a better control of energy deposition.
Limitations of this study
The intent of this paper was to explore the potential utility of equating thermal dose and radiation dose effects using cell survival as a common unit of measurement. As there is limited data collected with the intent of making such a direct comparison, there are assumptions that impact the uncertainties in the analysis including the in vitro cell survival data used in the study, the accuracy of survival models at ablative doses and simplification of the possible complexities of the joint biological effects of thermal energy and ionising radiation.
Limitations of in vitro cell survival data in this study
Perhaps the most significant limitation of our experiment is that in vitro studies do not represent clinical reality. By design, in vitro experiments use cells grown under carefully controlled conditions. The in vivo situation is obviously much more complex; for example, our models do not take into account the heat sink effect of the surrounding tissue vasculature [Citation84], cell-cycle differences [Citation85] or tissue inhomogenities which can distort thermal and radiation dose [Citation86–88]. Stochastic models have shown that time-temperature history, tumour geometry, tumour perfusion and uniformity of heating are all likely to affect cell survival rates [Citation89]. Nor do in vitro models account for immunological effects, inflammatory effects, chemokines or cytokines that are present in vivo [Citation90,Citation91]. In addition, the cell lines used to create the thermal dose and radiation dose survival curves in this paper do not correspond with the cell types (i.e. normal brain tissue) irradiated during a FUS thalamotomy or radiosurgery thalamotomy. This was because of the non-existence of published data for our chosen clinical example. The mismatch between in vitro cell lines and the cells found in human brain tissue creates uncertainties on the specific parameters determined for our model fit. As in vitro and in vivo cell survival data are accrued for thermal and radiation doses over a larger range of cell-types, the method presented in this paper can be refined to include better parameter estimates.
Limitations of survival models at ablative doses
Another limitation to our approach is that the mathematical models used for thermal and radiation dose derive from in vitro cell survival data acquired at sub-ablative doses. Both the thermal dose and ionising radiation cell survival models have been shown to have weaknesses at high, single-fraction doses. Ideally, experiments should be conducted on the same types of cells, with the same cell survival assay and for SRS-like doses. Multiple models for thermal damage and radiation damage could also be employed to better represent the likely uncertainty in the calculations [Citation92]. This is beyond the scope of the present paper.
Simplifying assumptions regarding the joint biological effects of thermal energy and ionising radiation
A simplifying assumption of the study is that thermal dose and radiation dose may be treated independently. In practice, there is likely some degree of interaction between the two. For instance, hyperthermia studies have demonstrated in vitro that the achievable radiosensitisation caused by heating is dependent on the sequence and time interval between heating and irradiation. While data remain inconclusive, there is a consensus that simultaneous heating and irradiation would maximise the dose enhancement effect due to heat, and as the interval between heat and radiation increases (regardless of sequence) the degree of enhancement decreases [Citation26]. Radiosensitisation may also be enhanced through heating by preferentially killing chronically hypoxic tissue as is often found in the centre of malignant tumours as they grow. These hypoxic regions tend to be radioresistant, and it is unclear how using heat to destroy these regions would effect the overall sensitivity profile to a joint treatment. A related effect is that heating can cause increased perfusion to targeted tissue, helping to reoxygenate (and thus radiosensitise) hypoxic areas of tissue [Citation93,Citation94].
This study attempts to relate thermal dose and radiation dose at a very low “ablative” level of cell kill, perhaps avoiding some of the complexities of the interactions between the biology of thermal and radiation insults. The thermal dose profiles presented in the results include a thin region of sub-lethally heated tissue. This tissue likely benefits from some of the radiosensitisation that has been demonstrated from these earlier hyperthermia results, and this is not accounted for in our model. However further investigation will be required to understand what, if any, clinical significance these sub-lethally heated regions would have on the ultimate outcome of a joint thermal/radiation treatment and how it might affect thermal or radiation dose prescriptions.
