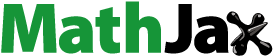
Abstract
Background
As magnetic resonance imaging (MRI) becomes increasingly integrated into radiotherapy (RT) for enhanced treatment planning and adaptation, the inherent geometric distortion in acquired MR images pose a potential challenge to treatment accuracy. This study aimed to evaluate the geometric distortion levels in the clinical MRI protocols used across Danish RT centers and discuss influence of specific sequence parameters. Based on the variety in geometric performance across centers, we assess if harmonization of MRI sequences is a relevant measure.
Materials and methods
Nine centers participated with 12 MRI scanners and MRI-Linacs (MRL). Using a travelling phantom approach, a reference MRI sequence was used to assess variation in baseline distortion level between scanners. The phantom was also scanned with local clinical MRI sequences for brain, head/neck (H/N), abdomen, and pelvis. The influence of echo time, receiver bandwidth, image weighting, and 2D/3D acquisition was investigated.
Results
We found a large variation in geometric accuracy across 93 clinical sequences examined, exceeding the baseline variation found between MRI scanners (σ = 0.22 mm), except for abdominal sequences where the variation was lower. Brain and abdominal sequences showed lowest distortion levels ([0.22, 2.26] mm), and a large variation in performance was found for H/N and pelvic sequences ([0.19, 4.07] mm). Post hoc analyses revealed that distortion levels decreased with increasing bandwidth and a less clear increase in distortion levels with increasing echo time. 3D MRI sequences had lower distortion levels than 2D (median of 1.10 and 2.10 mm, respectively), and in DWI sequences, the echo-planar imaging read-out resulted in highest distortion levels.
Conclusion
There is a large variation in the geometric distortion levels of clinical MRI sequences across Danish RT centers, and between anatomical sites. The large variation observed makes harmonization of MRI sequences across institutions and adoption of practices from well-performing anatomical sites, a relevant measure within RT.
Background
The role of magnetic resonance imaging (MRI) in radiotherapy (RT) is rapidly expanding with the introduction of MRI-only [Citation1,Citation2] and integrated MRI-Linac (MRL) workflows [Citation3,Citation4]. These have several benefits, including better soft-tissue conspicuity allowing direct visualization of the tumor/normal tissue in (daily) treatment adaptation, online-gated treatments based on MRI-cinematic imaging, and acquisition of functional information for research, all without exposing the patient to imaging related ionizing radiation.
RT aims for precise treatment delivery by minimizing margins to spare healthy tissue without compromising local control. Therefore, the geometric accuracy of images used for planning and adapting RT is critical. Unfortunately, MRI is known to be less geometrically accurate compared to CT [Citation5]. Geometric distortions are caused by MRI scanner-specific effects, such as gradient non-linearity, imperfectly shimmed main magnetic field, and eddy currents, and patient-specific effects such as magnetic susceptibility differences in tissues and chemical shifts of chemical compounds [Citation6].
MRI sequence settings influence the level of geometric distortion caused by these effects [Citation7,Citation8]. In general, the distortion level scale with the magnetic susceptibility differences, main field strength, and scales inversely with the receiver bandwidth (BW) [Citation7,Citation8]. Moreover, gradient recalled echo (GRE) and echo-planar imaging (EPI) sequences are more prone to these artifacts than spin-echo (SE) sequences, as spins are not realigned by a refocusing radiofrequency (RF) pulse in GRE, and due to the low effective receiver BW in EPI [Citation9,Citation10].
Actual geometric distortions have previously been shown to be clinically relevant in RT, with levels up to 4 mm in the brain [Citation11], and especially for non-centrally located targets such as liver metastases or breast, as geometric distortions generally increases with increasing distance to the MRI isocenter as magnetic gradient linearity becomes increasingly difficult to achieve. Several reports address tolerance levels to secure image quality and patient safety [Citation6,Citation10,Citation12–14]. General tolerance levels for geometric uncertainties in RT and MRI simulation below 2 mm is recommended [Citation10,Citation12] and suggested to be lowered for MRI-guided RT to <1 mm close to target regions, as distortions can propagate directly into dosimetric errors [Citation6]. A higher off-axis geometric accuracy is also important in MRL workflows, where the lateral motion of the couch is not possible [Citation3,Citation15,Citation16] or limited [Citation17] on current MRL systems.
MRI sequences used in RT are typically based on local preferences (maybe adopted from radiology), guided by national or international guidelines for anatomical targets [Citation18–22]. This results in a vast catalog of MRI sequences used across institutions. The lack of harmonization of sequences could potentially lead to large discrepancies in MRI quality, affecting treatment quality between centers. MRI accreditation programs may mitigate this, but so far exist only in radiology, where the focus is on high sensitivity rather than geometrical fidelity [Citation23,Citation24].
Previous studies of RT-specific MRI sequences have investigated the overall image quality, contrast, or geometric distortion for a limited number of sequences in single anatomies [Citation25–28]. The current study evaluated the geometric accuracy of target specific MRI protocols used across all RT centers in Denmark. The aim was to clarify if differences in geometric quality of MRI sequences across RT centers and anatomical regions exist, and to investigate if there is a clinically relevant potential for harmonization. Apart from determining the geometric distortion, the specific objectives were to investigate the influence of common MRI sequence parameters on the distortion levels and discuss the findings in a clinically relevant context.
