Abstract
The use of simulated biological fluids (SBFs) is a promising in vitro technique to better understand the release mechanisms and possible in vivo behaviour of materials, including fibres, metal-containing particles and nanomaterials. Applications of SBFs in dissolution tests allow a measure of material biopersistence or, conversely, bioaccessibility that in turn can provide a useful inference of a materials biodistribution, its acute and long-term toxicity, as well as its pathogenicity. Given the wide range of SBFs reported in the literature, a review was conducted, with a focus on fluids used to replicate environments that may be encountered upon material inhalation, including extracellular and intracellular compartments. The review aims to identify when a fluid design can replicate realistic biological conditions, demonstrate operation validation, and/or provide robustness and reproducibility. The studies examined highlight simulated lung fluids (SLFs) that have been shown to suitably replicate physiological conditions, and identify specific components that play a pivotal role in dissolution mechanisms and biological activity; including organic molecules, redox-active species and chelating agents. Material dissolution was not always driven by pH, and likewise not only driven by SLF composition; specific materials and formulations correspond to specific dissolution mechanisms. It is recommended that SLF developments focus on biological predictivity and if not practical, on better biological mimicry, as such an approach ensures results are more likely to reflect in vivo behaviour regardless of the material under investigation.
Introduction
It is well known within particle and fibre toxicology that the onset of disease can be dictated by the residence time and/or behaviour of exogenous material within a biological environment, for inhaled materials this would be the lungs. With the development of new and advanced materials comes a responsibility to evaluate their safety regarding human exposure. Traditional and regulatory accepted methods to do this involve costly and time-consuming animal studies that often need to be carried out on a case-by-case basis due to the growing diversity and applicability of advanced materials. However, there is an increasing divergence from animal testing and promotion of the well-known “three R’s” principle which advocates the Replacement, Reduction and Refinement of alternatives to animal testing (Abollino et al. Citation2018). Within Europe, companies have a legal obligation under REACH to try to use alternative methods and approaches before relying on animal studies (ECHA Citation2016). As the demand for risk and hazard assessment of materials increases, there is a growing need for robust in vitro and in chemico approaches for toxicological evaluation. New Approach Methodologies (NAMs) – defined by ECHA “to include in silico approaches, in chemico and in vitro assays, as well as the inclusion of information from the exposure of chemicals in the context of hazard assessment” – are being developed to help fill these gaps.
Dissolution in the context of toxicity
When discussing material pathogenicity, and how this is impacted by solubility, it is important to distinguish between the different modes of toxicity, principally (i) biodurability and (ii) bioaccessibility. Biodurability is a measure of particle persistence, where materials that are found to resist physical or chemical clearance are more likely to result in long-term adverse effects. Conversely, bioaccessibility relates to the release of materials’ soluble components, where toxicity is a result of these being accessible to a designated body or cellular compartment. In this way, pathogenicity can be a result of both poor and good solubility depending on the material characteristics. At this point, it is useful to define each of these in full and discuss how they are measured for regulatory purposes.
Biodurability
Biodurability is defined as ‘the extent to which particles and fibres are able to resist chemical, physical and other physiological clearance mechanisms within the body’ (Jurinski and Rimstidt Citation2001; Utembe et al. Citation2015). Resistance to physical clearance or chemical dissolution in the lung environment is primarily dictated by the materials chemistry and geometry, and how the host system exploits these parameters. For fibres, the geometry is particularly important as this not only determines the ability to enter the respiratory system but also dictates where deposition occurs within the lung and how fibres are treated by cells. The durability of long fibres (>20 µm) is of particular concern as these are not readily cleared by cellular processes, and in some cases has been shown to cause inflammation and frustrated phagocytosis (Dörger et al. Citation2001; Boyles et al. Citation2015b), as shown in .
Figure 1. Schematic highlighting the pathogenicity pathways of (a) fibres inside the alveolar space interacting with macrophages; (b) poorly soluble metals remaining in the alveolar space, alongside transfer through cellular mechanisms; and (c, d) soluble metals in intra- and extra-cellular regions.
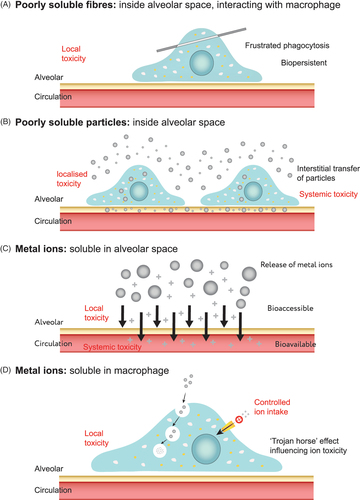
Within the EU, harmonised classifications exist for fibres dependent on their chemical compositions under Annex VI of the Classification, Labelling and Packaging Regulation (Regulation (EC) No 1272/2008; CLP). In particular, the content of alkaline/alkali earth oxides is used to determine if a class 1B or class 2 carcinogen classification should be applied; the former applying to those with an alkaline/alkali earth oxides content less than or equal to 18% weight percent, and the latter relating to those with greater than 18%. However, in order to demonstrate that fibre is not carcinogenic, current regulatory accepted methods (under Note Q of CLP) require in vivo studies to be performed, and involve short-term biopersistence tests by either inhalation, intratracheal or intra-peritoneal instillation, as well as the absence of pathogenicity in a long-term inhalation test. At present, in vitro methods which can corroborate these outcomes, such as those utilising simulated biological fluids (SBFs), are not incorporated into regulatory frameworks. Nonetheless there is a concerted effort to promote alternatives to animal tests in the regulatory environment.
Bioaccessibility
It is also important to understand the effect of the components released from materials that are susceptible to dissolution. In vitro bioelution studies, which provide information about bioaccessibility, are a first step in quantifying the release of ions within a biologically relevant media. This is particularly important for metal- and mineral-containing materials, where pathogenicity is largely related to pulmonary solubility and subsequent systemic uptake (Ellingsen et al. Citation2013); as well as for nanomaterials, where the development of reliable and robust methods for determining particle dissolution rates is considered paramount for enabling grouping and read-across approaches (Koltermann-Jülly et al. Citation2018), and would allow for a much needed distinction between rapidly dissolving and poorly soluble nanomaterials (Keller et al. Citation2020). For metal (zero valent), or metal-containing materials, it is this release of metal ions which is considered the key driving force behind any induced toxicity ().
The importance of solubility is recognised by IARC, and relates both to local effects as well as absorption and subsequent systemic effects at secondary sites (Heim et al. Citation2020). However, in vitro dissolution can be over-interpreted, particularly when excessive release of metal ions occurs, as bioaccessibility does not automatically imply bioavailability (Henderson et al. Citation2014). It should be noted that in vitro and in chemico bioaccessibility assays give an indication of bioavailability but do not actually measure such; absorption is not measured and speciation is often not identified. Even with these concerns, the use of in vitro tools are proposed (Heim et al. Citation2020) to determine bioaccessibility of metals and to provide risk assessment information for EU CLP regulation. Within the current regulation, a hazard associated to a mixture (including metal alloys which are considered as mixtures for the purposes of CLP) is defined by the concentration of individual materials and the ratio/percentage held within this mixture. However, the release kinetics in physiological environments may not be as straight forward as this and a relative bioaccessible concentration is thought to provide more accurate and useful information when predicting a hazard.