Other limitations of the work
Finally, different tissue and organ types have differential sensitivity to both ionising and thermal dose. This is also not modelled in the current approach, however it is inherent in the in vitro cell data used in the study. Differential response to ionising radiation is partly related to the length of the cell-cycle of a given cell type, as lethal DNA damage is manifested during mitosis. Cells with short lifespans tend to show early reaction to radiation insult. Slower-dividing cells tend to show later reactions [Citation1]. The primary mechanism of thermal cell death is a combination of protein denaturation and coagulative necrosis. This could reasonably be assumed to be similar across all cell lines. The differences then may be due to alternative mechanism of cell death such as disruption of intracellular signal transduction and cell motility [Citation42,Citation95,Citation96].
Conclusions
Radiation- and thermal-based treatments are difficult to compare because of the differences in the physics of the energy deposition and the resulting biological effects. The work presented in this paper is a first attempt at creating a method of equating thermal and radiation doses for ablative treatments by considering “ablation” as a common biological endpoint. It was shown how radiation dose and thermal dose could both be expressed in terms of percentage of surviving cells, and thus be compared with the same quantitative effect on tissues. Much work remains to be done in order to validate the regression equations and dose models against both in vitro and in vivo targets of different heterogeneous tissues.
Disclosure statement
Neal Kassell is an InSightec shareholder. The authors alone are responsible for the content and writing of the article.
References
- Hall EJ, Giaccia AJ. (2006). Radiobiology for the radiologist Philadelphia (PA): Lippincott Williams&Wilki.
- Horsman MR, Overgaard J. (2007). Hyperthermia: a potent enhancer of radiotherapy. Clin Oncol (R Coll Radiol) 19:418–26.
- Lederman M. (1981). The early history of radiotherapy: 1895-1939. Int J Radiat Oncol Biol Phys 7:639–48.
- Haemmerich D. (2010). Biophysics of radiofrequency ablation. Crit Rev Biomed Eng 38:53.
- Carrafiello G, Ierardi AM, Fontana F, et al. (2013). Microwave ablation of pancreatic head cancer: safety and efficacy. J Vasc Interv Radiol 24:1513–20.
- Chen JC, Moriarty JA, Derbyshire JA, et al. (2000). Prostate cancer: MR imaging and thermometry during microwave thermal ablation-initial experience. Radiology 214:290–7.
- Healey TT, Dupuy DE. (2012). Microwave ablation for lung cancer. Med Health R 95:52–3.
- Kuang M, Lu MD, Xie XY, et al. (2007). Liver cancer: increased microwave delivery to ablation zone with cooled-shaft antenna–experimental and clinical studies. Radiology 242:914–24.
- Skinner MG, Iizuka MN, Kolios MC, et al. (1998). A theoretical comparison of energy sources - microwave, ultrasound and laser - for interstitial thermal therapy. Phys Med Biol 43:3535.
- Vargas HI, Dooley WC, Gardner RA, et al. (2004). Focused microwave phased array thermotherapy for ablation of early-stage breast cancer: results of thermal dose escalation. Ann Surg Oncol 11:139–46.
- Yoshitani S, Hayashi K, Kuroda M, et al. (2005). [Results of local ablation therapy for liver metastases from colorectal cancer using radiofrequency ablation and microwave coagulation therapy (RFA/MCT)]. Gan to Kagaku Ryoho 32:1666–9.
- Zhang X, Chen B, Hu S, et al. (2008). Microwave ablation with cooled-tip electrode for liver cancer: an analysis of 160 cases. Hepatogastroenterology 55:2184–7.
- Ali MA, Carroll KT, Rennert RC, et al. (2016). Stereotactic laser ablation as treatment for brain metastases that recur after stereotactic radiosurgery: a multiinstitutional experience. Neurosurg Focus 41:E11. PubMed PMID: 27690654.
- Latorre M, Rinaldi C. (2009). Applications of magnetic nanoparticles in medicine: magnetic fluid hyperthermia. P R Health Sci J 28:227–38.
- Tseng HY, Lee GB, Lee CY, et al. (2009). Localised heating of tumours utilising injectable magnetic nanoparticles for hyperthermia cancer therapy. IET Nanobiotechnol 3:46–54.
- Hynynen K, Darkazanli A, Unger E, et al. (1993). MRI-guided non-invasive ultrasound surgery. Med Phy 20:107–15.