Methods
Eight RT and one radiological center, all collaborators in the National Interdisciplinary Network for Quality Assurance of MR Images in Radiotherapy (NIMBUS) [Citation29], participated in this study, resulting in 12 MRI scanners included. Of those, three were MRLs, eight were designated RT MRI, and one was a diagnostic MRI scanner (). The diagnostic MRI scanner was included for comparison, as RT-MRI and diagnostic MRI have a different focus on geometric accuracy. The study was conducted with a travelling phantom approach, where the same team visited each center with the same phantom.
Table 1. MRI/MRL scanners and hardware specifications.
Phantom
Geometric distortions were measured using a large third party phantom (Magphan® RT 820, The Phantom Laboratory, Salem, NY, USA). The phantom measured 35 × 27 × 21 cm3 and contained 513 spherical markers with a diameter of 1 cm. The phantom was filled with a background solution with T1 and T2 values in the range of 175–225 ms at 0.35 T, 350–400 ms at 1.5 T, and 500–550 ms at 3.0 T.[Citation30]
The phantom consisted of two individual modules, which were assembled at each center introducing a small variation in the relative positioning of the two modules (see Section ‘Distortion analysis tool’).
MRI acquisitions
MRI sequences
Each center selected clinical MRI protocols for two anatomical sites of their own choice (sequence parameters in Table S2, supplementary materials). The phantom was imaged with these sequences without any modifications to precisely replicate a clinical setup, and consisted of both 2D (axial, sagittal, and coronal) and 3D acquisitions.
To estimate the baseline variation in distortion levels between scanners, a reference 3D T1w GRE sequence was used in accordance with the literature [Citation31–33]. The reference sequences were adjusted to account for field strength differences (0.35, 1.5, 3 T) and vendor-specific restrictions (Table S1). The phantom was scanned with the reference sequence just prior to the clinical MRI sequences.
Influence of MRI sequence parameters
The influence of sequence parameters settings on distortion levels was studied retrospectively using DICOM [Citation34] header information for the individual clinical sequences. Specifically, the influence of receiver bandwidth per pixel (pBW), echo time (TE), image weighting (T1w, T2w, DWI), and 2D vs. 3D acquisition was investigated. The pBW was normalized to field strength (B0) to see the field independent effect. For EPI sequences, the effective pBW in the phase-encoding direction was calculated using (where
is the echo spacing and ETL is the echo train length), instead of the BW in frequency-encoding direction, as magnetic field inhomogeneity artifacts are seen predominantly here [Citation35,Citation36]. Theil-Sen robust linear regression [Citation37] was used to investigate the influence of sequence parameters on distortion level to limit the sensitivity to outliers. Differences in median distortion level between T1w/T2w and 2D/3D MRI sequences were tested using a Wilcoxon rank sum test (α = 5%)
Geometric distortion analysis
Distortion analysis tool
Distortion analysis of the MR images was performed with an in-house developed tool using MATLAB (MATLAB R2022b, The Mathworks Inc., Natick, MA, USA). Overall the tool was based on two steps: In step one, the spherical markers in the phantom in the MR images were localized using Spherical Hough transformation [Citation38]. In step two, the positions of the spherical markers were compared to reference positions found in CT images.
Rigid transformation of the positions of central spherical markers in the CT reference dataset (details in Section ‘Reference position dataset’) to the position in the MR images was used to account for phantom positioning differences. The spherical markers nearest the MRI isocenter considered the least affected by geometric distortion, were used for this [Citation39]. To account for phantom module assembly uncertainty, the positions of the spherical markers in the two modules of the phantom were transformed individually. The Euclidian distance between MRI and reference positions of all the identified spherical markers was reported as the magnitude distortion.
The distortion analysis tool was compared to commercially available software (Smári, The Phantom Laboratory, Salem, NY, USA) using the reference MRI sequence. This commercial software, however, required scans with large FOV and was therefore not compatible with most of the clinical MRI sequences.
Reference position dataset
A reference position dataset of the phantom was generated from CT scans (Siemens SOMATOM go.Open Pro, Siemens Healthcare, Erlangen, Germany), acquired at 130 kV, 432 mA, FOV of 492 × 492 × 268 mm3, and an exposure time of 1250 ms.
The reference position dataset was generated from five CT scans, where the phantom was reassembled before each scan. A rigid transformation was performed on the CT scans to account for phantom positioning. To include the reassembly uncertainty, the median position was found for each marker in the phantom as the reference position dataset.
Reported distortions
Distortions were reported as the 95th percentile maximum distortion (limiting outliers) within spherical volumes with diameters of 10 cm (DSV10), 20 cm (DSV20), 30 cm (DSV30), and with a spherical volume with the maximum diameter possible within the FOV (DSVmax, [30, 35, 40] cm for brain, H/N, abdomen, pelvis, and the reference sequence, respectively).
