ABSTRACT
Testosterone is a sex hormone produced by testicular Leydig cells in males. Blood testosterone concentrations increase at three time-periods in male life–fetal, neonatal (which can be separated into newborn and infant periods), and pubertal stages. After peaking in the early 20s, the blood bioactive testosterone level declines by 1–2% each year. It is increasingly apparent that a low testosterone level impairs general physical and mental health in men. Here, this review summarizes recent systematic reviews and meta-analyses of epidemiological studies in males (including cross-sectional, longitudinal, and androgen deprivation studies, and randomized controlled testosterone replacement trials) in relation to testosterone and obesity, body composition, metabolic syndrome, type 2 diabetes, cardiovascular disease, and longevity. Furthermore, underlying mechanisms are discussed using data from rodent studies involving castration or androgen receptor knockout. This review provides an update understanding of the role of testosterone in energy metabolism.
Abbreviations AR: androgen receptor; CV: cardiovascular; FDA: US Food and Drug Administration; HFD: high-fat diet; KO: knockout; MetS: metabolic syndrome; RCT: randomized controlled trial; SHBG: sex hormone binding globulin; SRMA: systematic review and meta-analysis; TRT: testosterone replacement therapy; T2DM:type 2 diabetes mellitus
Graphical Abstract
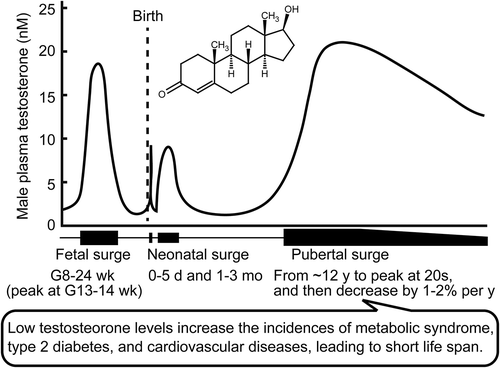
Testosterone levels in a human male. Low testosterone levels impair health in men. G, gestation; h, hour; mo, month; wk, week; y, year.
Blood testosterone levels in life
Testosterone is the primary male sexual hormone and is mostly produced by Leydig cells in the testis. Blood testosterone concentrations rise during three phases of human male life–fetal, neonatal (which can be separated into newborn and infant periods), and at sexual maturity () [Citation1–Citation3]. The first testosterone surge occurs at around 8–24 weeks’ gestation, with a peak at around 13–14 weeks’ gestation in humans [Citation4–Citation7]; in rats, the surge starts at E15.5 and peaks at E18.5 [Citation8,Citation9] and in mice these events occur at E14 and E18 [Citation10]. This surge in the middle of the gestation period is required for male sexual differentiation and asynchronously masculinizes the internal reproductive organs (e.g., prostate, seminal vesicle, and epididymis) and the external genitalia [Citation11,Citation12]. The second testosterone surge occurs at neonatal phase (i.e., newborn and infants). The testosterone level is elevated for several days after delivery; this then declines prior to a subsequent surge from 1–5 months, with a peak at around 1–3 months [Citation2,Citation7,Citation13]. The testosterone level is also elevated for several hours after delivery in rodents [Citation2,Citation14]. The neonatal testosterone surge contributes to brain masculinization [Citation2]. In the third pubertal surge, the testosterone level starts to rise in 12-year old in humans [Citation15], and increases until a peak in the early 20s; the equivalent rise starts at 5–6 weeks of age in rats [Citation16,Citation17] and at 4 weeks of age in mice [Citation10,Citation18]. This pubertal testosterone rise contributes to the development of secondary sexual characteristics and reproductive capacity.
Figure 1. Testosterone surges in a human male. Three testosterone surges–fetal, neonatal, and pubertal surges, are indicated. Testosterone levels among men (in Western countries) decline with age. G, gestation; mo, month; wk, week; y, year.
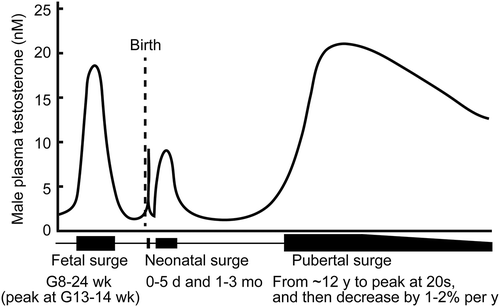
In blood, 50–60% of the testosterone is strongly bound to sex hormone binding globulin (SHBG), 40–50% binds weakly to albumin, and 1–2% testosterone exists as the free form [Citation19]. Free and albumin-bound testosterone are bioactive. After sexual maturation, the levels of total and bioactive testosterone steadily decrease by 1–2% per year [Citation19]. A low blood testosterone level in men is a criterion for the diagnosis of late-onset hypogonadism, LOH, which impairs general physical and mental health status and is diagnosed in ~ 2% of elderly men [Citation20]. Total and free testosterone levels of less than 11 nM and 0.22 nM, respectively, are the minimum criteria for the diagnosis of late-onset hypogonadism in Europe [Citation20]. SHBG shows high-affinity binding to testosterone and low-affinity binding to estrogen; the levels of this binding protein therefore affects the ratio of androgen and estrogen delivery to target tissues. Testosterone levels are also dramatically reduced by physical castration (orchidectomy) or chemical castration using a luteinizing hormone-releasing hormone analog, because testosterone is predominantly synthesized in response to luteinizing hormone.
Androgens and energy metabolism: human clinical and epidemiological studies
The expected life-span is about 6 years longer for women than for men in developed countries [Citation21]. Cardiovascular (CV) morbidity and mortality is over 2-fold greater in men than in women. The effects of sex hormones can explain many physiological differences between males and females. Estrogen has the potential to decrease CV risk, which rises after menopause in women [Citation21]. Therefore, the relatively low levels of estrogen in men could contribute to this sex difference. The Coronary Drug Project examined whether estrogen was protective against CV disease in men [Citation22]. However, contrary to expectations, estrogen treatment was found to increase the risk for unexpected CV mortality in men. This project highlighted the important concept that sex hormones do not produce the same outcomes in men and women with respect to the prevention of CV disease. In the past decade, a low level of testosterone, rather than a low level of estrogen, has become increasingly apparent as a CV risk factor in men [Citation23,Citation24].
Many clinical and epidemiological studies have assessed the effects of testosterone on all-cause death or mortality and morbidity due to metabolic diseases. Unfortunately, the conclusions of these studies are not always consistent due to differences in methodology, subjects, and/or population size. The systematic review and meta-analysis (SRMA) provides a valuable tool for the identification of reliable evidence base by overviewing all the available studies more reliable results by overviewing all the available studies. SRMA is useful for the evaluation of multiple datasets with conflicting results or limited statistical power. Since 2001, a significant number of SRMA reports relating to testosterone and metabolism and/or CV disease have been published. Relevant epidemiological studies have employed various models, including cross-sectional and prospective longitudinal study designs. This review has summarized these SRMA studies in men ().
Table 1. Summary of meta-analyses and a large-cohort studies of testosterone and metabolic disorders.
Relationships between testosterone and CV disease and/or longevity
SRMA studies of prospective longitudinal cohort research have indicated that low total testosterone levels are associated with increased all-cause and CV death [Citation25,Citation26]. A decrease in blood total testosterone by 2.18 standard deviations below the normal range increases the risk of all-cause and CV mortality by 35% and 25%, respectively [Citation25]. This effect was detected in the SRMA of prospective longitudinal studies (i.e., with observations at multiple time-points), suggesting a causal effect of low testosterone levels on these death rates. In addition, SRMA also indicated that a higher level of estrogen (17β-estradiol) was a risk factor for CV morbidity and mortality in men [Citation26], supporting the findings of The Coronary Drug Project [Citation22]. On the other hand, one SRMA found no association between low testosterone and CV morbidity, although it did increase all-cause and CV death [Citation26]. Another SRMA showed no correlation between a low testosterone level and CV morbidity risk in middle-aged men, but increased the risk in elderly subjects aged > 70 years-old [Citation27]. Collectively, these data indicated that low levels of testosterone (hypogonadism) appeared to increase the risk of early death. In addition, this may elevate CV risk in elderly men.