- Ebbini ES, Ter Haar G. (2015). Ultrasound-guided therapeutic focused ultrasound: current status and future directions. Int J Hyperthermia 31:77–89.
- Maloney E, Hwang JH. (2015). Emerging HIFU applications in cancer therapy. Int J Hyperthermia 31:302–9.
- Leksell L. (1951). The stereotaxic method and radiosurgery of the brain. Acta Chir Scand 102:316–19.
- Blomgren H, Lax I, Naslund I, et al. (1995). Stereotactic high dose fraction radiation therapy of extracranial tumors using an accelerator. Clinical experience of the first thirty-one patients. Acta Oncol 34:861–70.
- Papiez L, Timmerman R, DesRosiers C, et al. (2003). Extracranial stereotactic radioablation: physical principles. Acta Oncol 42:882–94.
- Timmerman R, Papiez L, McGarry R, et al. (2003). Extracranial stereotactic radioablation: results of a phase I study in medically inoperable stage I non-small cell lung cancer. Chest 124:1946–55.
- Dieterich S, Ford E, Pavord D, et al. (2015). Practical radiation oncology physics: a companion to gunderson and tepper's clinical radiation oncology. Philadelphia(PA): Elsevier - Health Sciences Division.
- Gunderson LL, Willett CG, Calvo FA, et al. (2011). Intraoperative irradiation: techniques and results: New York (NY): Humana Press.
- Dewey WC, Holahan EV. (1984). Hyperthermia-basic biology. Prog Exp Tumor Res 28:198–219.
- Overgaard J. (1982). Influence of sequence and interval on the biological response to combined hyperthermia and radiation. Natl Cancer Inst Monogr 61:325–32.
- Kosterev VV, Kramer-Ageev EA, Mazokhin VN, et al. (2015). Development of a novel method to enhance the therapeutic effect on tumours by simultaneous action of radiation and heating. Int J Hyperthermia 31:443–52.
- Gillette EL. (1984). Clinical use of thermal enhancement and therapeutic gain for hyperthermia combined with radiation or drugs. Cancer Res 44:4836s–41s.
- Dewhirst MW, Sim DA. (1984). The utility of thermal dose as a predictor of tumor and normal tissue responses to combined radiation and hyperthermia. Cancer Res 44:4772s–80s.
- Overgaard J. (1984). Formula to estimate the thermal enhancement ratio of a single simultaneous hyperthermia and radiation treatment. Acta Radiol Oncol 23:135–9.
- Valdagni R, Amichetti M. (1994). Report of long-term follow-up in a randomized trial comparing radiation therapy and radiation therapy plus hyperthermia to metastatic lymph nodes in stage IV head and neck patients. Int J Radiat Oncol Biol Phys 28:163–9.
- Huilgol NG, Gupta S, Sridhar CR. (2010). Hyperthermia with radiation in the treatment of locally advanced head and neck cancer: a report of randomized trial. J Cancer Res Ther 6:492–6.
- Overgaard J, Gonzalez Gonzalez D, Hulshof MC, et al. (1996). Hyperthermia as an adjuvant to radiation therapy of recurrent or metastatic malignant melanoma. A multicentre randomized trial by the European Society for Hyperthermic Oncology. Int J Hyperthermia 12:3–20.
- Vernon CC, Hand JW, Field SB, et al. (1996). Radiotherapy with or without hyperthermia in the treatment of superficial localized breast cancer: results from five randomized controlled trials. Int J Radiat Oncol Biol Phys 35:731–44.
- Sneed PK, Stauffer PR, McDermott MW, et al. (1998). Survival benefit of hyperthermia in a prospective randomized trial of brachytherapy boost +/- hyperthermia for glioblastoma multiforme. Int J Radiat Oncol Biol Phys 40:287–95.
- van der Zee J, González D, van Rhoon GC, et al. (2000). Comparison of radiotherapy alone with radiotherapy plus hyperthermia in locally advanced pelvic tumours: a prospective, randomised, multicentre trial. Lancet 355:1119–25.