Results
MRI acquisitions resulted in data from 12 MRI scanners. The entire data set from one scanner (MRI8) was corrupted and could not be analyzed (). Furthermore, baseline data acquired with the reference sequence from another scanner (MRI5) was excluded due to presumed faulty setup of the sequence parameters. This resulted in baseline geometric distortion data from 10 scanners and distortion data on 93 clinical sequences from 11 scanners (sequence parameters listed in Table S2). The clinical MRI protocols included brain (n = 15), head and neck (H/N, n = 21), liver and abdomen (abdomen, n = 20), prostate and rectum (pelvis, n = 37).
A comparison of the in-house developed analysis tool and the commercially available software showed in general lower distortion level for the commercial software based on the reference sequence data from four scanners. The maximum distortion differed with around 0.6 mm, but the same overall tendency was seen (Figure S3).
Geometric distortion of clinical MRI sequences
The baseline geometric distortion levels were in general low, and the standard deviation (σ) for all scanners ranged between 0.21 mm and 0.27 mm when evaluated at different DSVs, describing the smallest observable difference between scanners (). Baseline distortion levels were comparable between 1.5 T and 3 T scanners, and higher for the 0.35 T scanners. The diagnostic MRI scanner were comparable with the other 3 T MRI scanners.
Figure 1. Baseline geometric distortions. The 95th percentile maximum distortion within a spherical volume with a diameter of 10 cm (DSV10, blue), 20 cm (DSV20, red), 30 cm (DSV30, yellow), and 40 cm (DSV40, purple) around MRI isocenter are shown. Scanners are grouped in field strength. σ denotes the standard deviation across all scanners.

The minimum distortion levels of clinical MRI sequences (excluding DWI) were below 0.3 mm–1.2 mm (DSV10 – DSVmax), and comparable between the anatomical sites (). On the other hand, the maximum distortion levels differed greatly between anatomical sites with H/N and pelvis generally showing higher mean and maximum distortion levels than brain and abdominal MRI sequences ( and Figures S1 and S2, supplementary material), and therefore a larger range of distortion levels. The distortion levels were a factor of 2–3 higher for the DWI sequences (). Individual sequence distortions are listed in Table S3 (supplementary materials).
Figure 2. Magnitude distortion (in mm) within a spherical volume with top) a diameter of 10 cm (DSV10) and bottom) with a maximum diameter Determined by the field-of-view (FOV) of the MRI sequence (DSVmax) for all clinical MRI sequences investigated. DSVmax for (brain, head/neck (H/N), abdomen, pelvis) = [30, 30, 40, 35] cm. The MRI sequences are divided into anatomical sites (brain, H/N, abdomen, pelvis) and sequence weightings representative for that anatomical site (e.g., T1w) on the x-axis. Marker color specifies MRI system and marker type specifies MRI sequence types (gradient-recalled echo (GRE), spin-echo (SE), and echo-planar imaging (EPI)). σ denotes standard deviation within a sequence group.
![Figure 2. Magnitude distortion (in mm) within a spherical volume with top) a diameter of 10 cm (DSV10) and bottom) with a maximum diameter Determined by the field-of-view (FOV) of the MRI sequence (DSVmax) for all clinical MRI sequences investigated. DSVmax for (brain, head/neck (H/N), abdomen, pelvis) = [30, 30, 40, 35] cm. The MRI sequences are divided into anatomical sites (brain, H/N, abdomen, pelvis) and sequence weightings representative for that anatomical site (e.g., T1w) on the x-axis. Marker color specifies MRI system and marker type specifies MRI sequence types (gradient-recalled echo (GRE), spin-echo (SE), and echo-planar imaging (EPI)). σ denotes standard deviation within a sequence group.](/cms/asset/36297d29-7369-4ab7-ac5d-d1cc9fdcd73c/ionc_a_2266560_f0002_c.jpg)
The standard deviation of the distortion levels within similar sequences for the different anatomical sites ranged between [0.16, 0.98] (listed at the top in ) mm for non-DWI sequences (DSVmax). Abdominal sequences performed consistently better that the other anatomical sites and the variation seen in sequences were at the same level as variation between scanners, indicating no real difference in geometric distortion level ( = [0.17, 0.28] and
= 0.27 for DSVmax). For the other anatomical sites, the variation exceeded the baseline variation indicating real differences in geometric distortion level between sequences.
An example of the effect of severe image distortion in a dose plan of the phantom is shown in , where a target is distorted in a pelvic 2D T2w SE MRI sequence from the dataset (ID 92, Table S2 and S3). In the coronal and sagittal plane, the PTV does not cover the target.
Figure 3. A Stereotactic dose plan for graphical illustration of the effect of image created in the treatment planning system pinnacle (Philips Healthcare, The Netherlands) on a CT reference dataset (top), where an off-axis sphere was delineated as a gross tumor volume (GTV, red) in the axial, sagittal and coronal plane. A planning target volume (PTV, cyan) was created by a 2 mm isotropic margin expansion of the GTV. The goal of the single arc volumetric modulated arc therapy (VMAT) dose plan was to cover the PTV by 30 Gy conformally while keeping steep dose gradients outside the target. One of the acquired pelvic MRI sequences (2D T2w SE, ID 92) (bottom) with a high distortion level was registered to the CT based on the central spheres. Iso-dose curves of 30 Gy (dark blue) and 20 Gy (magenta) are shown on CT images (top). the coverage of the PTV was compromised due to the distortions. Image distortion in the cranio-caudal direction are evident in this image data set, as also seen by the outer shape of the phantom.