Testosterone replacement therapy (TRT) can boost blood testosterone levels and confer many beneficial effects such as maintaining muscle mass and bone mineral density, and improving quality of life [Citation28–Citation30]. Clinical use of TRT, which began ~ 70 years ago [Citation31], would be expected to improve outcomes and extend life-span in hypogonadal subjects [Citation32,Citation33]. However, several SRMA studies have failed to identify any association between TRT and CV morbidity [Citation34–Citation38] or CV death [Citation30,Citation36]. Meanwhile, one SRMA concluded that TRT increased CV risk in studies that were not funded by the pharmaceutical industry [Citation39]; another SRMA indicated that oral TRT, but not injected or transdermal TRT, increased CV risk [Citation40]. In response to reports of TRT side effects, both the US Food and Drug Administration (FDA) and Health Canada have issued warnings related to the usage of testosterone products [Citation41,Citation42]. The FDA, however, also emphasized that the benefits and safety of medications containing testosterone have not yet been established for the treatment of hypogonadal men. In contrast to the early warning from the FDA, The American Association of Clinical Endocrinologists and The American College of Endocrinology have produced an official position statement indicating that “there is no compelling evidence that testosterone therapy either increases or decreases cardiovascular risk” [Citation43]. Similarly, The European Pharmacovigilance Risk Assessment Committee did not find an association between TRT and cardiovascular risk [Citation44]. These discrepant findings related to TRT and CV risk arise from the methods and doses employed. Oral TRT fails to increase testosterone levels to the same extent as parenteral or transdermal delivery systems [Citation45], and may also cause hepatic injury [Citation31]. Notably, a recent large, well-designed, retrospective study of 83,010 veterans reported the relationship between an adequate TRT-induced rise in the testosterone level and significant decreases in all-cause mortality and CV risk [Citation46]. This large cohort study suggest that patients who failed to achieve adequate testosterone levels using TRT would not benefit from this treatment.
Relationships between testosterone and metabolic syndrome (MetS) or type 2 diabetes mellitus (T2DM)
MetS includes a set of CV disease factors such as abdominal obesity, dysglycemia (i.e., elevated fasting glucose), dyslipidemia (i.e., elevated triglyceride levels and low high-density lipoprotein (HDL) cholesterol levels), and hypertension [Citation47]. MetS is a risk factor for T2DM, which also increases risk for CV disease. Body fat distribution differs in men and women, with an android type (visceral accumulation) in men and the gynoid type (gluteal and femoral accumulation) in women [Citation48]; visceral fat obesity is also a prevalent feature of MetS [Citation49], suggesting the involvement of sex hormones in these fat distribution patterns and in MetS. Actually, SRMA of cross-sectional studies has revealed that a low testosterone level is commonly associated with an increased risk for MetS [Citation50–Citation54] or T2DM [Citation53,Citation55], after adjusting for age and body-mass index or lifestyle. SRMA of longitudinal studies also suggest a causal effect of low total testosterone levels on the prevalence of MetS [Citation50,Citation51] and T2DM [Citation54,Citation55] in men, while this associates with a lower risk in women [Citation54]. In addition, a low total testosterone level is an independent risk factor for MetS and T2DM [Citation50], while a low free testosterone level also increases the risk for MetS [Citation51]. A SRMA of longitudinal studies indicated that low total or free testosterone is associated with abdominal obesity, high triglyceride levels, hyperglycemia, low HDL levels, and hypertension [Citation51]. These associations were still present for the low total testosterone level following adjustment for age and lifestyle. This association was strongest for abdominal obesity, hyperglycemia, and high triglyceride levels, and weakest for hypertension [Citation51]. In addition, an increase in adipocyte mass and hyperglycemia can reduce testosterone reduction, resulting in progressive hypogonadism [Citation56].
A SRMA of randomized controlled trials (RCTs) indicated that TRT affected metabolic factors in hypogonadal men. TRT can reduce body fat mass and increase the fat-free mass, without affecting body weight, body-mass index, or waist circumference [Citation29,Citation45]. Reduction in fasting blood glucose levels and insulin resistance (using homeostatic model assessment) were also achievable [Citation45]. TRT commonly decreases total cholesterol levels [Citation29,Citation38,Citation45,Citation57]; however, no effect [Citation29] or lowering effects [Citation57] have been reported for LDL cholesterol, while a significant reduction [Citation36], a tendency to be lower [Citation57], or no effect [Citation45] has been reported for HDL cholesterols.
Some clear beneficial effects on metabolic factors have been reported in MetS and T2DM patients receiving TRT. In hypogonadal MetS patients, SRMA identified benefits of TRT including reductions in waist circumference, fasting glucose, and insulin resistance, and an increase in HDL levels [Citation50,Citation53]. SRMA of studies of T2DM patients with hypogonadism indicated that TRT reduced fasting glucose, glycated hemoglobin (HbA1c), triglyceride, and body fat, but had no effect on total cholesterol or HDL cholesterol levels, blood pressure, or body-mass index [Citation53,Citation55,Citation58]. In contrast, patients with MetS and/or T2DM in the absence of classic hypogonadism did not show these TRT benefits relating to glycemic control [Citation59].
A recent SRMA of observational studies indicated that the duration of TRT administration affected patient outcomes [Citation60]. Reductions in body weight and waist circumference of −3.5 kg and −6.23 cm, respectively were apparent after 2 years, but less marked after 1 year (−0.62 kg and −3.50 cm, respectively). In addition, a meta-regression analysis indicated that the reductions in body weight and waist circumference were more obvious in younger and more hypogonadal subjects. Generally, shorter follow-up terms are employed in RCTs (average of 9 months) than in observational studies (average of > 18 months). The SRMA of observational studies identified the improvements in fat mass, lean body mass, fasting glycemia, and insulin resistance, as well as in the levels of triglyceride, and total and HDL cholesterol. In a large retrospective study of TRT, normalization of testosterone levels conferred benefits relating to body-mass index, diabetes, and hypertension; the follow up of the normalized-TRT group was 3.0 ± 2.7 years and that of the non-normalized group was 1.5 ± 1.9 years [Citation46]. Further long-term follow-up RCTs are highly desirable.
Effects of androgen deprivation therapy for prostate cancer patients on MetS, T2DM, and CV disease
Prostate cancer is the most frequently diagnosed cancer in men and the second leading causes of cancer death in men [Citation61]. Androgen deprivation by physical castration or chemical approaches using luteinizing hormone-releasing hormone analogs or androgen receptor (AR) blockade by antiandrogens is often used before, during, and after radiation therapy for prostate cancer. The side effects of this hormone therapy on diabetes and cardiovascular risk were first reported at 2006 [Citation62]. A SRMA indicated that androgen deprivation therapy reduced the risk of all-cause mortality, without affecting CV death, demonstrating the benefits of this therapy [Citation63]. However, other SRMA studies found 10–38% and 17% increases in the incidence of CV disease and CV death, respectively [Citation64,Citation65], and a 12% increase in the risk for stroke [Citation66]. SRMAs have also found that androgen deprivation therapy altered body composition by increasing body fat and decreasing lean body mass [Citation67], increased the risk of diabetes by 36–39% [Citation68,Citation69], and increased the risk for MetS by 75% [Citation69]. These results provide a consensus that androgen deprivation increases the risk of T2DM, MetS, and CV disease. It is important to note that this relationship indicates a causal of low androgen levels on metabolic and CV diseases.
Summary of clinical and epidemiological studies
Epidemiological cohort studies indicate that a low testosterone level is robustly associated with an increased incidence of CV disease in males. The associated obesity, T2DM, and altered lipid profiles are linked to an increased CV risk [Citation49]. Although androgen deprivation therapy for prostate cancer patients can reduce overall mortality [Citation63], it can increase the risk for CV disease by influencing body composition (i.e., increasing fat and decreasing lean mass) and glycemic control. These results strongly suggest a causal relationship between a loss of androgen function, metabolic disorder, and CV morbidity. The observational period employed was found to affect extent of the body weight changes observed because a loss of lean mass decreases energy expenditure, leading to an increase in energy storage [Citation60].
Overall, TRT may protect patients with hypogonadism from MetS and T2DM. TRT-mediated protection from CV disease is most marked in subjects with metabolic derangements [Citation34]. However, the effect of TRT on CV risk remains controversial. This may reflect some differences in the duration of TRT administration or the study endopoint. Although TRT is effective in hypogonadal men, blood testosterone levels are affected by both circadian rhythms [Citation70] and seasonal variation [Citation71]. A SRMA of placebo-controlled RCTs demonstrated that the administration route affected the circulating testosterone levels, with a mean testosterone difference versus placebo of 7.69 nM (parenteral), 7.57 nM (transdermal), or 2.39 nM (oral) [Citation45]. The routes of delivery currently approved by the FDA are buccal, nasal, subdermal, transdermal, and intramuscular. Oral administration fails to exert positive effects due to extensive testosterone metabolism by detoxification enzymes in the liver [Citation31]. Although some modification to testosterone can avoid this metabolism, this modification causes liver toxicity [Citation31]. For these reasons, oral TRT is now not recommended. In addition to the delivery route, the pharmacokinetics of the testosterone-derivatives used for TRT are variable [Citation31]. A recent large longitudinal cohort study revealed the importance of circulating testosterone levels to the outcome of TRT [Citation46]. Hematocrit levels are closely associated with TRT-induced increase in testosterone levels, and these can be used to monitor patients [Citation35–Citation37]. This is important because both supraphysiological and subphysiological levels of testosterone can increase the risk of CV disease in men, indicating a U-shaped relationship between these factors [Citation72–Citation75]. In addition, delivery of excess testosterone does not bring any added beneficial effects [Citation75]. Therefore, more consistent outcomes could be achieved by selection of the most appropriate delivery route, by monitoring testosterone levels, and by determining the optimum treatment duration.