- Harima Y, Nagata K, Harima K, et al. (2001). A randomized clinical trial of radiation therapy versus thermoradiotherapy in stage IIIB cervical carcinoma. Int J Hyperthermia 17:97–105.
- Jones EL, Oleson JR, Prosnitz LR, et al. (2005). Randomized trial of hyperthermia and radiation for superficial tumors. J Clin Oncol 23:3079–85.
- Vasanthan A, Mitsumori M, Park JH, et al. (2005). Regional hyperthermia combined with radiotherapy for uterine cervical cancers: a multi-institutional prospective randomized trial of the international atomic energy agency. Int J Radiat Oncol Biol Phys 61:145–53.
- Mitsumori M, Zeng ZF, Oliynychenko P, et al. (2007). Regional hyperthermia combined with radiotherapy for locally advanced non-small cell lung cancers: a multi-institutional prospective randomized trial of the International Atomic Energy Agency. Int J Clin Oncol 12:192–8.
- Schroeder C, Gani C, Lamprecht U, et al. (2012). Pathological complete response and sphincter-sparing surgery after neoadjuvant radiochemotherapy with regional hyperthermia for locally advanced rectal cancer compared with radiochemotherapy alone. Int J Hyperthermia 28:707–14.
- Mallory M, Gogineni E, Jones GC, et al. (2016). Therapeutic hyperthermia: the old, the new, and the upcoming. Crit Rev Oncol Hematol 97:56–64.
- Khan FM. (2014). The physics of radiation therapy. 5th ed. Philadelphia (PA): Wolters Kluwer Health.
- Kirkpatrick JP, Meyer JJ, Marks LB. (2008). The linear-quadratic model is inappropriate to model high dose per fraction effects in radiosurgery. Semin Radiat Oncol 18:240–3.
- Lepock JR. (2005). How do cells respond to their thermal environment? Int J Hyperthermia 21:681–7.
- Mouratidis PX, Rivens I, ter Haar G. (2015). A study of thermal dose-induced autophagy, apoptosis and necroptosis in colon cancer cells. Int J Hyperthermia 31:476–88.
- Sapareto SA, Dewey WC. (1984). Thermal dose determination in cancer therapy. Int J Radiat Oncol Biol Phys 10:787–800.
- Park C, Papiez L, Zhang S, et al. (2008). Universal survival curve and single fraction equivalent dose: useful tools in understanding potency of ablative radiotherapy. Int J Radiat Oncol Biol Phys 70:847–52.
- Kavanagh BD, Newman F. (2008). Toward a unified survival curve: in regard to Park et al. (Int J Radiat Oncol Biol Phys 2008;70:847–852) and Krueger et al. (Int J Radiat Oncol Biol Phys 2007;69:1262–1271). Int J Radiat Oncol Biol Phys 71:958–9.
- Wang JZ, Huang Z, Lo SS, et al. (2010). A generalized linear-quadratic model for radiosurgery, stereotactic body radiation therapy, and high–dose rate brachytherapy. Sci Transl Med 2:39ra48.
- Hanin LG, Zaider M. (2010). Cell-survival probability at large doses: an alternative to the linear-quadratic model. Phys Med Biol 55:4687–702.
- Astrahan M. (2008). Some implications of linear-quadratic-linear radiation dose-response with regard to hypofractionation. Med Phys 35:4161.
- Guerrero M, Li XA. (2004). Extending the linear–quadratic model for large fraction doses pertinent to stereotactic radiotherapy. Phys Med Biol 49:4825–35.
- Garcia LM, Wilkins DE, Raaphorst GP. (2007). Alpha/beta ratio: a dose range dependence study. Int. J. Radiat. Oncol. Biol. Phys 67:587.
- McDannold N, Zhang Y-Z, Power C, et al. (2013). Nonthermal ablation with microbubble-enhanced focused ultrasound close to the optic tract without affecting nerve function: laboratory investigation. J Neurosurg 119:1208–20.
- Carlson DJ, Stewart RD, Semenenko VA, et al. (2009). Combined use of Monte Carlo DNA damage simulations and deterministic repair models to examine putative mechanisms of cell killing. Radiat Res 169;447–59.