Influence of MRI sequence parameters
Regardless of sequence type, distortion levels decreased with pBW (). Distortion levels also increased with increasing TE, except for 2D acquisitions, where no change or a slight decrease in distortion (DSV30) was observed as TE increased (). As regards DWI sequences, the read-out type had a strong influence on distortion level: Spin-Echo based-EPI (SE-EPI) read-out showed much higher distortion levels compared to Turbo Spin Echo (TSE) and GRE based-EPI (GRE-EPI) read-out, which used segmented readout with multiple shots (Siemens trade name, RESOLVE).
No significant difference (p = .48) in distortion level was found between T1w/T2w sequences (), but a clear difference was observed for 2D (DWI excluded) vs. 3D (p < .001, ) with median levels of 2.10 mm vs. 1.10 mm and maximum level of 4.07 mm vs. 1.60 mm (DSV30).
Figure 4. Influence of MRI sequence parameters on geometric distortion. (a) Magnitude distortion as a function of pixel bandwidth (pBW/B0) for 2D sequences. (b) Magnitude distortion as a function of echo time (TE). (c,d) same plots for 3D sequences. (e,f) Same plots for diffusion-weighted (DWI) sequences. Blue: distortion within a spherical volume with a diameter of 10 cm (DSV10). orange: distortion within DSV20. Yellow: distortion within DSV30. TSE: turbo spin-echo, GRE-EPI: echo planar imaging gradient-recalled echo (Siemens resolve sequence), SE-EPI: echo planar imaging spin echo. Dashed lines show trend lines.

Discussion
As geometric accuracy is a cornerstone of RT, the evolving role of MRI in RT demands special attention by the RT community to ensure acceptable levels of distortion, and sufficient consistency between centers. This study investigated the geometric accuracy of MRI sequences used in RT across nine centers.
The baseline geometric distortion between the 11 investigated MRI scanners ranged between 0.27 (DSV10, MRI2) and 2.01 (DSV40, MRL2) mm with a mean standard deviation of 0.23 mm, indicating the smallest measurable difference in distortion level across scanners. The highest distortion level in clinical MRI sequences was 5.02 (MRI2, H/N) and 10.89 (MRI4, pelvis) mm for anatomical and DWI sequences, respectively (DSVmax). The variation between similar sequence types used in brain, H/N, and pelvis was larger than the baseline variation between scanners, indicating a difference in distortion level due to sequence setup. The sequences used for brain and abdomen had better geometric accuracy than those used for H/N and pelvis. We also found that 2D MRI sequences had a higher distortion level than 3D MRI sequences. Generally the distortion level decreased with increasing bandwidth (pBW), whereas for increasing TE the distortion levels increased slightly (3D and DWI) but decreased for 2D. For DWI, the majority of SE-EPI based sequences had noticeably larger distortion levels than other DWI sequences.
Overall the baseline distortion levels of all scanners were lower than the recommended tolerance levels [Citation12,Citation14] and comparable between 1.5 T and 3 T. The distortion levels for the two 0.35 T scanners (MRL2 and MRL3) were larger. This could reflect sub-optimal implementation of the reference sequence, which is further supported by the low distortion levels for the clinical MRI sequences on these systems.
The in-house developed distortion analysis tool enabled analysis of MRI sequences with small FOV. The tool reported larger distortions compared to the commercial software, but similar overall trend with increasing distance to isocenter (Figure S3), when compared with the reference sequence. However, the tool agreed better with previously reported distortion levels across scanners [Citation8,Citation14,Citation40], also for the specific phantom and scanner used in this study [Citation41].
Brain and abdominal sequences had better geometric accuracy than H/N and pelvic sequences. A plausible explanation for this is that brain and abdominal sequences more frequently were 3D MRI acquisitions, and furthermore all abdominal sequences were default MRL sequences that presumably have been optimized for high geometric accuracy, since they are used more directly for treatment planning. On the other hand the MRL sequences in general had large voxel sizes (>1 mm), introducing some uncertainty in the estimations. In 3D MRI acquisitions, 3D distortion correction including through-slice distortion correction was applied, resulting in lower distortion levels than its 2D counterpart in agreement with the literature [Citation40,Citation42,Citation43]. Recently, 3D distortion correction has become available for multi-slice 2D sequences without slice-gap, and has reduced distortion levels for 2D sequences [Citation44–46]. In this study, 3D distortion correction for 2D sequences was not available, and the resulting higher distortion levels for the 2D sequences underlines the need for 3D distortion correction options. Another point to note is that although 3D sequences have better geometry, they are inherently slower, and may not provide the optimal T2-contrast in feasible scan times [Citation47].