In contrast to studies conducted in European and American men, total testosterone levels were not found to decline with age in Japanese men [Citation76]. Different clinical and pathophysiological phenotypes are also observed in T2DM patients and obese subjects in Asian and Western populations [Citation48,Citation77]. In addition, Korean historical records (from the 14th to the early 20th century) indicate that Korean eunuchs (castrated men) had longer lifespans than non-castrated men of a similar socio-economic status [Citation78]. On the other hand, the lifespans of prepubertally castrated males and intact male singers did not differ in Europe between the end of the 16th century to the middle of the 19th century [Citation79]. Epidemiological studies have mostly been performed in Western countries and it is therefore important to investigate the effects of testosterone in study groups from Asia and other regions. In addition, it is also unclear whether the association between low testosterone levels and high mortality appeared in the late of 20th and 21st centuries.
The etiology of hypogonadism involves a decline in testosterone synthesis by Leydig cells during aging [Citation80]. A decline in the hypothalamus–pituitary axis is also observed in hypogonadal men [Citation81]. Exogenous TRT reduces endogenous testosterone production via negative feedback of the hypothalamus–pituitary axis, and this may accelerate the decline of the testes. This indicates that in longer term, increasing endogenous testosterone production represents a better alternative to TRT. Therefore, it is reasonable to investigate whether endogenous testosterone levels can be increased by physical exercise [Citation82] or dietary interventions (e.g., low calorie diet [Citation83]) before administering TRT.
Androgens and energy metabolism: rodent mechanistic studies
Mechanisms of action
The effects of androgens such as testosterone and its active metabolite, dihydrotestosterone, are mediated by the AR, which is a member of the nuclear receptor superfamily of ligand-activated transcription factors [Citation84]. The ligand-activated AR binds to the androgen response element within target gene promoters [Citation85] and thus stimulates the transcription of target genes. Coactivators and corepressors are essential for the modulation of AR transactivation [Citation86–Citation91]. Exon 1 in the AR gene contains polymorphic CAG and GGN trinucleotide repeats, which affect the transcriptional activity of the AR [Citation92]. Two separate short polyglutamine repeats, proximal to the C-terminus of the polymorphic polyglutamine tract, are also involved in AR transactivation [Citation93]. AR-mediated androgen activities contribute to the development and functions of male reproductive tissues [Citation94] such as the prostate, seminal vesicle, and epididymis, and also to the development of non-reproductive tissues such as the liver [Citation95], adipocyte [Citation96,Citation97], muscle [Citation98], kidney [Citation99], heart [Citation100], adrenal gland [Citation101], and pancreatic β-cells [Citation102,Citation103]. The mechanisms underlying the links between testosterone and energy metabolism have been studied using rodent models of castration and AR knockout (AR-KO). The AR-KO model is useful for understanding the role of androgen in specific organs [Citation104–Citation110]. Castration decreased heart weight [Citation111], suggesting a role in the heart. Global AR-KO produces angiotensin II-induced cardiac fibrosis [Citation100]. AR-KO and castration accelerated atherosclerosis in apolipoprotein E-deficient background [Citation112], whereas in mice lacking a receptor for LDL, macrophage-specific AR-KO reduced atheromatous lesions [Citation113]. These results suggest that androgens directly affect the cardiac muscle cells, as well as the vascular endothelial and smooth muscle cells, and thus have an effect on CV disease without affecting energy metabolism [Citation114]. This review focuses on rodent studies of the effects of androgen ablation on energy metabolism, including body composition and T2DM, because these were involved in CV risk and are evident and important in the clinical and epidemiological studies described above. In addition, the characteristics of global and tissue-specific AR-KO mice are summarized in .
Table 2. Summary of the metabolic changes in androgen receptor knockout male mice.
Body weight, obesity, and adipose tissue mass
Changes in body composition following castration are well characterized in domesticated animals such as cattle, sheep, and pigs [Citation115,Citation116]. As a common feature, castration increases the ratio of fat mass to lean mass, but its effects on feed efficiency (body weight gain/calorie intake) and body weight are not consistent [Citation115,Citation116]. Aggressive body weight gain and increased food consumption are observed in rabbits and pigs following castration [Citation116,Citation117]. Castration-dependent weight gain is also observed in hamsters [Citation118]; in contrast to humans, the adult female hamster is heavier than the male [Citation119]. Although the increase in calorie intake resulting from increased food consumption directly causes body weight gain, castration decreases calorie intake in male mice and rats [Citation111,Citation120–Citation123]. Investigations of castration-induced obesity and adipose tissue accumulation are rather limited in these extensively studied laboratory animals. Although it is still unclear whether testosterone affects food consumption in humans, accumulating data from clinical and epidemiological studies indicate that a low testosterone level increases body fat mass, abdominal obesity, and weight gain. Therefore, this review highlights the characteristics of castrated or AR-KO mice exhibiting obesity.
AR-KO mice show characteristic late-onset obesity after puberty [Citation124–Citation126]. This obese phenotype is observed in multiple laboratories, but not in every laboratory [Citation124–Citation132]. In castration models, the obese phenotype is observed when mice are castrated before [Citation133] or after [Citation111] maturation, although controversy remains [Citation120–Citation122,Citation128,Citation134–Citation137]. These results suggest that while androgen ablation by AR-KO or castration can cause obesity in laboratory animals, androgen ablation alone may be insufficient for the development of obesity. Genomic background and/or growth environment are probably involved in these differences.
Castration-induced obesity is high-fat diet (HFD)-dependent and is due to an increase in feed efficiency [Citation111]. This increase seems to be important for the development of obesity in AR-KO mice, where food consumption is unchanged but significant body weight gain is observed [Citation124]. The AR-KO and castration models produce similar results relating body weight and body composition [Citation128]. Both body weight and food intake are decreased when androgen-ablated mice are fed standard chow. On the other hand, control and androgen-ablated mice have comparable body weights, even though food intake is decreased by approximately 25% in androgen-ablated mice receiving a HFD [Citation128], confirming an increase in feed efficiency. The HFD-fed androgen-ablated mice exhibit an increase in fat accumulation and a decrease of lean body mass, in association with glucose intolerance, insulin resistance, and fatty liver [Citation128]. These results suggest that the androgen-ablated obese phenotype is highly dependent on the type of food consumed. In addition to obesity, these finding suggest that the augmentation of feed efficiency is associated with metabolic changes in androgen-ablated mice.
Castrated obese mice have an elevated rectal body temperature [Citation111,Citation138], whereas non-obese castrated mice do not show such differences in heat generation [Citation128]. On the other hand, oxygen consumption is decreased in obese AR-KO mice [Citation126] but not in non-obese AR-KO mice or castrated mice [Citation127,Citation128]. These results suggest that a decrease in oxygen consumption, except for heat production, is involved, at least in part, in the development of obesity.
Body fat distribution is important in metabolic and CV diseases [Citation47,Citation49]. Visceral fat accumulation or increased visceral-to-subcutaneous fat ratio increases the risk for CV disease. AR expression is observed in both visceral and subcutaneous white adipose tissue, and its expression is higher in visceral fat than in subcutaneous fat [Citation139]. These results suggest that fat tissue is a direct target of androgen–AR signaling. The obese phenotype in androgen-ablated mice is accompanied by excess accumulation of visceral fat [Citation111,Citation124–Citation126]. In addition, mice lacking AR function due to a deletion of its DNA-binding domain (using Artm1Jdz floxed strain) exhibit late-onset catch-up body weight gain and exceed the body weight of the control group; these changes involve an increase in visceral fat levels [Citation127]. In contrast, the accumulation of subcutaneous fat mass is observed in androgen ablation, irrespective of body weight [Citation111,Citation124,Citation126–Citation128,Citation136]. It seems likely that the accumulation of visceral fat, but not of subcutaneous fat, is associated with androgen ablation-induced obesity and metabolic disorder.