- Viglianti BL, Dewhirst MW, Abraham JP, et al. (2014). Rationalization of thermal injury quantification methods: application to skin burns. Burns 40:896–902.
- Elias WJ, Huss D, Voss T, et al. (2013). A pilot study of focused ultrasound thalamotomy for essential tremor. N Engl J Med 369:640–8.
- Rieke V, Butts Pauly K. (2008). MR thermometry. J Magn Reson Imag 27:376–90.
- Kondziolka D, Ong JG, Lee JY, et al. (2008). Gamma Knife thalamotomy for essential tremor. J Neurosurg 108;111–17.
- Dewey W, Hopwood L, Sapareto S, et al. (1977). Cellular responses to combinations of hyperthermia and radiation. Radiology 123:463–74.
- Raaphorst GP, Szekely J, Lobreau A, et al. (1983). A comparison of cell killing by heat and/or X rays in Chinese hamster V79 cells, Friend erythroleukemia mouse cells, and human thymocyte MOLT-4 cells. Radiat Res 94:340–9.
- Flentje M, Flentje D, Sapareto SA. (1984). Differential effect of hyperthermia on murine bone marrow normal colony-forming units and AKR and L1210 leukemia stem cells. Cancer Res 44:1761–6.
- Armour EP, McEachern D, Wang Z, et al. (1993). Sensitivity of human cells to mild hyperthermia. Cancer Res 53:2740–4.
- Raaphorst GP, Feeley MM. (1994). Hyperthermia radiosensitization in human glioma cells comparison of recovery of polymerase activity, survival, and potentially lethal damage repair. Int J Radiat Oncol Biol Phys 29:133–9.
- Lepock JR. (2003). Cellular effects of hyperthermia: relevance to the minimum dose for thermal damage. Int J Hyperthermia 19:252.
- Scheenstra AEH, Rossi MMG, Belderbos JSA, et al. (2014). Alpha/beta ratio for normal lung tissue as estimated from lung cancer patients treated with stereotactic body and conventionally fractionated radiation therapy. Int J Radiat Oncol Biol Phys 88:224.
- Williams MV, Denekamp J, Fowler JF. (1985). A review of alpha/beta ratios for experimental tumors: implications for clinical studies of altered fractionation. Int J Radiat Oncol Biol Phys 11:87.
- Hynynen K, Colucci V, Chung A, et al. (1996). Noninvasive arterial occlusion using MRI-guided focused ultrasound. Ultrasound Med Biol 22:1071–7.
- Abbas G, Schuchert MJ, Pennathur A, et al. (2007). Ablative treatments for lung tumors: radiofrequency ablation, stereotactic radiosurgery, and microwave ablation. Thorac Surg Clin 17:261–71.
- Dewhirst MW, Lora-Michiels M, Viglianti BL, et al. (2003). Carcinogenic effects of hyperthermia. Int J Hyperthermia 19:236–51.
- Fry W, Mosberg W, Barnard J, Fry F. (1954). Production of focal destructive lesions in the central nervous system with ultrasound. J Neurosurg 11:471–8.
- Yarmolenko PS, Moon EJ, Landon C, et al. (2011). Thresholds for thermal damage to normal tissues: an update. Int J Hyperthermia 27:320–43.
- Dewey W. (1994). Arrhenius relationships from the molecule and cell to the clinic. Int J Hyperthermia 10:457–83.
- Foley JL, Eames M, Snell J, et al. (2013). Image-guided focused ultrasound: state of the technology and the challenges that lie ahead. Imaging Med 5:357–70.
- Solazzo S, Mertyna P, Peddi H, et al. (2008). RF ablation with adjuvant therapy: comparison of external beam radiation and liposomal doxorubicin on ablation efficacy in an animal tumor model. Int J Hyperthermia 24:560–7.
- Horkan C, Dalal K, Coderre JA, et al. (2005). Reduced tumor growth with combined radiofrequency ablation and radiation therapy in a rat breast tumor model 1. Radiology 235:81–8.