For each of these concerns, the simulation of body compartments and application of SBFs may prove advantageous. It should be noted that for the remainder of this review, unless specifically defined, a generalised term of “particle” or “material” is used to encompass all geometries, dimensions and aspect ratios, and therefore covers materials of any shape (e.g. spherical, irregular, fibrous, platelet), including fibres, metal-containing particles and nanomaterials (encompassing nanoparticles (NPs) and nanofibers). This is because SBFs and biodissolution studies can be used to understand both biodurability and bioaccessibility. However, it is important to keep in mind that the different modes of toxicity will influence how the literature is discussed; in some instances the concern is around persistence, and in others, the toxicity of released components. For the purposes of this review fibres are defined as particles while have a maximum width less than 3 µm, a length greater than 5 µm and an aspect ratio greater than 3:1 based on WHO (Citation1997) guidelines. Likewise, following the European Commission recommendation, a nanomaterial is a particle (natural, incidental or manufactured) with one or more external dimensions in the size range of 1–100 nm (EC Citation2011). The focus of this review remains on the simulation of environments encountered by inhaled materials within the lung, and as such, many of the observations can be applied to all inhaled materials.
General use of simulated biological fluids
The use of SBFs in in vitro solubility assessment of materials is a promising technique which can allow for a better understanding of the materials behaviour within biological environments, helping to determine durability (biodurability), release mechanisms (bioaccessibility) and potentially to predict in vivo behaviour. Such information can provide a particularly useful level of predictive power, allowing inference of a materials toxicity (Mukhtar and Limbeck Citation2013).
In the replication of biological fluids, the generalised term of SBFs is often used, as it is in this review. However, this should not be confused with simulated body fluid – this is a specific body fluid and a mimic of human blood plasma used in the testing of biomaterials and implants – which goes by the same abbreviation (Kokubo and Takadama Citation2006). The focus of this review will be on use of simulated lung fluids (SLFs), a specific group of SBFs, which attempt to replicate the environments encountered upon respiration of particles or fibres. When used in dissolution tests SLFs could be an effective alternative to in vivo studies, if results are predictive, reliable and reproducible.
Simulated biological fluid specificity to environments
The main objective of SBFs is to accurately mimic the in vivo behaviour of a material within a relevant biological compartment, and will depend upon the route of exposure, administration or translocation. Primarily, this focuses on the fluid’s composition and its ability to replicate the physiological conditions. The first SBF was presented in 1976 by J. L. Gamble as a description of extracellular fluid of the skeletal muscle. Over the years, this has been refined to distinguish between different extracellular compartments, and modified to mimic intracellular fluids. Specific routes of entry into the body include the lung, dermis, eyes or gastrointestinal tract (GIT). In turn, SBFs have been fashioned to model specific compartments/environments such as the lung lining fluid, mucus, sweat, saliva and tears, to name but a few. depicts how complex and diverse the requirements are for accurate simulation of these various environments, as well as highlighting how dependent the route of exposure is on which compartments a material may encounter.
Figure 2. Schematic demonstrating the diversity of simulated biological fluids and the differences in their composition and physiochemical properties. In particular, the alveoli and lysosome have been highlighted indicating the locations and characteristics of the lysosomal fluid, lung lining fluid and interstitial fluid. [adapted with permission from (Plumlee et al. Citation2006)].
![Figure 2. Schematic demonstrating the diversity of simulated biological fluids and the differences in their composition and physiochemical properties. In particular, the alveoli and lysosome have been highlighted indicating the locations and characteristics of the lysosomal fluid, lung lining fluid and interstitial fluid. [adapted with permission from (Plumlee et al. Citation2006)].](/cms/asset/201552d2-74ff-4363-af1d-ef14a0a7ab21/itxc_a_1903386_f0002_c.jpg)
SBFs have been used to test a wide variety of phenomena including the dermal exposure to particles (Stefaniak and Harvey Citation2006), development of drug delivery systems (Frohlich Citation2017), release of metal NPs, or ions, from textiles (Balakumaran et al. Citation2016), degradation of chemical warfare agents (Karote et al. Citation2013) and the behaviour, fate and potential toxicity of inhaled substances (Eidson Citation1994; Boyles et al. Citation2019; Liu et al. Citation2019; Lammel et al. Citation2020).
The use of SBFs has been widespread in the literature (Ansoborlo et al. Citation1999; Kokubo and Takadama Citation2006; Marques et al. Citation2011; Radivojev et al. Citation2019); however, there has been a broad variety of fluids reported and often a lack of commonality, even within recent publications (Hassoun et al. Citation2018; Hu et al. Citation2019; Keller et al. Citation2020; Zhong et al. Citation2020). The study of oral exposure through the GIT has demonstrated the greatest level of harmonisation. A likely reason for this is due to oral administration being the most common and convenient method for systemic delivery of drugs (Marques et al. Citation2011). It has also been extensively studied in relation to human health risk assessment of contaminant soils, leading to the development of the Unified Bioaccessibility Method (UBM) (Wragg et al. Citation2011). The UBM is a single comprehensive and standardised in vitro method to simulate the passage of a material through various compartments in the GIT including the mouth, stomach and intestines. Simulated saliva, gastric, intestine, duodenal fluid, and bile are used to mimic these compartments and prescribed compositions are provided (Wragg et al. Citation2011). The UBM was developed further to create the Fed ORganic Estimation human Simulation Test (FOREhST) in vitro procedure for determining bioaccessibility of materials in soils (Cui et al. Citation2016). As the respiratory system also contains a variety of distinct biological compartments, the development of a similar standardised method for determining bioaccessibility could be used. However, at present there is no common or standardised methodology for inhalation investigations despite previous attempts such as that of Guldberg et al. (Citation2003). This gap has been recognised by the OECD, particularly in relation to nanomaterials, who have a number of ongoing projects to develop test guidelines for assessing their dissolution and transformation in biological and environmental media (OECD Citation2018, Citation2020). In one recent example an inhalation-ingestion bioaccessibility assay was developed, subjecting samples to incubation in SLF, followed by synthetic gastric and intestinal solutions to simulate clearance from the lung into the GIT (Kastury et al. Citation2018b). The study concluded that compared to long-term extraction in SLF, or simulated gastric fluid alone, metal(loid) bioaccessibility increased significantly when inhalation and ingestion pathways were combined. In another approach, particulate matter samples were incubated in artificial mucus fluid prior to oral bioaccessibility extraction tests to investigate the release of potentially toxic metal ions (Alpofead et al. Citation2017). This simulated the deposition and transport of particulates from the mucociliary escalator in the respiratory tract to the GIT. The study showed that pre-incubation in mucus fluid actually encouraged the release of further metals when entering the simulated GIT, indicating that previous studies in gastric fluid alone may underestimate metal release from inhaled particulate matter.