Adipose tissue-specific AR-KO mice receiving a standard diet do not develop obesity or visceral fat accumulation [Citation96,Citation97]. When these adipocyte AR-KO mice are fed with a HFD, they accumulate visceral fat but are not overweight [Citation97], suggesting that an increase in visceral fat mass by AR-KO is insufficient to induce weight-gain. In contrast, liver-specific AR-KO mice are overweight and also develop fatty liver and visceral obesity in an HFD-dependent manner [Citation95]. In the absence of an increased food intake, neuronal AR-KO also produces late-onset obesity, with visceral fat and liver triglyceride accumulation [Citation140], although inconsistencies remain [Citation141,Citation142]. Fatty liver is also observed in mice with obesity induced by castration [Citation111] or global AR-KO [Citation125]; whereas, an obese phenotype also occurs by other AR-KO mice without incidence of fatty liver [Citation126]. Although there is no consensus among these results, it is likely that visceral fat accumulation is necessary and that the liver contributes to low androgen-induced obesity. In addition, fatty liver might be associated with the development of impaired fasting glucose.
A decrease in lipolysis, rather than an increase in lipogenesis, can cause the accumulation of visceral (mesenteric and intraperitoneal) fat. The expression of adipocyte triglyceride lipase [Citation111], hormone-sensitive lipase [Citation111,Citation126], and lipoprotein lipase [Citation126] are suppressed in visceral fat from castrated or global AR-KO obese mice. In contrast, increasing lipogenesis and free fatty acid transport appears to be responsible for androgen deficiency-induced triglyceride accumulation in the liver [Citation111,Citation143]. An increased expression of lipogenic genes is observed in the liver of hepatocyte AR-KO mice [Citation95] and in neuronal AR-KO mice [Citation140]. These results suggest that both direct and indirect androgen effects are involved in the regulation of lipogenic gene expression in the liver, and that androgens differentially regulate lipid metabolism in visceral adipose tissue and the liver.
The castration-induced obese phenotype is disappeared when mice were treated with antibiotics, suggesting the involvement of gut microbiota [Citation111,Citation138]. The gut microbiota is strongly associated with obesity [Citation144–Citation147]. Recently, androgen effects on gut microbiota have become increasingly apparent [Citation148–Citation151] and are involved in the sex bias of some diseases. It is possible that indirect androgen effects on the gut microbiome are stronger than the direct effects on visceral fat regulation.
Muscle and lean body mass
Thigh circumference is negatively associated with the prevalence of CV morbidity and mortality [Citation152], suggesting the importance of thigh muscle mass. The muscle-specific AR-KO did not show a loss of quadricep mass [Citation98,Citation153], whereas global AR-KO mice show this phenotype [Citation129,Citation154]. In addition, global AR-KO mice showed inferior limb decreases (i.e., gastrocnemius and soleus) [Citation131]. On the other hand, skeletal muscle myofiber AR-KO mice showed decreases [Citation98] or no effect [Citation153] on inferior limb mass. Muscle satellite cell-specific AR-KO also did not affect the inferior muscle mass [Citation155]. Castration-dependent muscle loss is more prominent in non-postural muscles than in the postural muscle of the forelimb, whereas similar reductions in muscle mass are observed in both the postural and non-postural muscles of the hindlimb [Citation133]. On the other hand, castrated and global AR-KO mice show decreases in relative lean mass in a HFD-dependent manner [Citation128]. Castration induced the loss of thigh muscle (quadriceps and hamstring) mass in a HFD-dependent manner; however, the loss of thigh muscle was not induced by castration when the mice were administered antibiotics [Citation111]. Taken together, inferior limb and thigh muscle may represent weak and/or indirect androgen target, whereas the levator ani is a strong and direct androgen target [Citation98]. Gut microbiota is involved in muscle loss [Citation156], while it remains unclear whether changes in the gut environment are involved in low testosterone-associated loss of lean body mass.
Insulin sensitivity
Men have lower blood adiponectin levels than women [Citation157–Citation160]. Increased adiponectin levels can alleviate insulin resistance. Inconsistent effects of androgen on adiponectin levels have been reported. Both negative [Citation138,Citation158,Citation161] and positive [Citation128,Citation159,Citation160], associations with testosterone levels have been reported. A similar lack of consistency is present in findings in AR-KO male mice [Citation125–Citation127].
Non-obese castrated rats show exacerbated hepatic and extra-hepatic (including muscle) insulin resistance, which increases the fasting glucose level [Citation162]. Obese AR-KO or castrated mice showed a decreased [Citation95,Citation125,Citation140] or unchanged [Citation111,Citation126] insulin sensitivity. In the latter cases, adiponectin levels were increased by androgen deficiency [Citation111,Citation126]. Non-obese AR-KO and castrated mice exhibited decreased [Citation128] or unchanged [Citation127] insulin sensitivity and decreased [Citation128] or increased [Citation127] adiponectin levels, respectively. Therefore, the regulation of adiponectin by androgen deficiency may affect insulin sensitivity. In addition, it is unlikely that insulin sensitivity due to androgen ablation is associated with obesity.
Insulin secretion by pancreatic β-cells
Pancreatic β-cells are responsible for the production and secretion of insulin, which reduces blood glucose concentrations. Therefore, the maintenance of insulin secretion by β-cells is important for the prevention of T2DM. The amount of insulin secreted depends on β-cell mass and β-cell function (i.e., the ability of each β-cell to secrete insulin in response to glucose); reductions in either these variable can contribute to the development of T2DM [Citation163–Citation166]. Androgen signaling seems to serve different roles in β-cell function in a species-dependent manner [Citation103,Citation167]. β-Cell AR-KO male mice exhibited reduced insulin secretion, without a loss of β-cell mass, and HFD-dependent glucose intolerance [Citation102]. In mice, the extranuclear AR stimulates glucagon-like peptide-1 signaling, leading to an enhancement of insulin secretion. In male rats, the AR is predominantly localized in the nucleus [Citation103,Citation168] and increases β-cell mass both by increasing β-cell growth and reducing β-cell death [Citation103]. Ten weeks after castration, the β-cell mass was reduced to 30% of that observed in control rats and an excessive rise in glucose levels was observed 30 min after glucose injection [Citation103]. This type of glucose intolerance reflects impairment of β-cell function [Citation166], and castration has been shown to decrease insulin levels following a glucose load in male rats [Citation162]. Testosterone also increases insulin expression [Citation169] and suppresses the β-cell death induced by streptozotocin in male rats [Citation170,Citation171]. In contrast to rats, castration does not affect β-cell mass in male mice [Citation103], and it decreases streptozotocin-induced cell-death [Citation172,Citation173]. These differences may be attributed to the different subcellular localization of β-cell AR in mice and rats [Citation103]. Collectively, β-cell androgen signaling contributes to insulin secretion and prevents T2DM development both in male rats and in male mice. In β-cells, key signaling molecules involved in insulin secretion and β-cell development, such as the glucagon-like peptide-1 receptor, insulin receptor β, PDX-1, and MafA are decreased by hyperglycemia [Citation174–Citation179]. β-Cell AR protein is also degraded by the ubiquitin-proteasome system under chronic high-glucose conditions [Citation103], suggesting the attenuation of androgen signaling under pathologic conditions. Therefore, hyperglycemia-induced AR degradation may contribute to β-cell failure. Although extranuclear AR is observed in human β-cells [Citation102], detailed analyses of AR localization at different life stages, or for a range of genetic backgrounds, are desirable because the β-cell mass is almost completely formed by the age of 20 years old in humans.
Summary of rodent studies
In mice, castration and AR-KO do not consistently produce obesity and glucose intolerance. Unlike the hyperphagia caused by ovariectomy in females [Citation180], a decrease in food intake was caused by a loss of androgen function and this could at least partially explain the failure to cause metabolic diseases in rodents. However, several rodent studies found that androgen deficiency induced metabolic dysfunction. In some models, administration of a HFD is required for the induction of metabolic dysfunction in androgen-ablated mice. Competition or compensation between androgen signaling and glucocorticoid signaling may be involved in metabolic disorders [Citation181]. Apart from the direct effects of androgens on β-cells and hepatocytes, indirect effects seemed to result in an increase of adipose tissue mass, fatty liver, or loss of muscle mass. Future studies are needed to clarify the mechanisms by which androgen deficiency induces metabolic disorders.
Acknowledgments
I am extremely grateful to Professor Emeritus Yoshihisa Nakano (Osaka Prefecture University), Professor Hiroshi Inui (Osaka Prefecture University), and Professor Ryoichi Yamaji (Osaka Prefecture University) for their continuous support and encouragement.
Disclosure statement
No potential conflict of interest was reported by the author.
Additional information
Funding
References
- Ellis L. Evolutionary Neuroandrogenic Theory and Universal Gender Differences in Cognition and Behavior. Sex Roles. 2011;64(9–10):707–722.
- Clarkson J, Herbison AE. Hypothalamic control of the male neonatal testosterone surge. Philos Trans R Soc Lond B Biol Sci. 2016;371(1688):20150115.
- Zambrano E, Guzmán C, Rodríguez-González GL, et al. Fetal programming of sexual development and reproductive function. Mol Cell Endocrinol. 2014;382(1):538–549.