The variation in geometric accuracy within the H/N and pelvis for similar sequence types was noticeable. For pelvic 2D T2w SE sequences, the range of distortion level was [0.81, 4.37] mm (DSVmax, Table S3 ID68 and ID88, respectively). A closer look reveals that the best performing sequence was acquired in the axial plane whereas the worst performing sequence was acquired in the coronal plane. The difference in distortion level might be due to the difference in the performance of gradient coils in the different directions, however these sequences are from different MRI systems. The influence of orientation was also observed for H/N where two similar 2D T2w SE (T2w mDIXON TSE sequences, ID40 and ID53) showed distortion levels of 1.26 mm and 3.57 mm in axial and coronal acquisitions, respectively. For T2w sequences, acquisition durations were comparable for 2D and 3D sequences (Figure S4(a)), so well performing sequences with clinical feasible acquisition times are feasible. The large effect of distortion in non-axial planes were seen in a hypothetical example in , where it resulted in areas of targets outside the PTV. This implies image distortions could have dosimetric consequences.
Figure 5. Boxplot showing distortion levels for 2D and 3D T1w/T2w/true FISP and 2D diffusion-weighted (DWI) MRI sequences within a spherical volume with a diameter of 30 cm (DSV30). the red line shows the median value. n denotes number of MRI sequences within each group.

We retrospectively analyzed the influence of certain parameters known to play a role for the geometric distortion levels [Citation7,Citation8,Citation10]. The aim was to identify which parameters contribute to the distortion level based on a set of real clinical sequences. As expected, we found a decrease in distortion level with increasing pBW. However, since the phantom used in this study did not contain materials with large differences in magnetic susceptibility, unlike in air/tissue interfaces in patients, only scanner-specific main field inhomogeneity was presumably reflected in the measured effect of pBW. Distortions due to main field inhomogeneity also scale with field strength (at constant BW), resulting in 3 T scanners being more prone to this source of distortion [Citation48], which again might not have be reflected with this phantom, and might be more pronounced in patients. Patient-induced distortions were not assessed in this study, but the magnitude of patient-induced distortion in RT has previously been reported elsewhere to be in the range of a maximum 0.3–1.5 mm (1.0–3 T) [Citation39,Citation49,Citation50]. Worth noting is also that although increasing pBW may lead to lower distortions, it also implies reduced sampling time, leading to a reduction in the signal-to-noise ratio (SNR) [Citation8] which could hamper lesion conspicuity. The effects of TE were small, when compared for all sequence types. Previous studies, where one sequence parameter is changed systematically, showed an effect of reducing distortion levels when reducing TE for GRE sequences [Citation7]. Since the majority of included clinical sequences investigated were SE based, it might explain the vague relation observed, as the refocusing radiofrequency realign the spins, and thereby reduce distortions due to field inhomogeneity. TE may however be confounded by many other factors not investigated in this study, due to the complex interactions between several sequence parameter.
As the role of DWI is evolving, ranging from aiding target delineation and dose-painting [Citation51], to prediction and response assessment [Citation52–54], the geometric integrity of the images should be considered in relation to the specific purpose. As EPI based DWI is especially prone to susceptibility-induced distortion in the phase-encoding direction (due to the low effective BW) it is important to choose the phase-encoding direction carefully to avoid the large distortions close to the target due to susceptibility differences from e.g., the air in the pelvis [Citation10,Citation55]. We found that for DWI sequences, the read-out method has a large effect, as EPI shows severe distortions (up to 12 mm for DSVmax), whereas other fast imaging sequences (e.g., turbo SE (TSE)) greatly reduced the observed distortions. Therefore, it should be considered to use TSE based DWI sequences for RT, as also suggested by AAMP Task Group 284 [Citation10]. TSE however is slower and the SNR is reduced due to long readout time. An alternative is the split echo diffusion weighted sequence (SPLICE) [Citation56,Citation57], or multi-shot/read-segmented EPI sequences, where the effective BW in phase-encoding direction is higher, and the distortion due to inhomogeneities therefore can be diminished [Citation55]. Kooreman et al. [Citation58,Citation59] reported a clear effect of the gradient non-linearity of the gradient system on the derived apparent diffusion coefficient (ADC) values, but the influence of susceptibility-induced distortions on ADC values is unknown.
In this multicenter study we focused on geometric accuracy exclusively, although other image quality parameters, such as resolution, scan time, a contrast to noise level, normal tissue and tumor conspicuity etc. [Citation25,Citation26,Citation28], may be just as important when selecting an MRI sequence for RT. However, the strength of this study is the large catalogue of clinical sequences investigated, ensuring clinical relevance. Within each of the investigated anatomies, there is room for improvement regarding geometrical accuracy, without losing other important image quality features and within clinical feasible scan times. To our knowledge, a study with this aim and of this size has not been published before. We hope that these results can guide attempts for harmonization of MRI sequences between centers nationally, potentially also internationally through collaborative networks in the RT community. Based on these findings, NIMBUS invites the Danish Multidisciplinary Cancer Groups (dmcg.dk) to discuss possible strategies for improving MRI sequences.