Standardisation of simulated biological fluid
A 2017 ISO technical report (TR) for the assessment of nanomaterial biodurability identified a number of standardised test methods using SBFs for sweat, sebum, and digestive fluid replication, however, again no common or recognised methodology for inhalation investigations with SLFs. The TR only goes as far as providing suggested fluid compositions, various examples are provided for lung extracellular fluid, and one for lysosomal fluid (this example is termed, phagolysosomal simulant fluid (PSF) and is discussed later in comparison to other lysosomal simulant fluids). Given the range of fluids used as SLFs in the literature, the aim of this review was to highlight those fluids, or specific fluid constituents, that have been shown to suitably replicate the actual biological environment of the lung, either by composition or the observed effect. This review provides an overview of biofluids that are used in relation to exposure by inhalation through examination of the current peer-reviewed literature. Focus is given to fluids that are used to simulate specific extracellular and intracellular compartments found in the lung, namely lung lining fluid and lysosomal fluid. Both historic and progressive use of these fluids have been appraised and, where possible, certain components that play pivotal roles in dissolution mechanisms have been identified.
In the assessment of these fluids it is important to recognise the contribution that different design approaches may have on how effective the use of SBF may be, namely: (i) replication of biological conditions, (ii) operational validation through comparison to in vitro or in vivo findings and (iii) robustness (i.e. the ability to provide reproducible results from the test method). A key aim of this review was to highlight when any of these approaches have been examined or justified, allowing a comparison of these elements to determine critical considerations for the design and use of SLFs.
Biological and biochemical environments encountered by inhaled materials
Many parameters influence the deposition of inhaled materials along the respiratory tract, and these largely relate to the materials physicochemical characteristics as well as ventilatory conditions (Oberdörster Citation1993; Pilcer and Amighi Citation2010); these aspects, however, are out of the scope of this review. What is important to consider when assessing the use of SLFs that attempt to replicate dissolution of inhaled material within the lung, is the environment that said materials are exposed to, and how clearance and/or dissolution are attained, or at least attempted. Apart from the parameters defined by the material itself, such as particle size (including the diameter and length for a fibre), surface area, surface chemistry and chemical composition (Heim et al. Citation2020), there are key parameters of SLF itself which may affect material solubility that can be analogous with the physiological environments encountered, including temperature, pH, the presence of precipitants and ligands, protein content and ionic strength (Kanapilly Citation1977). Here we give a brief account of what is known in relation to these parameters within the literature, to aid in verifying which components within SLFs are most required and most accurate.
In the conducting zone of the respiratory tree is the mucociliary escalator, which is a mucus layer transported along by a ciliated cell system, ultimately working to propel inhaled material towards the larynx; this mechanism removes exogenous material from the lungs depositing in the GIT, or particularly soluble materials may be removed at this stage by diffusion (Oberdörster Citation1993). Although it is possible to replicate this mucus layer, the composition of this (either bronchial or nasal fluid) is markedly different to that found within the alveolar, and pragmatically, the most reasonable approach may be to assume that the fluids predicting GIT behaviour would ultimately be more relevant when considering material which deposits along the mucociliary escalator. Material that circumvents deposition in the conducting zone, and deposits within the gas exchange region will find itself within one of two environments and is subjected to other means of clearance, namely by resident macrophages, which patrol this region and engulf exogenous material, or even endocytosis by lung epithelial cells (not professional phagocytes) has been reported (Oberdörster Citation1993). In the gas exchange region the fluid is dissimilar to the mucus layer of the mucociliary escalator, and is termed lung surfactant, or lung lining fluid. It is these environments that are of most interest when establishing a suitable environment to model dissolution within the respiratory system: lung surfactant, and intracellular compartments. Interstitial fluid, referring to the fluid that surrounds tissues/cells, e.g. surrounding the alveoli (), may also hold some relevance; at times this term is used when describing a formulation which others still refer to as Gamble’s (Heim et al. Citation2020), as well as if inhaled material gains access to the pulmonary interstitium. However, if the material is found to reach the interstitium it would either be internalised by interstitial macrophages, or transported to the lymphatic system (Oberdörster Citation1993); in any eventuality, the appropriate simulant fluid to model this environment would reflect that of the intracellular phagocyte, or be designed to mimic an environment unlike that of the lung, e.g. blood serum ().
An understanding of these environments, described below, should allow an indication of how suitable, or unsuitable, some SLFs may be.
Extracellular compartment
The main function of lung lining fluid, consisting of an alveolar sub-phase fluid and pulmonary surfactant, which is secreted by type II alveolar epithelial cells, is to prevent collapse of the alveoli space while breathing, and is done through the relief of surface tension (Creuwels et al. Citation1997; Ng et al. Citation2004). Incidentally, this surface tension is also responsible for fluid movement within the alveolar space, explained by the Marangoni effect (Sosnowski Citation2018). There is no requirement of great volumes, therefore within the alveoli, lung surfactant is as little as 0.07–0.5 µm thick (Gamble Citation1976; Sleigh et al. Citation1988; Hatch Citation1992; Kelly and Mudway Citation2003; Ng et al. Citation2004), compared to up to 20 µm in the upper respiratory tract (Yoneda Citation1976; Widdicombe and Widdicombe Citation1995). The composition of lung lining fluid has been relatively well described. Ionic strength has been reported with various values, with Na+ reported as being between 82 and 132 mM, Cl− between 84 and 140mM, K+ as 6–29 mM, Ca2+ as 4 mM, P as 17 mM, and S as 21 mM (Joris et al. Citation1993; Knowles et al. Citation1997; Jayaraman et al. Citation2001b). Although some are found to be unbound and present as ions (e.g. Cl, Na, and K), others are thought to be bound, and held within macromolecules, such as P in phospholipids and S within sulphated mucins (Joris et al. Citation1993). Protein and lipid components have been established, and organic ligands with high complexation potential to metal ions have been found and characterised in the surrounding area, including citric, lactic, malic, oxalic and salicylic acids (Rozalen et al. Citation2013). Citrate and lactate, within human blood plasma, have been measured at 0.12 mM and 1.84 mM, respectively (Plumlee and Ziegler Citation2003; Rozalen et al. Citation2013); with composition of interstitial fluids considered to be similar to blood plasma, only with lower concentration of proteins (Rozalen et al. Citation2013). There have been over 1,500 specific proteins identified in human bronchoalveolar lavage (BAL) fluid (Wu et al. Citation2005). These can be derived locally, through secretion from lung-resident cells, and are therefore unique to this environment, such as surfactant proteins secreted from type II epithelial cells (Serrano and Pérez-Gil Citation2006), or they may enter the lungs from other compartments, such as serum albumin (Stockley Citation1984; Hermans et al. Citation1998; Kim et al. Citation2002), as a circumstance of vascular permeability (Webber and Widdicombe Citation1989; Hatch Citation1992).