- Tapanainen J, Kellokumpu-Lehtinen P, Pelliniemi L, et al. Age-related changes in endogenous steroids of human fetal testis during early and midpregnancy. J Clin Endocrinol Metab. 1981;52(1):98–102.
- Siiteri PK, Wilson JD. Testosterone formation and metabolism during male sexual differentiation in the human embryo. J Clin Endocrionol Metab. 1974;38(1):113–125.
- Christine Knickmeyer R, Baron-Cohen S. Fetal testosterone and sex differences. Early Hum Dev. 2006;82(12):755–760.
- Forest MG, De Peretti E, Bertrand J. Hypothalamic-pituitary-gonadal relationships in man from birth to puberty. Clin Endocrinol. 1976;5(5):551–569.
- Ward IL, Ward OB, Affuso JD, et al. Fetal testosterone surge: specific modulations induced in male rats by maternal stress and/or alcohol consumption. Horm Behav. 2003;43(5):531.
- Habert R, Picon R. Testosterone, dihydrotestosterone and estradiol-17 beta levels in maternal and fetal plasma and in fetal testes in the rat. J Steroid Biochem. 1984;21(2):193–198.
- O’Shaughnessy PJ, Baker PJ, Johnston H. The foetal Leydig cell– differentiation, function and regulation. Int J Androl. 2006;29(1):90–95. discussion 105-108.
- Welsh M, Saunders PT, Fisken M, et al. Identification in rats of a programming window for reproductive tract masculinization, disruption of which leads to hypospadias and cryptorchidism. J Clin Invest. 2008;118(4):1479–1490.
- Suzuki K, Ogino Y, Murakami R, et al. Embryonic development of mouse external genitalia: insights into a unique mode of organogenesis. Evol Dev. 2002;4(2):133–141.
- Corbier P, Edwards DA, Roffi J. The neonatal testosterone surge: a comparative study. Arch Int Physiol Biochim Biophys. 1992;100(2):127–131.
- Ward OB, Ward IL, Denning JH, et al. Postparturitional testosterone surge in male offspring of rats stressed and/or fed ethanol during late pregnancy. Horm Behav. 2002;41(2):229–235.
- Starka L, Pospisilova H, Hill M. Free testosterone and free dihydrotestosterone throughout the life span of men. J Steroid Biochem Mol Biol. 2009;116(1–2):118–120.
- Silva MA, Villasenor RMV, Marquez SR, et al. Testosterone Levels and Development of the Penile Spines and Testicular Tissue during the Postnatal Growth in Wistar Rats. Adv Sex Med. 2013;3:1–9.
- Reckelhoff JF, Zhang H, Granger JP. Testosterone exacerbates hypertension and reduces pressure-natriuresis in male spontaneously hypertensive rats. Hypertension. 1998;31(1 Pt 2):435–439.
- Wu X, Arumugam R, Zhang N, et al. Androgen profiles during pubertal Leydig cell development in mice. Reproduction. 2010;140(1):113–121.
- Kaufman JM, Vermeulen A. The decline of androgen levels in elderly men and its clinical and therapeutic implications. Endocr Rev. 2005;26(6):833–876.
- Wu FC, Tajar A, Beynon JM, et al. Identification of late-onset hypogonadism in middle-aged and elderly men. New Engl J Med. 2010;363(2):123–135.
- Liu PY, Death AK, Handelsman DJ. Androgens and cardiovascular disease. Endocr Rev. 2003;24:313–340.
- The coronary drug project. Design, methods, and baseline results. Circulation. 1973;47(3Suppl):I1–50.
- Laughlin GA, Barrett Connor E, Bergstrom J. Low serum testosterone and mortality in older men. J Clin Endocrinol Metab. 2008;93:68–75.
- Khaw KT, Dowsett M, Folkerd E, et al. Endogenous testosterone and mortality due to all causes, cardiovascular disease, and cancer in men: european prospective investigation into cancer in Norfolk (EPIC-Norfolk) Prospective Population Study. Circulation. 2007;116:2694–2701.
- Araujo AB, Dixon JM, Suarez EA, et al. Clinical review: endogenous testosterone and mortality in men: a systematic review and meta-analysis. J Clin Endocrinol Metab. 2011;96(10):3007–3019.
- Corona G, Rastrelli G, Monami M, et al. Hypogonadism as a risk factor for cardiovascular mortality in men: a meta-analytic study. Eur J Endocrinol. 2011;165(5):687–701.
- Ruige JB, Mahmoud AM, De Bacquer D, et al. Endogenous testosterone and cardiovascular disease in healthy men: a meta-analysis. Heart. 2011;97(11):870–875.
- Bhasin S, Cunningham GR, Hayes FJ, et al. Testosterone therapy in men with androgen deficiency syndromes: an Endocrine Society clinical practice guideline. J Clin Endocrinol Metab. 2010;95(6):2536–2559.
- Isidori AM, Giannetta E, Greco EA, et al. Effects of testosterone on body composition, bone metabolism and serum lipid profile in middle-aged men: a meta-analysis. Clin Endocrinol. 2005;63(3):280–293.
- Elliott J, Kelly SE, Millar AC, et al. Testosterone therapy in hypogonadal men: a systematic review and network meta-analysis. BMJ Open. 2017;7(11):e015284.
- Shoskes DA, Hakim LS. Testosterone replacement therapy. Transl Androl Urol. 2016;5(6):812–813.
- Comhaire F. Hormone replacement therapy and longevity. Andrologia. 2016;48(1):65–68.
- Shores MM, Smith NL, Forsberg CW, et al. Testosterone treatment and mortality in men with low testosterone levels. J Clin Endocrinol Metab. 2012;97(6):2050–2058.
- Corona G, Maseroli E, Rastrelli G, et al. Cardiovascular risk associated with testosterone-boosting medications: a systematic review and meta-analysis. Expert Opin Drug Saf. 2014;13(10):1327–1351.
- Corona GG, Rastrelli G, Maseroli E, et al. Testosterone Replacement Therapy and Cardiovascular Risk: a Review. World J Mens Health. 2015;33(3):130–142.
- Fernandez-Balsells MM, Murad MH, Lane M, et al. Clinical review 1: adverse effects of testosterone therapy in adult men: a systematic review and meta-analysis. J Clin Endocrinol Metab. 2010;95(6):2560–2575.
- Calof OM, Singh AB, Lee ML, et al. Adverse events associated with testosterone replacement in middle-aged and older men: a meta-analysis of randomized, placebo-controlled trials. J Gerontol A Biol Sci Med Sci. 2005;60(11):1451–1457.
- Haddad RM, Kennedy CC, Caples SM, et al. Testosterone and cardiovascular risk in men: a systematic review and meta-analysis of randomized placebo-controlled trials. Mayo Clin Proc. 2007;82(1):29–39.
- Xu L, Freeman G, Cowling BJ, et al. Testosterone therapy and cardiovascular events among men: a systematic review and meta-analysis of placebo-controlled randomized trials. BMC Med. 2013;11:108.
- Borst SE, Shuster JJ, Zou B, et al. Cardiovascular risks and elevation of serum DHT vary by route of testosterone administration: a systematic review and meta-analysis. BMC Med. 2014;12:211.
- FDA. FDA Drug Safety Communication: FDA cautions about using testosterone products for low testosterone due to aging; requires labeling change to inform of possible increased risk of heart attack and stroke with use. 2014 Available from: https://wwwfdagov/Drugs/DrugSafety/ucm436259htm.
- Health Canada. Information Update - Possible cardiovascular problems associated with testosterone products. Available from: 2014. http://healthycanadiansgcca/recall-alert-rappel-avis/hc-sc/2014/40587a-engphp.
- Goodman N, Guay A, Dandona P, et al. American Association Of Clinical Endocrinologists And American College Of Endocrinology Position Statement On The Association Of Testosterone And Cardiovascular Risk. Endocr Pract. 2015;21(9):1066–1073.
- European Medicine Agency. PRAC review does not confirm increase in heart problems with testosterone medicines. 2014 Available from: http://wwwemaeuropaeu/ema/indexjsp?curl=pages/news_and_events/news/2014/10/news_detail_002187jsp&mid=WC0b01ac058004d5c1.
- Corona G, Giagulli VA, Maseroli E, et al. Therapy Of Endocrine Disease: testosterone supplementation and body composition: results from a meta-analysis study. Eur J Endocrinol. 2016;174(3):R99–R116.
- Sharma R, Oni OA, Gupta K, et al. Normalization of testosterone level is associated with reduced incidence of myocardial infarction and mortality in men. Eur Heart J. 2015;36(40):2706–2715.
- Alberti KG, Eckel RH, Grundy SM, et al. Harmonizing the metabolic syndrome: a joint interim statement of the International Diabetes Federation Task Force on Epidemiology and Prevention; National Heart, Lung, and Blood Institute; American Heart Association; World Heart Federation; International Atherosclerosis Society; and International Association for the Study of Obesity. Circulation. 2009;120(16):1640–1645.