In conclusion, a large variation in performance within anatomical sites was found, indicating that a nationwide improvement of MRI sequences may be relevant. Harmonization of different types of clinical MRI sequences across centers could mitigate the large variation in sequence performance that was found and heighten the overall quality of MR images used for RT on a national basis and in multicenter trials.
Supplemental Material
Download MS Word (1.1 MB)Acknowledgements
We thank the radiographers at all participating centers for skillful assistance during data collection. We would like to thank Claus P. Behrens and Ivan R. Vogelius for contributing with discussions regarding the clinical relevance of the study.
Disclosure statement
Odense University Hospital has research agreements with Philips (Eindhoven, The Netherlands) and Elekta AB (Stockholm, Sweden).
Data availability statement
The raw image data of this study are available from the corresponding author, SWH, upon request. The authors confirm that the data supporting the findings of this study are available within the article’s supplementary materials (Table S2 and S3).
Additional information
Funding
References
- Edmund JM, Nyholm T. A review of substitute CT generation for MRI-only radiation therapy. Radiat Oncol. 2017;12(1):28. doi: 10.1186/s13014-016-0747-y.
- Seppälä T, Visapää H, Collan J, et al. Converting from CT- to MRI-only-based target definition in radiotherapy of localized prostate cancer. Strahlenther Onkol. 2015;191(11):862–868. http://link.springer.com/10.1007/s00066-015-0868-5.
- Raaymakers BW, Lagendijk JJW, Overweg J, et al. Integrating a 1.5 T MRI scanner with a 6 MV accelerator: proof of concept. Phys Med Biol. 2009;54(12):N229–N237. doi: 10.1088/0031-9155/54/12/N01.
- Bertelsen AS, Schytte T, Møller PK, et al. First clinical experiences with a high field 1.5 T MR linac. Acta Oncol. 2019;58(10):1352–1357. doi: 10.1080/0284186X.2019.1627417.
- Wang D, Strugnell W, Cowin G, et al. Geometric distortion in clinical MRI systems. Magn Reson Imaging. 2004;22(9):1211–1221. doi: 10.1016/j.mri.2004.08.012.
- Weygand J, Fuller CD, Ibbott GS, et al. Spatial precision in magnetic resonance imaging-guided radiation therapy: the role of geometric distortion. Int J Radiat Oncol Biol Phys. 2016;95(4):1304–1316. doi: 10.1016/j.ijrobp.2016.02.059.
- Port JD, Pomper MG. Quantification and minimization of magnetic susceptibility artifacts on GRE images. J Comput Assist Tomogr. 2000;24(6):958–964. doi: 10.1097/00004728-200011000-00024.
- Walker A, Liney G, Metcalfe P, et al. MRI distortion: considerations for MRI based radiotherapy treatment planning. Australas Phys Eng Sci Med. 2014;37(1):103–113. doi: 10.1007/s13246-014-0252-2.
- Jezzard P, Clare S. Sources of distortion in functional MRI data. Hum. Brain Mapp. 1999;8(2–3):80–85. doi: 10.1002/(SICI)1097-0193(1999)8:2/3<80::AID-HBM2>3.0.CO;2-C.
- Glide-Hurst C, Paulson ES, McGee KP, et al. Task group 284 report: magnetic resonance imaging simulation in radiotherapy: considerations for clinical implementation, optimization, and quality assurance. Med Phys. 2021;48:e636–e670.
- Moerland MA, Beersma R, Bhagwandien R, et al. Analysis and correction of geometric distortions in 1.5 T magnetic resonance images for use in radiotherapy treatment planning. Phys Med Biol. 1995;40(10):1651–1654. doi: 10.1088/0031-9155/40/10/007.
- Patel I. Physics aspects of quality control in radiotherapy – IPEM report 81. 2nd ed. New York: Institution of Physics & Engineering in Medicine & Biology; 2018.
- Paulson ES, Crijns SPM, Keller BM, et al. Consensus opinion on MRI simulation for external beam radiation treatment planning. Radiother Oncol. 2016;121(2):187–192. doi: 10.1016/j.radonc.2016.09.018.
- Kavaluus H, Nousiainen K, Kaijaluoto S, et al. Determination of acceptance criteria for geometric accuracy of magnetic resonance imaging scanners used in radiotherapy planning. Phys Imaging Radiat Oncol. 2021;17:58–64. doi: 10.1016/j.phro.2021.01.003.
- Lagendijk JJW, Raaymakers BW, Raaijmakers AJE, et al. MRI/linac integration. Radiother Oncol. 2008;86(1):25–29. doi: 10.1016/j.radonc.2007.10.034.
- Bol GH, Lagendijk JJW, Raaymakers BW. Virtual couch shift (VCS): accounting for patient translation and rotation by online IMRT re-optimization. Phys Med Biol. 2013;58(9):2989–3000. doi: 10.1088/0031-9155/58/9/2989.
- Klüter S. Technical design and concept of a 0.35 T MR-Linac. Clin Transl Radiat Oncol. 2019;18:98–101.