Protein and antioxidant content
The fluid of the gas exchange region of the lung (the alveoli) contains a higher concentration of proteins compared to the upper respiratory tract (Hatch Citation1992). Of all the proteins present in lung lining fluid, albumin is the most abundant, contributing 2.9–5.35 mg/ml (up to 50%) of the total protein (5.7–11.7 mg/ml). Other highly abundant proteins in lung lining fluid (measured in BAL fluid) include immunoglobulins IgA (0.026–1.42 mg/ml), IgG (0.64–0.89 mg/ml) and IgM (0.028 mg/ml), transferrin (0.03–0.16 mg/ml), and the digestive enzyme lysozyme (0.03–0.07 mg/ml) (Holter et al. Citation1986; Webber and Widdicombe Citation1989; Rennard et al. Citation1990; Thompson et al. Citation1990; Hatch Citation1992; Sutinen et al. Citation1995; Dargaville et al. Citation1999), as well as various proteases, including cathepsin D (0.08 mg/ml), dipeptidyl peptidase 4 (0.001 mg/ml), dipeptidyl carboxypeptidase I/angiotensin-converting enzyme (0.002 mg/ml), and elastase (0.067 mg/ml), released from neutrophils during times of inflammation (Woods et al. Citation2020). In addition, the presence of antioxidants in lung lining fluid is known (Cross et al. Citation1994; Charrier et al. Citation2014), with their role recognised as detoxification (Krawic et al. Citation2017). The concentration of various antioxidants has previously been identified in BAL fluid, and although this represents the concentrations in the lung as a whole, they do provide useful benchmark values for lung lining fluid. For example, ascorbate was shown to be within a range of 40–200 µM (0.007–0.035 mg/ml), urate 90–207 µM (0.015–0.035 mg/ml), and glutathione 109–129 µM (0.034–0.04 mg/ml) (Cantin et al. Citation1987; Hatch Citation1992; Slade et al. Citation1993; van der Vliet et al. Citation1999). Further detoxification within lung lining fluid is achieved by highly abundant metal binding proteins, specifically glycoproteins such as albumin and mucin (Cooper et al. Citation1985; Duranti et al. Citation2001; Bal et al. Citation2013). As well as specific functionality, the existence of proteins with inhaled particles has a number of implications due to formation of a protein corona on the particle surface, leading to key surface modifications, causing significant differences in particle appearance and behaviour, and ultimately in vivo response (Nel et al. Citation2006; Monopoli et al. Citation2011). For example, Raesch et al. (Citation2015) characterised the protein corona formed in pulmonary surfactant upon NP inhalation; different NPs demonstrated remarkably different protein corona, consisting of up to 417 different proteins. And although different materials have been shown to associate with different proteins, some commonality has been observed, with key immunomodulatory proteins commonly associating with various materials, including surfactant protein A, napsin A and complement proteins (Kumar et al. Citation2016). In the study by Raesch et al. (Citation2015), despite the differences in the surfactant-NP protein corona, there was a distinct preference for proteins with high lipid and surface binding such as surfactant proteins SP-A and SP-D, and glycoprotein DMBT1. Of the surfactant secreted by alveolar epithelial cells, approximately 10% is comprised of surfactant proteins (SP-A, SP-B, SP-C and SP-D), with the remaining 90% of surfactant being composed of lipids, mostly phospholipids including phospathidycholine (PC) in the form dipamitoylphosphatidylcholine (DPPC) (41–70%), phosphotidylglycerol (8–10%), phosphatidylethonanolamine (5%), phosphatidylinositol (3%), and phosphatidylserine, but also neutral lipids such as cholesterol, triglycerides and free fatty acids (Kahn et al. Citation1995; Creuwels et al. Citation1997; Goerke Citation1998; Lang et al. Citation2005). It is these lipid components that offer the aforementioned protection against alveolar collapse, as well as providing further support in defence of the lungs, through opsonisation of exogenous material, as well as activation and recruitment of immune cells (Van Iwaarden et al. Citation1992; Citation1994; Kalina et al. Citation1995; Creuwels et al. Citation1997).
pH of extracellular fluid
As well as fluid composition, the pH of lung lining fluid is a parameter needing clarification. Although, the pH here is often considered near-neutral (pH 7.4) (Adamcakova-Dodd et al. Citation2014), there is not a set, or stable pH, as this environment is subject to change dependent of circumstance – during active host defence in the form of inflammation, for example (Ng et al. Citation2004), which would be the case if exogenous material was to reach the alveoli. In the upper respiratory tract the pH of the fluid lining has been measured in humans to be pH 6.6 via a bronchoscopy-associated pH probe, and pH 6.8 in excised tissue, and in exposed mouse trachea to be pH 6.9–7.1 (Bodem et al. Citation1983; Jayaraman et al. Citation2001a, Citation2001b). Although changes were shown to align with similar changes in blood pH (Jayaraman et al. Citation2001a), the mechanism of pH alteration has been assigned to release of H+ and/or HCO3− from bronchial epithelial cells (Lee et al. Citation1998; Coakley et al. Citation2003), in the already CO2-rich environment. Buffering of this fluid is thought to be obtained by secreted proteins such as mucin glycoproteins (Ng et al. Citation2004). In the alveolar space, the presence of alveolar macrophages is expected to influence the composition of the lung lining fluid and, being a source of high levels of H+, will impact greatly of alveolar fluid pH (Ng et al. Citation2004). Accurate measurements of alveolar pH are not easily obtained, but it has been suggested that this fluid is approximately pH 6.9 (Nielson et al. Citation1981). pH buffering within this environment is thought to be through a number of mechanisms including a CO2-bicarbonate buffer system (Kanapilly Citation1977) or the activity of surfactant proteins (Ng et al. Citation2004). Alveolar macrophages will, under resting conditions, release H+ at a rate of 2–3 nmol/min/106 cells, this is increased by up to 5 times when cells are activated (Bidani et al. Citation1989; Heming and Bidani Citation1995). So in the presence of inhaled materials, for example, when there is an increase in the cells’ metabolic activity and recruitment of further immune cells, it is possible that the increased release of H+ would reduce the localised pH (Ng et al. Citation2004). In addition to this, the environment surrounding activated macrophages would also be enriched with nitric oxide (NO) and other reactive nitrogen and oxygen species, as well as the secretions of recruited neutrophils, including further reactive oxygen species (ROS) as well as granule components (Tam et al. Citation2011).