- Tchernof A, Despres JP. Pathophysiology of human visceral obesity: an update. Physiol Rev. 2013;93(1):359–404.
- Despres JP, Lemieux I. Abdominal obesity and metabolic syndrome. Nature. 2006;444(7121):881–887.
- Corona G, Monami M, Rastrelli G, et al. Testosterone and metabolic syndrome: a meta-analysis study. J Sex Med. 2011;8(1):272–283.
- Brand JS, Rovers MM, Yeap BB, et al. Testosterone, sex hormone-binding globulin and the metabolic syndrome in men: an individual participant data meta-analysis of observational studies. PloS One. 2014;9(7):e100409.
- Brand JS, van der Tweel I, Grobbee DE, et al. Testosterone, sex hormone-binding globulin and the metabolic syndrome: a systematic review and meta-analysis of observational studies. Int J Epidemiol. 2011;40(1):189–207.
- Corona G, Rastrelli G, Maggi M. Diagnosis and treatment of late-onset hypogonadism: systematic review and meta-analysis of TRT outcomes. Best Pract Res Clinical Endocrinol Metab. 2013;27(4):557–579.
- Ding EL, Song Y, Malik VS, et al. Sex differences of endogenous sex hormones and risk of type 2 diabetes: a systematic review and meta-analysis. JAMA. 2006;295(11):1288–1299.
- Corona G, Monami M, Rastrelli G, et al. Type 2 diabetes mellitus and testosterone: a meta-analysis study. Int J Androl. 2011;34(6 Pt 1):528–540.
- Kelly DM, Jones TH. Testosterone and obesity. Obes Rev. 2015;16(7):581–606.
- Whitsel EA, Boyko EJ, Matsumoto AM, et al. Intramuscular testosterone esters and plasma lipids in hypogonadal men: a meta-analysis. Am J Med. 2001;111(4):261–269.
- Cai X, Tian Y, Wu T, et al. Metabolic effects of testosterone replacement therapy on hypogonadal men with type 2 diabetes mellitus: a systematic review and meta-analysis of randomized controlled trials. Asian J Androl. 2014;16(1):146–152.
- Grossmann M, Hoermann R, Wittert G, et al. Effects of testosterone treatment on glucose metabolism and symptoms in men with type 2 diabetes and the metabolic syndrome: a systematic review and meta-analysis of randomized controlled clinical trials. Clin Endocrinol. 2015;83(3):344–351.
- Corona G, Giagulli VA, Maseroli E, et al. Testosterone supplementation and body composition: results from a meta-analysis of observational studies. J Endocrinol Invest. 2016;39(9):967–981.
- Siegel RL, Miller KD, Jemal A. Cancer statistics. CA Cancer J Clin. 2018;68(1):7–30.
- Keating NL, O’Malley AJ, Smith MR. Diabetes and cardiovascular disease during androgen deprivation therapy for prostate cancer. J Clin Oncol. 2006;24(27):4448–4456.
- Nguyen PL, Je Y, Schutz FA, et al. Association of androgen deprivation therapy with cardiovascular death in patients with prostate cancer: a meta-analysis of randomized trials. JAMA. 2011;306(21):2359–2366.
- Zhao J, Zhu S, Sun L, et al. Androgen deprivation therapy for prostate cancer is associated with cardiovascular morbidity and mortality: a meta-analysis of population-based observational studies. PloS One. 2014;9(9):e107516.
- Bosco C, Bosnyak Z, Malmberg A, et al. Quantifying observational evidence for risk of fatal and nonfatal cardiovascular disease following androgen deprivation therapy for prostate cancer: a meta-analysis. Eur Urology. 2015;68(3):386–396.
- Meng F, Zhu S, Zhao J, et al. Stroke related to androgen deprivation therapy for prostate cancer: a meta-analysis and systematic review. BMC Cancer. 2016;16:180.
- Haseen F, Murray LJ, Cardwell CR, et al. The effect of androgen deprivation therapy on body composition in men with prostate cancer: systematic review and meta-analysis. J Cancer Surviv. 2010;4(2):128–139.
- Wang H, Sun X, Zhao L, et al. Androgen deprivation therapy is associated with diabetes: evidence from meta-analysis. J Diabetes Investig. 2016;7(4):629–636.
- Bosco C, Crawley D, Adolfsson J, et al. Quantifying the evidence for the risk of metabolic syndrome and its components following androgen deprivation therapy for prostate cancer: a meta-analysis. PloS One. 2015;10(3):e0117344.
- Diver MJ, Imtiaz KE, Ahmad AM, et al. Diurnal rhythms of serum total, free and bioavailable testosterone and of SHBG in middle-aged men compared with those in young men. Clin Endocrinol. 2003;58(6):710–717.
- Wehr E, Pilz S, Boehm BO, et al. Association of vitamin D status with serum androgen levels in men. Clin Endocrinol. 2010;73(2):243–248.
- Blouin K, Boivin A, Tchernof A. Androgens and body fat distribution. J Steroid Biochem Mol Biol. 2008;108(3–5):272–280.
- Shores MM, Biggs ML, Arnold AM, et al. Testosterone, dihydrotestosterone, and incident cardiovascular disease and mortality in the cardiovascular health study. J Clin Endocrinol Metab. 2014;99(6):2061–2068.
- Shores MM, Arnold AM, Biggs ML, et al. Testosterone and dihydrotestosterone and incident ischaemic stroke in men in the Cardiovascular Health Study. Clin Endocrinol. 2014;81(5):746–753.
- Basaria S, Coviello AD, Travison TG, et al. Adverse events associated with testosterone administration. New Engl J Med. 2010;363(2):109–122.
- Iwamoto T, Yanase T, Horie H, et al. Late-onset hypogonadism (LOH) and androgens: validity of the measurement of free testosterone levels in the diagnostic criteria in Japan. Int J Urol. 2009;16(2):168–174.
- Kodama K, Tojjar D, Yamada S, et al. Ethnic differences in the relationship between insulin sensitivity and insulin response: a systematic review and meta-analysis. Diabetes Care. 2013;36(6):1789–1796.
- Min KJ, Lee CK, Park HN. The lifespan of Korean eunuchs. Curr Biol. 2012;22(18):R792–R793.
- Nieschlag E, Nieschlag S, Behre HM. Lifespan and testosterone. Nature. 1993;366(6452):215.
- Zirkin BR, Tenover JL. Aging and declining testosterone: past, present, and hopes for the future. J Androl. 2012;33(6):1111–1118.
- Tajar A, Forti G, O’Neill TW, et al. Characteristics of secondary, primary, and compensated hypogonadism in aging men: evidence from the European Male Ageing Study. J Clin Endocrinol Metab. 2010;95(4):1810–1818.
- Kraemer WJ, Ratamess NA. Hormonal responses and adaptations to resistance exercise and training. Sports Med. 2005;35(4):339–361.
- Corona G, Rastrelli G, Monami M, et al. Body weight loss reverts obesity-associated hypogonadotropic hypogonadism: a systematic review and meta-analysis. Eur J Endocrinol. 2013;168(6):829–843.
- Evans RM. The steroid and thyroid hormone receptor superfamily. Science. 1988;240:889–895.
- Heemers HV, Tindall DJ. Androgen receptor (AR) coregulators: a diversity of functions converging on and regulating the AR transcriptional complex. Endocr Rev. 2007;28:778–808.
- Harada N, Yasunaga R, Higashimura Y, et al. Glyceraldehyde-3-phosphate dehydrogenase enhances transcriptional activity of androgen receptor in prostate cancer cells. J Biol Chem. 2007;282(31):22651–22661.
- Harada N, Ohmori Y, Yamaji R, et al. ARA24/Ran enhances the androgen-dependent NH2- and COOH-terminal interaction of the androgen receptor. Biochem Biophys Res Commun. 2008;373(3):373–377.
- Harada N, Yokoyama T, Yamaji R, et al. RanBP10 acts as a novel coactivator for the androgen receptor. Biochem Biophys Res Commun. 2008;368(1):121–125.
- Harada N, Takagi T, Nakano Y, et al. Protein arginine methyltransferase 10 is required for androgen-dependent proliferation of LNCaP prostate cancer cells. Biosci Biotechnol Biochem. 2015;79(9):1430–1437.
- Heinlein CA, Chang C. Androgen receptor (AR) coregulators: an overview. Endocr Rev. 2002;23:175–200.
- Wang L, Hsu CL, Chang C. Androgen receptor corepressors: an overview. Prostate. 2005;63:117–130.
- Palazzolo I, Gliozzi A, Rusmini P, et al. The role of the polyglutamine tract in androgen receptor. J Steroid Biochem Mol Biol. 2008;108(3–5):245–253.