- Niyazi M, Brada M, Chalmers AJ, et al. ESTRO-ACROP guideline “target delineation of glioblastomas. Radiother Oncol. 2016;118(1):35–42. doi: 10.1016/j.radonc.2015.12.003.
- Ellingson BM, Bendszus M, Boxerman J, et al. Consensus recommendations for a standardized brain tumor imaging protocol in clinical trials. Neuro Oncol. 2015;17:1188–1198.
- Jensen K, Al-Farra G, Dejanovic D, et al. Imaging for target delineation in head and neck cancer radiotherapy. Semin Nucl Med. 2021;51(1):59–67. doi: 10.1053/j.semnuclmed.2020.07.010.
- DNOG. Dansk Neuro Onkologisk Gruppe Retningslinjer for strålebehandling [Danish Neuro Oncology Group Guidelines for Radiotherapy]. 2016.
- DAPROCA. Billeddiagnostik ved prostatacancer [Medical Imaging for Prostate Cancer Danish Guidelines]. 2022.
- van der Heide UA, Frantzen-Steneker M, Astreinidou E, et al. MRI basics for radiation oncologists. Clin Transl Radiat Oncol. 2019;18:74–79. doi: 10.1016/j.ctro.2019.04.008.
- Bertelsen A, Bernchou U, Schytte T, et al. The effect of respiration-induced target motion on 3D magnetic resonance images used to guide radiotherapy. Phys Imaging Radiat Oncol. 2022;24:167–172. doi: 10.1016/j.phro.2022.11.010.
- Whiteside L, McDaid L, Hales RB, et al. To see or not to see: evaluation of magnetic resonance imaging sequences for use in MR linac-based radiotherapy treatment. J Med Imaging Radiat Sci. 2022;53(3):362–373. doi: 10.1016/j.jmir.2022.06.005.
- Devic S. MRI simulation for radiotherapy treatment planning. Med Phys. 2012;39(11):6701–6711. doi: 10.1118/1.4758068.
- Nousiainen K, Mäkelä T, Peltonen JI. Characterizing geometric distortions of 3D sequences in clinical head MRI. MAGMA. 2022;35(6):983–995. doi: 10.1007/s10334-022-01020-8.
- Eccles CL, Adair Smith G, Bower L, et al. Magnetic resonance imaging sequence evaluation of an MR linac system; early clinical experience. Tech Innov Patient Support Radiat Oncol. 2019;12:56–63. doi: 10.1016/j.tipsro.2019.11.004.
- Danish Comprehensive Cancer Center. National interdisciplinary network for quality assurance of MR images in radiotherapy (NIMBUS) [Internet]. https://www.dccc.dk/english/projectsandnetwork/nimbus/.
- The Phantom Laboratory. Magphan ® RT phantom product guide. Salem: The Phantom Laboratory; 2022. p. 1–20.
- Baldwin LN, Wachowicz K, Fallone BG. A two-step scheme for distortion rectification of magnetic resonance images. Med Phys. 2009;36(9):3917–3926. doi: 10.1118/1.3180107.
- Baldwin LN, Wachowicz K, Thomas SD, et al. Characterization, prediction, and correction of geometric distortion in 3 T MR images. Med Phys. 2007;34(2):388–399. doi: 10.1118/1.2402331.
- Nejad-Davarani SP, Kim JP, Du D, et al. Large field of view distortion assessment in a low-field MR-linac. Med Phys. 2019;46(5):2347–2355. doi: 10.1002/mp.13467.
- NEMA PS3/ISO 12052. Digital imaging and communications in medicine (DICOM) standard. [Internet]. Rosslyn (VA): National Electrical Manufacturers National Electrical Manufacturers. http://medical.nema.org/.
- Jezzard P, Balaban RS. Correction for geometric distortion in echo planar images from B0 field variations. Magn Reson Med. 1995;34(1):65–73. doi: 10.1002/mrm.1910340111.
- Andersson JLR, Skare S, Ashburner J. How to correct susceptibility distortions in spin-echo echo-planar images: application to diffusion tensor imaging. Neuroimage. 2003;20(2):870–888. doi: 10.1016/S1053-8119(03)00336-7.
- Danziger Z. Theil-Sen Robust linear regression [Internet]. MATLAB Cent. File Exch. 2023 [cited 2023 May 8]. https://www.mathworks.com/matlabcentral/fileexchange/48294-theil-sen-robust-linear-regression.
- Xie L. Spherical Hough transform for 3D images [Internet]. MATLAB Cent. File Exch. 2023 [cited 2023 May 3]. https://uk.mathworks.com/matlabcentral/fileexchange/48219-spherical-hough-transform-for-3d-images.
- Hasler SW, Bernchou U, Bertelsen A, et al. Tumor-site specific geometric distortions in high field integrated magnetic resonance linear accelerator radiotherapy. Phys Imaging Radiat Oncol. 2020;15:100–104. doi: 10.1016/j.phro.2020.07.007.
- Keesman R, van de Lindt TN, Juan-Cruz C, et al. Correcting geometric image distortions in slice-based 4D-MRI on the MR-linac. Med Phys. 2019;46(7):3044–3054. doi: 10.1002/mp.13602.