Intracellular compartments
Although when reaching the alveoli the fluid first encountered is that described above, the main defence mechanism used to remove exogenous material is no longer transport along the mucociliary escalator, instead fibres and particles will be exposed to the action of the two cell phenotypes, firstly interstitial macrophages (Beeston et al. Citation2010), and later neutrophils. These are professional phagocytes, accordingly these cells will actively locate and engulf exogenous material, often by receptor-mediated phagocytosis. The purpose of this is removal, which is achieved by intracellular degradation within membrane bound vesicles, or by motility, when the cells laden with engulfed material physically remove themselves by reaching the mucociliary escalator or lymphatic system (Collier et al. Citation1992). During internalisation, the cell forms a phagosome to engulf the material. This vesicle then combines with others (namely lysosomes) to form a phagolysosome (Kreyling Citation1992; Marques et al. Citation2011). This intracellular compartment contains both chelators and precipitators known to influence solubility (Kreyling Citation1992), and an environment in which a number of conditions are engaged to digest the phagocytosed material. This includes a drop in pH to acidic conditions, stimulation of proteolytic lysosomal enzymes, and production of ROS to aid digestion or act as signalling molecules (generating the oxidative/respiratory burst) (Nyberg et al. Citation1989b; Collier et al. Citation1992; Kreyling Citation1992; Oberdörster Citation1993; Breitner et al. Citation2018).
Phagolysosome composition
As would be expected from the transition between these distinct intracellular compartments, the phagolysosome is not static, neither in location (in fact they are very mobile (Bandyopadhyay et al. Citation2014)) nor composition. In general terms, the composition of lysosomes includes hydrolytic enzymes and structural elements of the lysosome membrane: lipids and proteins (Tappel Citation1969). However, to be more precise, this environment is compositionally complex and, furthermore, will undergo a multitude of changes with internalisation of exogenous material. There is a vast range of enzymes present within lysosomes, from all major enzyme groups, including but not limited to proteases, peptidases, nucleases, phosphatases, and glycosidases. This gives an equally vast range in substrate specificity (Tappel Citation1969). This environment is also rich in complexation agents, including citrate and bicarbonate; constitutively produced by all mammalian cells by the mitochondria. Citrate is found both within the cytoplasm (Nyberg et al. Citation1989b; Collier et al. Citation1992) and intracellular membrane bound vesicles including late endosomes, lysosomes (Gomez et al. Citation2010), and phagolysosomes, where it can be present at concentrations up to 0.2 mM, and bicarbonate up to 30 mM (Nyberg et al. Citation1989b; Collier et al. Citation1992). Although metal carbonate and bicarbonate complexes have been observed, they are unstable in acidic aqueous medium and readily decompose to release CO2. Specific ligands [e.g. tris(2-pyridylmethyl)amines] are required to stabilise these complexes (Cheyne et al. Citation2006). With the transition of phagosome to phagolysosome – during which intracellular vesicles merge with the phagosome and degranulation occurs, spilling the vesicle contents into the phagolysosome – the high level of enzymes present within lysosomes, previously described, contribute to a rise in protein concentration, at times contributing to 30–40% of phagolysosome content. Along with water, there is an influx of ROS and various ions, changing the ionic strength. Proton pumps are active at the vesicle membrane, and the compartment acidifies (Hampton et al. Citation1998).
Range of phagolysosome pH
Measurements of lysosomal pH have been previously reported, including rat alveolar macrophages demonstrating lysosomal pH values of between 5.2 and 5.8 (Johnson Citation1994; Johansson et al. Citation1997), with similar values of pH 5.2 demonstrated in interstitial macrophages of the same species (Johansson et al. Citation1997). In rabbits, after inhalation and subsequent internalisation of silica particles by alveolar macrophages, the pH of the phagolysosome was measured to be 4.9 the day after particle exposure, with further decreases to 4.5 one week after exposure (Nyberg et al. Citation1989b). In vitro, also with internalisation of silica particles by rabbit alveolar macrophages, pH was found to range between 5.1 and 5.5 (Nyberg et al. Citation1989a, Citation1989b), and HeLa cells have been shown to contain lysosomes which reach pH values of under pH 4 (Benjaminsen et al. Citation2013). This range in pH reflects the dynamic endocytosis system, where the pH is expected to range from 6.0 to 6.5 in early endosomes, 5.0–6.0 in late endosomes, and 4.5–5.0 in lysosomes (Schmaljohann Citation2006). In fact, when considering the known optimum pH for substrate-specific interactions of lysosomal enzymes, this scale of lysosome pH may even be greater, for example optimum hydrolysis of some structural amino acids components, or of peptides a can be up to pH 7.2 and 7.8, respectively, while optimal hydrolysis of albumin can be at as low as pH 2.5 (Tappel Citation1969). Low pH has been known to contribute to the dissolution of many materials (Lundborg et al. Citation1984; Rozalen et al. Citation2013) but this is not considered the physiological reason for lowering pH. Numerous enzymes present within lysosomal fluid are sensitive to pH, and it is acidification of the local environment that causes activation of these enzymes, leading to digestion of engulfed material (Hampton et al. Citation1998; Kagan et al. Citation2010). In some cases this action is through ROS generation, where free radicals such as hydroxyl radicals and hypochlorous acid are formed through the activity of enzymes such as nicotinamide adenine dinucleotide phosphate-oxidase (NADPH oxidase), myeloperoxidase, or numerous proteases (Hampton et al. Citation1998).
Simulation of the lung environment
In the decades since J. L Gamble (Citation1976) first described a simulation of extracellular fluid of skeletal muscle, the use of simulated extracellular fluids, and more recently intracellular fluids, has been developed and refined, with focus often placed on the respiratory system. In the early applications of these SBFs it was thought that the ion concentration was similar to that of human blood plasma (Kokubo et al. Citation1987), and therefore physiologically accurate; however, as many of the ions present would complex with proteins it was later deemed that ion concentrations were too high. More recent formulations have used accurate concentrations of dissociated ions, resulting in less Ca and Mg (Oyane et al. Citation2003). There have also been examples of solutions used for in vitro bioaccessibility studies that are relatively simple solutions, for example, water or saline (0.9% NaCl) solutions were both used to investigate metal release from exhaust particles (Artelt et al. Citation1998; Birmili et al. Citation2006). However, the specificity of SBF use has generally been increasing and different biological compartments and environments are being replicated. For studies mimicking the lungs, this has evolved into fluids to specifically replicate two environments described above, both the extracellular regions of the lung – including interstitial and lung lining fluid – and the intracellular regions of phagocytic cells, as indicated in . As has already been discussed there is presently no standardised or accepted fluid composition for the replication of lung compartments and therefore a wide range of compositions have been used.