- Grieco CA, Simon CJ, Mayo-Smith WW, et al. (2006). Percutaneous image-guided thermal ablation and radiation therapy: outcomes of combined treatment for 41 patients with inoperable stage I/II non–small-cell lung cancer. J Vasc Interv Radiol 17:1117–24.
- Chan MD, Dupuy DE, Mayo-Smith WW, et al (2011). Combined radiofrequency ablation and high-dose rate brachytherapy for early-stage non-small-cell lung cancer . Brachytherapy 10:253–9.
- Imada H, Nomoto S, Tomimatsu A, et al. (1999). Local control of nonsmall cell lung cancer by radiotherapy combined with high power hyperthermia using an 8 MHz RF capacitive heating device. Jpn J Hyperthermic Oncol 15:19–24.
- Anscher MS, Samulski TV, Dodge R, et al. (1997). Combined external beam irradiation and external regional hyperthermia for locally advanced adenocarcinoma of the prostate. Int J Radiat Oncol Biol Phys 37:1059–65.
- Lindquist C, Paddick I. (2007). The Leksell Gamma Knife Perfexion and comparisons with its predecessors. Neurosurgery 61:130–40.
- Livraghi T, Mueller PR, Silverman SG. (2008). Tumor ablation: principles and practice. In: van Sonnenberg E, McMullen W, Solbiati L, eds. New York (NY): Springer Science and Business Media.
- Lagendijk J, Schellekens M, Schipper J, et al. (1984). A three-dimensional description of heating patterns in vascularised tissues during hyperthermic treatment. Phys Med Biol 29:495.
- Barranco SC, Romsdahl MM, Humphrey RM. (1971). The radiation response of human malignant melanoma cells grown in vitro. Cancer Res 31:830–3.
- Aubry JF, Tanter M, Pernot M, et al. (2003). Experimental demonstration of noninvasive transskull adaptive focusing based on prior computed tomography scans. J Acoust Soc Am 113:84–93.
- Clement G, Hynynen K. (2002). A non-invasive method for focusing ultrasound through the human skull. Phys Med Biol 47:1219–36.
- Moskvin V, Timmerman R, Fau - DesRosiers C, et al. (2004). Monte carlo simulation of the Leksell Gamma Knife: II. Effects of heterogeneous versus homogeneous media for stereotactic radiosurgery. Phys Med Biol 49:4879–95.
- Rosner GL, Clegg ST, Prescott DM, et al. (1996). Estimation of cell survival in tumours heated to nonuniform temperature distributions. Int J Hyperthermia 12:223–39.
- Hong ZY, Song KH, Yoon JH, et al. (2015). An experimental model-based exploration of cytokines in ablative radiation-induced lung injury in vivo and in vitro. Lung 193:409–19.
- Kahn J, Tofilon PJ, Camphausen K. (2012). Preclinical models in radiation oncology. Radiat Oncol 7:223.
- Pearce JA. (2013). Comparative analysis of mathematical models of cell death and thermal damage processes. Int J Hyperthermia 29:262–80.
- Griffin RJ, Dings RP, Jamshidi-Parsian A, et al. (2010). Mild temperature hyperthermia and radiation therapy: role of tumour vascular thermotolerance and relevant physiological factors. Int J Hyperthermia 26:256–63.
- Song CW, Shakil A, Griffin RJ, et al. (1997). Improvement of tumor oxygenation status by mild temperature hyperthermia alone or in combination with carbogen. Semin Oncol 24:626–32.
- Liang P, MacRae TH. (1997). Molecular chaperones and the cytoskeleton. J Cell Sci 110:1431–40.
- Pratt WB, Silverstein AM, Galigniana MD. (1999). A model for the cytoplasmic trafficking of signalling proteins involving the hsp90-binding immunophilins and p50cdc37. Cellular Signal 11:839–51.
- Ling CC, Robinson E. (1988). Moderate hyperthermia and low dose rate irradiation. Radiat Res 114:379–84.
- Sapareto SA, Hopwood LE, Dewey WC, Raju MR, Gray JW. (1978). Effects of hyperthermia on survival and progression of Chinese hamster ovary cells. Cancer Res 38:393.