- Walker A, Chlap P, Causer T, et al. Development of a vendor neutral MRI distortion quality assurance workflow. J Appl Clin Med Phys. 2022;23(10):e13735.
- Yahanda AT, Goble TJ, Sylvester PT, et al. Impact of 3-dimensional versus 2-dimensional image distortion correction on stereotactic neurosurgical navigation image fusion reliability for images acquired with intraoperative magnetic resonance imaging. Oper Neurosurg. 2020;19(5):599–607. doi: 10.1093/ons/opaa152.
- Karger CP, Höss A, Bendl R, et al. Accuracy of device-specific 2D and 3D image distortion correction algorithms for magnetic resonance imaging of the head provided by a manufacturer. Phys Med Biol. 2006;51(12):N253–N261. doi: 10.1088/0031-9155/51/12/N04.
- Kato Y, Okudaira K, Kamomae T, et al. Evaluation of system-related magnetic resonance imaging geometric distortion in radiation therapy treatment planning: two approaches and effectiveness of three-dimensional distortion correction. Nagoya J Med Sci. 2022;84:29–41.
- Haukipuro E. 3D geometric correction in 2D multi-slice acquired MR images used in radiation therapy planning [Master's Thesis, Aalto University]; 2020.
- Torfeh T, Hammoud R, Perkins G, et al. Characterization of 3D geometric distortion of magnetic resonance imaging scanners commissioned for radiation therapy planning. Magn Reson Imaging. 2016;34(5):645–653. doi: 10.1016/j.mri.2016.01.001.
- Stemkens B, Paulson ES, Tijssen RHN. Nuts and bolts of 4D-MRI for radiotherapy. Phys Med Biol. 2018;63(21):21TR01. doi: 10.1088/1361-6560/aae56d.
- Stanescu T, Wachowicz K, Jaffray DA. Characterization of tissue magnetic susceptibility-induced distortions for MRIgRT. Med Phys. 2012;39(12):7185–7193. doi: 10.1118/1.4764481.
- Tijssen RHN, Vos R, Philippens MEP, et al. Online geometric fidelity inspection for MR-Guided treatments on 1.5 T MRI-Linac: vosualizing the cumulative effect of gradient errors and patient specific suceptibilities. MRinRT. 2019;7:58.
- Glide-Hurst C, Nejad-Davarani S, Weiss S, et al. Per-organ assessment of subject-induced susceptibility distortion for MR-only male pelvis treatment planning. Radiat Oncol. 2018;13(1):149. doi: 10.1186/s13014-018-1090-2.
- Kerkmeijer LGW, Groen VH, Pos FJ, et al. Focal boost to the intraprostatic tumor in external beam radiotherapy for patients with localized prostate cancer: results from the FLAME randomized phase III trial. J Clin Oncol. 2021;39(7):787–796. doi: 10.1200/JCO.20.02873.
- Gurney-Champion OJ, Mahmood F, van Schie M, et al. Quantitative imaging for radiotherapy purposes. Radiother Oncol. 2020;146:66–75. doi: 10.1016/j.radonc.2020.01.026.
- van Houdt PJ, Saeed H, Thorwarth D, et al. Integration of quantitative imaging biomarkers in clinical trials for MR-guided radiotherapy: conceptual guidance for multicentre studies from the MR-Linac consortium imaging biomarker working group. Eur J Cancer. 2021;153:64–71. doi: 10.1016/j.ejca.2021.04.041.
- Mahmood F, Hjorth Johannesen H, Geertsen P, et al. Diffusion MRI outlined viable tumour volume beats GTV in intra-treatment stratification of outcome. Radiother Oncol. 2020;144:121–126. doi: 10.1016/j.radonc.2019.11.012.
- Bergen RV, Ryner L, Essig M. Field-map correction in read-out segmented echo planar imaging for reduced spatial distortion in prostate DWI for MRI-guided radiotherapy applications. Magn Reson Imaging. 2020;67:43–49. doi: 10.1016/j.mri.2019.12.005.
- Schick F. SPLICE: sub-second diffusion-sensitive MR imaging using a modified fast spin-echo acquisition mode. Magn Reson Med. 1997;38(4):638–644. doi: 10.1002/mrm.1910380418.
- Rahbek S, Schakel T, Mahmood F, et al. Optimized flip angle schemes for the split acquisition of fast spin-echo signals (SPLICE) sequence and application to diffusion-weighted imaging. Magn Reson Med. 2023;89(4):1469–1480. doi: 10.1002/mrm.29545.
- Kooreman ES, van Houdt PJ, Nowee ME, et al. Feasibility and accuracy of quantitative imaging on a 1.5 T MR-linear accelerator. Radiother Oncol. 2019;133:156–162. https://linkinghub.elsevier.com/retrieve/pii/S0167814019300167. doi: 10.1016/j.radonc.2019.01.011.
- Kooreman ES, van Houdt PJ, Keesman R, et al. ADC measurements on the Unity mr-linac – A recommendation on behalf of the Elekta Unity MR-linac consortium. Radiother Oncol. 2020;153:106–113. doi: 10.1016/j.radonc.2020.09.046.