Comparing fluids which simulate lung lining fluid
Gamble’s solution
Of all the SLFs modelling the extracellular environment of the lung, Gamble’s solution is the most prevalent. It has been widely used for the risk assessment of human exposure to inhalable pollutants, including assessment of fibre dissolution (Alexander et al. Citation1994), durability of carbon nanotubes (Osmond-McLeod et al. Citation2011), NP drug delivery (Menon et al. Citation2014), degradation of airway stents (Jang et al. Citation2014), bioaccessibility of Cr in soils (Broadway et al. Citation2010), and trace metals in particulate matter (Huang et al. Citation2018; Luo et al. Citation2019; Xie et al. Citation2019b), for example Hg from mine waste (Gray et al. Citation2010), metal emissions in fuels (Coufalik et al. Citation2019), Pt in road dust (Wiseman et al. Citation2018) or As in Chinese haze pollution (Xie et al. Citation2019a). Since its introduction in 1967, several researchers have modified the initial formulation (Gamble Citation1976; Boisa et al. Citation2014). However, the use of the generic term SLF to describe different formulations can lead to confusion as to which composition has actually been used. The name “Gamble’s solution” is often retained with variation in the nomenclature such as “modified Gamble’s solution” (Christensen et al. Citation1994), or ‘Gamble’s serum simulant” (Ansoborlo et al. Citation1990) being used. The composition of Gamble’s solution, and other fluids used to simulate the neutral pH extracellular environment of the lung, is given in .
Table 1. Composition (g/L) of frequently used biofluids used to simulate the neutral conditions of the lung lining fluid.
Gamble’s solution has been used in conjunction with other fluids, and can be found in the assessment of many materials, including man-made mineral fibres (MMMF) (Zoitos et al. Citation1997; Guldberg et al. Citation1998; Sebastian et al. Citation2002), Be ore materials (bertrandite, beryl, BeSO4, BeO) (Deubner et al. Citation2011), Ag NPs (Breitner et al. Citation2018), rare earth elements (e.g. Ce, La, Nd and Gd) in refinery emissions (Gao et al. Citation2020) and polycyclic aromatic hydrocarbons (PAHs) in coal-related particulate matter (Gao et al. Citation2019). One example highlighted the influence of oxygen on both UO3 and UF4 breakdown in Gamble’s solution (Ansoborlo et al. Citation1990, Citation1992). The addition of superoxide dismutase (SOD) to the composition, or simply bubbling with oxygen, led to increased U mineral breakdown and a reduced half-time of 100 to 26 days for UF4 and 70 to 16 days for industrial UO3. Another study found UF4 dissolution in Gamble’s solution to better reflect in vivo results from intratracheal instillation than cell culture medium (CCM) (Chazel et al. Citation2000, Citation2003). These results reinforce the well-accepted conclusion that fluid composition has an effect on the material dissolution behaviour, in these cases with the addition of O2 or SOD.
However, other parameters can also strongly influence solubility, and not all materials are as sensitive to compositional changes. In 1997, Zoitos et al. conducted a round robin of 4 laboratories measuring the solubility of several man-made vitreous fibres (MMVF) in SLFs. Although the SLFs were based on Gamble’s solution, there were differences in fluid composition from laboratory to laboratory; these were accurately summarised in the publication, alongside fibre compositions. Overall, the data showed considerable variation in dissolution rates with individual values differing from one another by up to a factor of four. While this may be partly influenced by differences in the fluid compositions, other factors relating to the specific methodology used – in this case a flow-through system – such as flow rate may also have significantly influenced dissolution values. While this was the case, the values were in well-defined ranges for each fibre type, allowing clear distinction between glass wool, stone wool and slag wool. A second interlaboratory comparison conducted in 2003 provided similar conclusions (Guldberg et al. Citation2003).
Conversely, a study of the biodurability of talc found the composition of the fluid to have no effect on the dissolution rate, reporting similar dissolution rates in Gamble’s solution and a phosphate buffered saline solution, both at pH 7.4 (Jurinski and Rimstidt Citation2001). Likewise, when Garger et al. (Citation2004, Citation2013) compared the dissolution of airborne radioactive fuel particles in original Gamble’s solution with a modified version (containing an increased salt content and reduced citrate content) they found 137Cs and 90Sr to have no dependence on the type of simulant. However, 239 + 240Pu dissolved significantly faster in Gamble’s than the modified version (pH 8.2), indicating the effects to be material specific. In an analogous study, Aladova et al. (Citation2007) presented the dissolution of Pu in aerosol particles (Pu oxide and Pu nitrate) in other SLFs of pH 7.4. One of these was a modification of Gamble’s known as serum ultrafiltrate (SUF) and the other being Ringer’s solution, which contains a far simpler salt formulation and practically no complexation agents. When comparing the two radionuclide studies, Pu in fuel particles dissolved slowly into Gamble’s solution compared to Pu oxide into Ringer’s solution, and even more into SUF (Garger et al. Citation2013).
Hatch’s solution
An alternative to Gamble’s solution is a composition known as Hatch’s solution, however its use within the literature is less evident. Where Gamble’s solution contains citrate as a substitute for organic components, Hatch’s solution incorporates proteins and enzymes in the mixture (Sysalova et al. Citation2014). It has primarily been used in bioaccessibility studies of airborne particulates including welding fumes (Berlinger et al. Citation2008; Ellingsen et al. Citation2013, Citation2017; Berlinger, Weinbruch et al. Citation2019; Zadrapova et al. Citation2019), urban particulate matter (Sysalova et al. Citation2014) and airborne particulates from car batteries (Dartey et al. Citation2014). When investigating the bioaccessibility of welding fumes, Hatch’s solution was used alongside Gamble’s solution, to evaluate the use of proteins and enzymes in the composition (Berlinger et al. Citation2008). The solubility of metals in the welding fumes and associated particulate matter (Fe, Mn, Cr, Ni, Mo, Ti) was found to vary greatly depending on the fluid composition and the process being conducted, this was observed also for other hot work processes in Hatch’s solution (Berlinger, Skogen et al. Citation2019). While higher metal concentrations were obtained with Hatch’s solution after the 24 h period, the lack of in vivo data means no comment can be made on the most accurate fluid for replication of in vivo behaviour. While this is the case, the authors indicated a preference for Hatch’s solution due it being more compositionally similar to human lung lining fluid.
In a similar study, a variety of SLF compositions were compared including original Gamble’s solution, Hatch’s solution and simulated epithelial lung fluid (SELF) (Kastury et al. Citation2018a). Bioaccessibility of As and Pb was significantly higher in Hatch’s solution, in some cases up to 47 times, and this was attributed to the more complex composition, with components such as albumin being well known for binding metals. However as with the previous study, the lack of in vivo data means no conclusion can be made about the most appropriate fluid. The same was reported for a number of Mn compounds in Hatch’s solution, being 20–40 times more soluble than the Gamble’s equivalent (Vitarella et al. Citation2000). A similar study of welding fumes was carried out in Hatch’s solution to determine the solubility of Cr, Mo, V and W (Ellingsen et al. Citation2017). It was concluded that the bioaccessibility of Mo in shipyard welding fumes to be substantially higher than Cr, V or W (Ellingsen et al. Citation2017). In a study examining extraction of metals from soils impacted by open cast mining, when comparing Gamble’s and Hatch’s solutions a variation in extraction and bioaccessibility was observed across the different metals (Zadrapova et al. Citation2019).