- Harada N, Mitani T, Higashimura Y, et al. Involvement of three glutamine tracts in human androgen receptor transactivation. J Steroid Biochem Mol Biol. 2010;118(1–2):77–84.
- Heinlein CA, Chang C. Androgen receptor in prostate cancer. Endocr Rev. 2004;25:276–308.
- Lin HY, Yu IC, Wang RS, et al. Increased hepatic steatosis and insulin resistance in mice lacking hepatic androgen receptor. Hepatology. 2008;47(6):1924–1935.
- Yu IC, Lin HY, Liu NC, et al. Hyperleptinemia without obesity in male mice lacking androgen receptor in adipose tissue. Endocrinology. 2008;149(5):2361–2368.
- McInnes KJ, Smith LB, Hunger NI, et al. Deletion of the androgen receptor in adipose tissue in male mice elevates retinol binding protein 4 and reveals independent effects on visceral fat mass and on glucose homeostasis. Diabetes. 2012;61(5):1072–1081.
- Ophoff J, Van Proeyen K, Callewaert F, et al. Androgen signaling in myocytes contributes to the maintenance of muscle mass and fiber type regulation but not to muscle strength or fatigue. Endocrinology. 2009;150(8):3558–3566.
- Zeier M, Schonherr R, Amann K, et al. Effects of testosterone on glomerular growth after uninephrectomy. Nephrol Dial Transplant. 1998;13(9):2234–2240.
- Ikeda Y, Aihara K, Sato T, et al. Androgen receptor gene knockout male mice exhibit impaired cardiac growth and exacerbation of angiotensin II-induced cardiac fibrosis. J Biol Chem. 2005;280(33):29661–29666.
- Kochakian CD, Tillotson C, Endahl GL. Castration and the growth of muscles in the rat. Endocrinology. 1956;58(2):226–231.
- Navarro G, Xu W, Jacobson DA, et al. Extranuclear Actions of the Androgen Receptor Enhance Glucose-Stimulated Insulin Secretion in the Male. Cell Metabolism. 2016;23(5):837–851.
- Harada N, Yoda Y, Yotsumoto Y, et al. Androgen signaling expands beta-cell mass in male rats and beta-cell androgen receptor is degraded under high-glucose conditions. Am J Physiol Endocrinol Metab. 2017;314:E274–E286.
- De Gendt K, Verhoeven G. Tissue- and cell-specific functions of the androgen receptor revealed through conditional knockout models in mice. Mol Cell Endocrinol. 2012;352(1–2):13–25.
- Yu IC, Lin HY, Sparks JD, et al. Androgen receptor roles in insulin resistance and obesity in males: the linkage of androgen-deprivation therapy to metabolic syndrome. Diabetes. 2014;63(10):3180–3188.
- Chang C, Yeh S, Lee SO, et al. Androgen Receptor (AR) Pathophysiological Roles in Androgen Related Diseases in Skin, Bone/Muscle,Metabolic Syndrome and Neuron/Immune Systems: lessons Learned from Mice Lacking AR in Specific Cells. Nucl Recept Signal. 2013;11:e001.
- Kerkhofs S, Denayer S, Haelens A, et al. Androgen receptor knockout and knock-in mouse models. J Mol Endocrinol. 2009;42(1):11–17.
- Rana K, Davey RA, Zajac JD. Human androgen deficiency: insights gained from androgen receptor knockout mouse models. Asian J Androl. 2014;16(2):169–177.
- Chang C, Lee SO, Wang RS, et al. Androgen receptor (AR) physiological roles in male and female reproductive systems: lessons learned from AR-knockout mice lacking AR in selective cells. Biol Reprod. 2013;89(1):21.
- Verhoeven G, Willems A, Denolet E, et al. Androgens and spermatogenesis: lessons from transgenic mouse models. Philos Trans R Soc Lond B Biol Sci. 2010;365(1546):1537–1556.
- Harada N, Hanaoka R, Horiuchi H, et al. Castration influences intestinal microflora and induces abdominal obesity in high-fat diet-fed mice. Sci Rep. 2016;6:23001.
- Bourghardt J, Wilhelmson AS, Alexanderson C, et al. Androgen receptor-dependent and independent atheroprotection by testosterone in male mice. Endocrinology. 2010;151(11):5428–5437.
- Huang CK, Pang H, Wang L, et al. New therapy via targeting androgen receptor in monocytes/macrophages to battle atherosclerosis. Hypertension. 2014;63(6):1345–1353.
- Takov K, Wu J, Denvir MA, et al. The role of androgen receptors in atherosclerosis. Mol Cell Endocrinol. 2017. DOI:10.1016/j.mce.2017.10.006
- Field RA. Effect of castration on meat quality and quantity. J Anim Sci. 1971;32(5):849–858.
- Balaji N, Sivaraman T, Sivakumar T, et al. Influence of castration on growth rate and body measurements in large white yorkshire pigs. Indian J Anim Res. 2006;40(2):123–126.
- Qing Z, Xian Z, Hua Z. The effect of early castration on weight gain in male rabbits. In: Cahiers Options Mediterraneennes. 1994;8:401–405.
- Payne AP. A comparison of the effects of androstenedione, dihydrotestosterone and testosterone propionate on aggression in the castrated male golden hamster. Physiol Behav. 1974;13(1):21–26.
- Swanson HH. Effects of pre- and post-pubertal gonadectomy on sex differences in growth, adrenal and pituitary weights of hamsters. J Endocrinol. 1967;39(4):555–564.
- Gentry RT, Wade GN. Androgenic control of food intake and body weight in male rats. J Comp Physiol Psychol. 1976;90(1):18–25.
- Krotkiewski M, Kral JG, Karlsson J. Effects of castration and testosterone substitution on body composition and muscle metabolism in rats. Acta Physiol Scand. 1980;109(3):233–237.
- Inoue T, Zakikhani M, David S, et al. Effects of castration on insulin levels and glucose tolerance in the mouse differ from those in man. Prostate. 2010;70(15):1628–1635.
- Wade GN, Gray JM. Gonadal effects on food intake and adiposity: a metabolic hypothesis. Physiol Behav. 1979;22(3):583–593.
- Sato T, Matsumoto T, Yamada T, et al. Late onset of obesity in male androgen receptor-deficient (AR KO) mice. Biochem Biophys Res Commun. 2003;300:167–171.
- Lin HY, Xu Q, Yeh S, et al. Insulin and leptin resistance with hyperleptinemia in mice lacking androgen receptor. Diabetes. 2005;54(6):1717–1725.
- Fan W, Yanase T, Nomura M, et al. Androgen receptor null male mice develop late-onset obesity caused by decreased energy expenditure and lipolytic activity but show normal insulin sensitivity with high adiponectin secretion. Diabetes. 2005;54:1000–1008.
- Rana K, Fam BC, Clarke MV, et al. Increased adiposity in DNA binding-dependent androgen receptor knockout male mice associated with decreased voluntary activity and not insulin resistance. Am J Physiol Endocrinol Metab. 2011;301(5):E767–E778.
- Dubois V, Laurent MR, Jardi F, et al. Androgen Deficiency Exacerbates High-Fat Diet-Induced Metabolic Alterations in Male Mice. Endocrinology. 2016;157(2):648–665.
- Ophoff J, Callewaert F, Venken K, et al. Physical activity in the androgen receptor knockout mouse: evidence for reversal of androgen deficiency on cancellous bone. Biochem Biophys Res Commun. 2009;378(1):139–144.
- Notini AJ, Davey RA, McManus JF, et al. Genomic actions of the androgen receptor are required for normal male sexual differentiation in a mouse model. J Mol Endocrinol. 2005;35(3):547–555.
- MacLean HE, Chiu WS, Notini AJ, et al. Impaired skeletal muscle development and function in male, but not female, genomic androgen receptor knockout mice. FASEB J. 2008;22(8):2676–2689.
- Adthapanyawanich K, Kumchantuek T, Nakata H, et al. Morphology and gene expression profile of the submandibular gland of androgen-receptor-deficient mice. Arch Oral Biol. 2015;60(2):320–332.
- Ihemelandu EC, Ibebunjo C. Comparison of the effect of castration on the development of postural and non-postural muscles of mice. Acta Anat. 1992;143(4):283–288.
- Nikolaenko L, Jia Y, Wang C, et al. Testosterone replacement ameliorates nonalcoholic fatty liver disease in castrated male rats. Endocrinology. 2014;155(2):417–428.
- Rothwell NJ, Stock MJ. Energy balance and brown fat activity in adrenalectomized male, female, and castrated male rats. Metabolism. 1986;35(7):657–660.
- Christoffersen B, Raun K, Svendsen O, et al. Evalution of the castrated male Sprague-Dawley rat as a model of the metabolic syndrome and type 2 diabetes. Int J Obes. 2006;30(8):1288–1297.