Simulated epithelium lung fluid (SELF)
In addition to Gamble’s and Hatch’s solutions, several other lung fluid formulations have been designed to approximate the composition of the extracellular fluid. A SELF, as mentioned above, was formulated by Boisa et al. (Citation2014) to have more physiological relevance. This was done by including specific components such as lung surfactants, high molecular mass proteins, and antioxidants in addition to the inorganic components that are found within the more traditional formulations (Boisa et al. Citation2014). This was used to determine human risk to Pb in soils (Boisa et al. Citation2014), Pb in urban street dust (Dean et al. Citation2017), Ni in soils (Zhong and Jiang Citation2017) and inhalation exposure to organic contaminants such as PAHs (Li et al. Citation2019).
Greenwell et al. (Citation2003) employed a similar SELF fluid, however this time excluding the inorganic salts and solely containing ascorbate, urate and reduced glutathione in water. When compared to rat epithelial lining fluid obtained by pulmonary lavage they actually found it to be more effective at minimising oxidative effects. This was attributed to the rat epithelial fluid containing different quantities of the three major low-molecular weight antioxidants, alongside a myriad of other antioxidant and pro-oxidant molecules such as superoxide dismutase, cellular debris and neutrophil respiratory burst end products (Greenwell et al. Citation2003).
Simulated interstitial fluid (SILF)
SILF is another modified extracellular solution that has been reported in the literature. This is compositionally similar to Gamble’s solution, also utilising citrate in place of proteins (Gupta and Ahsan Citation2011). A number of studies have reported the use of SILF to investigate the release properties of inhaled drug formulations due to its close replication of the mucosal fluids present in the respiratory system (Gupta and Ahsan Citation2011; Costabile et al. Citation2015; Pellosi et al. Citation2018). Others have employed SILF to investigate the solubility of airborne U compounds (Heffernan et al. Citation2001) and asbestos (Vigliaturo et al. Citation2018). Two analogous studies investigated the effect of adding organic components to the SILF composition, namely bovine lung extract and DPPC. Spitler et al. (Citation2015) added natural bovine lung extract, a pulmonary surfactant containing phospholipids, proteins and lipids naturally present in vivo. Interestingly, they found there to be no apparent difference in dissolution rates of U from soil, however acknowledged that further studies with different quantities of surfactant and on a sample of the separated respirable fraction would be necessary to confirm this conclusion (Spitler et al. Citation2015). Likewise, Sr was the only element from a variety of air samples that showed increased bioaccessibility with the inclusion of DPPC (Pelfrene et al. Citation2017).
“Bio-relevant” SLF
To further enhance the “bio-relevance” of SLF, a recent study set out to design a fluid primarily for use in inhalation biopharmaceutical assays (Kumar et al. Citation2017; Hassoun et al. Citation2018). The composition included the major soluble lipids and proteins including DPPC, dipalmitoylphosphatidylglycerol, cholesterol, albumin, IgG, transferrin and antioxidants (ascorbate, urate and glutathione), as had been identified in the characterisation of healthy human respiratory tract lining fluid (Bicer Citation2015). A pragmatic approach was taken to maintain biological relevance with fluid stability and reproducibility. Species such as unsaturated lipids were avoided due to their susceptibility to oxidation. Characterisation indicated that the fluid was stable for 2 weeks if refrigerated. However, at 37 °C changes became significant after 24 h, meaning any in vitro experimentation would have to be limited to this time period. This formulation was found to be a useful comparison to in vivo effects, as poorly water-soluble drugs which would not be soluble in traditional Gamble’s solution had their solubility enhanced in the lipid-rich fluid more akin to actual lung lining fluid (Kumar et al. Citation2017).
Artificial interstitial fluid (AIF)
AIF, presented by Stopford et al. (Citation2003), has been used to predict the behaviour of inhaled exogenous material, for example CuO and TiO2 (Cathe et al. Citation2017). However, this is not exclusive to the respiratory system and primarily mimics the fluids surrounding cells within skin tissue. Cathe et al. (Citation2017) incubated CuO and TiO2 NPs (sized 24 and 53 nm respectively) in AIF prior to exposure to human keratinocyte cells. The incubation led to increased agglomeration and therefore greater deposition on the cell monolayer than when incubated in cell culture medium. Interestingly, this increased deposition lead to greater toxicity of CuO but not TiO2. While this is a useful insight into the behaviour of NPs in physiological fluids, the increased toxicity observed here is due to increase deposition within an in vitro exposure plate, which is an artefact of the method and therefore potentially an artificial effect that is not known to occur in vivo.
Serum ultrafiltrate (SUF)
The previously described SUF, originally based on Gamble’s solution, has also been reported as a mimic of ultrafiltrate blood serum and lung fluid. It has been used in solubility tests of airborne U from processing and recycling plants (Metzger et al. Citation1997; Metzger and Cole Citation2004) and Th in U mill tailings (Eidson and Mewhinney Citation1980; Eidson and Griffith Citation1984; Reif Citation1994; Cheng et al. Citation1997; Stefaniak et al. Citation2009). One study investigated the solubility of yellowcake (uranium ore) in SUF and a SLF to determine the importance of composition, pH, and temperature. The major compositional differences between the fluids were that the SLF contained Mg and a higher concentration of Ca, while SUF contained ammonium ions. Both solutions were kept at a pH of 7.3. For the protein substitutes, acetate and citrate were used in SLF, while SUF contained amino acids and diethylenetriaminepentaacetic acid (DTPA). The results indicated precipitation to be a major factor in both fluids indicating solution composition changes to not be readily reversible, however the authors indicated SUF was more easily maintained as a clear solution (Eidson and Griffith Citation1984).
Subsequent studies by Cheng and colleagues have employed SUF to determine the dissolution of Pu and metal tritides (hydrides of 3H), often in line with the ICRP 66 lung dosimetric model which details how to calculate radiation doses in the respiratory tract (ICRP Citation1994). The findings included the influence of particle size on dissolution kinetics (Cheng et al. Citation1997, Citation2002, Citation2004). The dissolution reported by Cheng et al. (Citation2002) were significantly faster than that reported for hafnium tritide by Inkret et al. (Citation2001). Inkret et al. (Citation2001) extrapolated a biological half-time from these in vitro results and compared a half-life of 30 days for titanium tritide to 105 days for hafnium tritide. A separate study indicated 114 days for zirconium tritide (Zhou et al. Citation2010), indicating the biokinetics of inhaled tritides to be material specific. It should be noted that Inkret and colleagues did not indicate the composition of their SLF, and only that it was of neutral pH. In one particular study, the in vitro solubility of Pu oxide in SUF was found to be consistent with values reported in ICRP (ICRP Citation1994).