- Smith MR. Changes in fat and lean body mass during androgen-deprivation therapy for prostate cancer. Urology. 2004;63(4):742–745.
- Harada N, Hanaoka R, Hanada K, et al. Hypogonadism alters cecal and fecal microbiota in male mice. Gut Microbes. 2016;7(6):533–539.
- Ibrahim MM. Subcutaneous and visceral adipose tissue: structural and functional differences. Obes Rev. 2010;11(1):11–18.
- Yu IC, Lin HY, Liu NC, et al. Neuronal androgen receptor regulates insulin sensitivity via suppression of hypothalamic NF-kappaB-mediated PTP1B expression. Diabetes. 2013;62(2):411–423.
- Raskin K, De Gendt K, Duittoz A, et al. Conditional inactivation of androgen receptor gene in the nervous system: effects on male behavioral and neuroendocrine responses. J Neurosci. 2009;29(14):4461–4470.
- Juntti SA, Tollkuhn J, Wu MV, et al. The androgen receptor governs the execution, but not programming, of male sexual and territorial behaviors. Neuron. 2010;66(2):260–272.
- Senmaru T, Fukui M, Okada H, et al. Testosterone deficiency induces markedly decreased serum triglycerides, increased small dense LDL, and hepatic steatosis mediated by dysregulation of lipid assembly and secretion in mice fed a high-fat diet. Metabolism. 2013;62(6):851–860.
- Delzenne NM, Cani PD. Interaction between obesity and the gut microbiota: relevance in nutrition. Annu Rev Nutr. 2011;31:15–31.
- Turnbaugh PJ, Ley RE, Mahowald MA, et al. An obesity-associated gut microbiome with increased capacity for energy harvest. Nature. 2006;444(7122):1027–1031.
- Lam YY, Mitchell AJ, Holmes AJ, et al. Role of the gut in visceral fat inflammation and metabolic disorders. Obesity. 2011;19(11):2113–2120.
- Ley RE, Turnbaugh PJ, Klein S, et al. Microbial ecology: human gut microbes associated with obesity. Nature. 2006;444(7122):1022–1023.
- Yurkovetskiy L, Burrows M, Khan AA, et al. Gender bias in autoimmunity is influenced by microbiota. Immunity. 2013;39(2):400–412.
- Markle JG, Frank DN, Mortin-Toth S, et al. Sex differences in the gut microbiome drive hormone-dependent regulation of autoimmunity. Science. 2013;339(6123):1084–1088.
- Org E, Mehrabian M, Parks BW, et al. Sex differences and hormonal effects on gut microbiota composition in mice. Gut Microbes. 2016;7(4):313–322.
- Moreno-Indias I, Sanchez-Alcoholado L, Sanchez-Garrido MA, et al. Neonatal androgen exposure causes persistent gut microbiota dysbiosis related to metabolic disease in adult female rats. Endocrinology. 2016;157(12):4888–4898.
- Heitmann BL, Frederiksen P. Thigh circumference and risk of heart disease and premature death: prospective cohort study. BMJ. 2009;339:b3292.
- Chambon C, Duteil D, Vignaud A, et al Myocytic androgen receptor controls the strength but not the mass of limb muscles. Proc Natl Acad Sci U S A. 2010;107(32):14327–14332.
- Callewaert F, Venken K, Ophoff J, et al. Differential regulation of bone and body composition in male mice with combined inactivation of androgen and estrogen receptor-alpha. FASEB J. 2009;23(1):232–240.
- Dubois V, Laurent MR, Sinnesael M, et al. A satellite cell-specific knockout of the androgen receptor reveals myostatin as a direct androgen target in skeletal muscle. FASEB J. 2014;28(7):2979–2994.
- Bindels LB, Delzenne NM. Muscle wasting: the gut microbiota as a new therapeutic target?. Int J Biochem Cell Biol. 2013;45(10):2186–2190.
- Kadowaki T, Yamauchi T. Adiponectin and adiponectin receptors. Endocr Rev. 2005;26(3):439–451.
- Nishizawa H, Shimomura I, Kishida K, et al. Androgens decrease plasma adiponectin, an insulin-sensitizing adipocyte-derived protein. Diabetes. 2002;51(9):2734–2741.
- Laughlin GA, Barrett-Connor E, May S. Sex-specific association of the androgen to oestrogen ratio with adipocytokine levels in older adults: the Rancho Bernardo Study. Clin Endocrinol. 2006;65(4):506–513.
- Laughlin GA, Barrett-Connor E, May S. Sex-specific determinants of serum adiponectin in older adults: the role of endogenous sex hormones. Int J Obes. 2007;31(3):457–465.
- Lanfranco F, Zitzmann M, Simoni M, et al. Serum adiponectin levels in hypogonadal males: influence of testosterone replacement therapy. Clin Endocrinol. 2004;60(4):500–507.
- Xia F, Xu X, Zhai H, et al. Castration-induced testosterone deficiency increases fasting glucose associated with hepatic and extra-hepatic insulin resistance in adult male rats. Reprod Biol Endocrinol. 2013;11:106.
- Rhodes CJ. Type 2 diabetes-a matter of beta-cell life and death?. Science. 2005;307(5708):380–384.
- Cho JH, Kim JW, Shin JA, et al. beta-cell mass in people with type 2 diabetes. J Diabetes Investig. 2011;2(1):6–17.
- Ra D. Banting Lecture. From the triumvirate to the ominous octet: a new paradigm for the treatment of type 2 diabetes mellitus. Diabetes. 2009;58(4):773–795.
- Ferrannini E, Mari A. beta-Cell function in type 2 diabetes. Metabolism. 2014;63(10):1217–1227.
- Morimoto S, Morales A, Zambrano E, et al. Sex steroids effects on the endocrine pancreas. J Steroid Biochem Mol Biol. 2010;122(4):107–113.
- Harada N, Katsuki T, Takahashi Y, et al. Androgen receptor silences thioredoxin-interacting protein and competitively inhibits glucocorticoid receptor-mediated apoptosis in pancreatic β-cells. J Cell Biochem. 2015;116(6):998–1006.
- Morimoto S, Fernandez-Mejia C, Romero-Navarro G, et al. Testosterone effect on insulin content, messenger ribonucleic acid levels, promoter activity, and secretion in the rat. Endocrinology. 2001;142(4):1442–1447.
- Morimoto S, Mendoza-Rodriguez CA, Hiriart M, et al. Protective effect of testosterone on early apoptotic damage induced by streptozotocin in rat pancreas. J Endocrinol. 2005;187(2):217–224.
- Palomar-Morales M, Morimoto S, Mendoza-Rodriguez CA, et al. The protective effect of testosterone on streptozotocin-induced apoptosis in beta cells is sex specific. Pancreas. 2010;39(2):193–200.
- Rossini AA, Williams RM, Appel MC, et al. Sex differences in the multiple-dose streptozotocin model of diabetes. Endocrinology. 1978;103(4):1518–1520.
- Li RJ, Qiu SD, Wang HX, et al. Androgen receptor: a new player associated with apoptosis and proliferation of pancreatic beta-cell in type 1 diabetes mellitus. Apoptosis. 2008;13(8):959–971.
- Xu G, Kaneto H, Laybutt DR, et al. Downregulation of GLP-1 and GIP receptor expression by hyperglycemia: possible contribution to impaired incretin effects in diabetes. Diabetes. 2007;56(6):1551–1558.
- Otani K, Kulkarni RN, Baldwin AC, et al. Reduced beta-cell mass and altered glucose sensing impair insulin-secretory function in betaIRKO mice. Am J Physiol Endocrinol Metab. 2004;286(1):E41–E49.
- Hribal ML, Perego L, Lovari S, et al. Chronic hyperglycemia impairs insulin secretion by affecting insulin receptor expression, splicing, and signaling in RIN beta cell line and human islets of Langerhans. FASEB J. 2003;17(10):1340–1342.
- Vanderford NL. Regulation of beta-cell-specific and glucose-dependent MafA expression. Islets. 2011;3(1):35–37.
- Chen F, Sha M, Wang Y, et al. Transcription factor Ets-1 links glucotoxicity to pancreatic beta cell dysfunction through inhibiting PDX-1 expression in rodent models. Diabetologia. 2016;59(2):316–324.
- Wajchenberg BL. beta-cell failure in diabetes and preservation by clinical treatment. Endocr Rev. 2007;28(2):187–218.
- Brown LM, Clegg DJ. Central effects of estradiol in the regulation of food intake, body weight, and adiposity. J Steroid Biochem Mol Biol. 2010;122(1–3):65–73.
- Harada N, Inui H, Yamaji R. Competitive and compensatory effects of androgen signaling and glucocorticoid signaling. Receptors Clin Investig. 2015;2(3):e785.