Comparing fluids which simulate the lysosome
Due to their function, lysosome simulations obviously differ from those of lung lining fluids. As described previously, a lysosome will contain proteolytic enzymes and ROS used to digest the engulfed substances, with an acidic pH generally described as being pH 4.5 (see earlier discussions on pH variability within the intracellular compartments). Although the extent of phagocytosis is not completely verified (Dean et al. Citation2017), and will be dependent upon dose and material physicochemical parameters, it has been hypothesised that within 24 h of deposition more than 90% of inhaled materials will be phagocytised by alveolar or airway macrophages (Aladova et al. Citation2007). It is therefore important to also assess materials in this acidic intracellular environment to get an accurate picture of their bioaccessibility and durability within the lung. Of all the simulated lung fluids that are used, it is those which model the environment of the (phago)lysosome that arguably have the greatest influence over material solubility, but which also pose most questions regarding the suitability of its composition. Literature on fibres, in particular, emphasises the influence of pH. It has often been reported that different types of fibres have very different in vitro dissolution rates dependent on the pH. Glass wool fibres favour dissolution at neutral pH, while stone wool dissolved faster at pH 4.5 (Thelohan and de Meringo Citation1994; Guldberg et al. Citation1998); as shown in the comparison of MMVF11.2 glass wool and basalt-based stone wool MMVF21 (Christensen et al. Citation1994). The study concluded that a lower Al2O3 content favours greater dissolution at neutral pH, and with associated high SiO2 and relatively low CaO content, the same fibre was less soluble under acidic conditions. In fact, varying composition of stone wool fibres has been a strategy to develop less biopersistent materials, and was achieved by either lowering alumina content to less than 3–4% or by increasing alumina content so it is greater than 17–18% with a concomitant decrease in SiO2 to below 42–43% (Guldberg et al. Citation2000). When Luoto et al. (Citation1994) exposed MMVF to alveolar macrophage (AM) culture they found the dissolution profiles to be different to those obtained in Gamble’s solution (pH 7) or CCM (RPMI 1640 medium supplemented with 10% FCS). For example, Fe dissolution was minimal in the neutral Gamble’s solution and high in acidic AM culture (Luoto et al. Citation1994). Cannizzaro et al. (Citation2019) assessed RCF and two alkaline earth silicate (AES) wools incubated in Gamble’s solution at pH 7.4 and a lowered pH of 4.5. RCF were found to be unaffected during the 14-day exposure of either fluid. For AES, the acid solution was found to cause dissolution of thin fibres only, in neutral solutions dissolution patterns were specific to fibre composition. AES containing CaO dissolved rapidly and completely within 14 days through leaching of Si and Ca [as measured by inductively coupled plasma atomic emission spectrometry (ICP-AES)], while fibres not containing CaO were shown to release moderate levels of Mg and Si (Cannizzaro et al. Citation2019). In a study which examined a similar panel of high-temperature insulation wools (HTIW), Boyles et al. (Citation2018) also demonstrated a high dissolution of AES when compared to RCF. Cannizzaro et al. (Citation2019) tested calcium magnesium silicate wool (CMS) and magnesium silicate wool (MS), whereas Boyles et al. (Citation2018) tested calcium silicate wool (CaS) in addition to CMS and MS. Boyles et al. found dissolution to be greater in pH 4.5 solutions compared to pH 7.4 solutions, and fibres containing Mg and Ca were shown to release these components at almost equal levels. The dissolution tests conducted by Boyles et al. (Citation2018) used a fluid composition which differed from that of Cannizzaro et al. (Citation2019). Still based on Gamble’s solution, the Mg- and Ca-contributing salts had been excluded, to improve detection of low level releases from the fibres (Boyles et al. Citation2018), this may have contributed to the differences observed between these two studies.
Artificial lysosomal fluid (ALF)
Although not likened to any specific biological environment, acidic solutions had already been used in a fibre durability study in 1984, and consisted of simple citric acid or phthalate buffers (Morgensen Citation1984). It was acknowledged only later, by Thelohan and de Meringo (Citation1994), that the use of acidic solutions were to replicate the lysosomal compartment. The composition of the acidic solution used, also in the assessment of MMVF solubility, was more complex than those of previous studies; unfortunately no explanation to the origin of this formulation was given. The term artificial lysosomal fluid was assigned to this formulation in later studies on metals release from stainless steel (Herting et al. Citation2006); ALF has become a commonly used fluid for simulating the lysosome.
ALF has since been slightly modified compositionally to test a variety of materials such as stainless steels (Herting et al. Citation2007), alloys (Hedberg et al. Citation2010), and occupational dust (Witt et al. Citation2014; Brown et al. Citation2019; Figueroa-Lara et al. Citation2019). A study on stainless steel alloys determined preferential leaching of Fe followed by Ni and Cr, primarily in the first hour of immersion in ALF (Herting et al. Citation2008). Hedberg et al. (Citation2010) demonstrated that the organic species in ALF strongly complexed leached Cr (III) from alloy. Smaller particles of Cr and Fe were shown to dissolve faster than large particles for alloys, but the opposite was true for pure metals. Authors attributed this to particle agglomeration, and the shift in thermodynamic equilibrium resulting from the release of metal ionic species into the medium (Hedberg et al. Citation2010). Interestingly, this effect was only observed in ALF but not in Gamble’s solution or other biological simulants. A separate study confirmed the influence of particle diameter on Fe release from stainless steel, as well as the preference of ALF to leach Fe, over Cr and in this case also Ni (Midander et al. Citation2007). Stainless steel 316 L powders leached more Fe than Cr or Ni, disproportionally to the particle composition concentrations. When considering the particles tested within this study alone (data for a stainless steel sheet was obtained from elsewhere), a strong particle size-dependent leaching of Fe was observed. This was also true for Cr and Ni, but to a lesser extent (Midander et al. Citation2007). The release of all elements was far lower when tested in Gamble’s solution, with no clear effect of size. (Midander et al. Citation2007).
A number of studies have indicated ALF to correlate with in vivo behaviour. Ag ion release in ALF was seen to be consistent with the toxicity results observed in primary alveolar macrophages from mice (Hamilton et al. Citation2018). Likewise, the stability of a variety of NPs in ALF was shown to correlate well the behaviour in J774A.1 cells (MilosevicAc et al. Citation2019). Studies in ALF allowed the distinction between NP dissolution (as observed for iron oxide NPs) and aggregation (as observed for silica NPs), confirming the behaviour observed in the lysosome environment of the J774A.1 cells. The composition of ALF and other commonly used lysosome-simulant fluids is shown in .
Table 2. Composition (g/L) of frequently used biofluids for simulation of the acidic conditions of the lysosome.
Studies comparing ALF with other SLFs
Frequently, ALF is used in parallel studies with other SLFs to allow comparisons to be made. It has been used to assess a wide range of materials including the solubility of airborne particulate matter (Wiseman and Zereini Citation2014; Polezer et al. Citation2019), fly ash (Bourliva et al. Citation2020), bioaccessibility of PAHs (Gao et al. Citation2019) and rare earth elements (Gao et al. Citation2020), mobility of Pt group elements (Zereini et al. Citation2012), the solubility of chrysotile asbestos contained within fragments of break-debris (Boyles et al. Citation2019), zeolite surface behaviour (Giordani et al. Citation2019), NP aggregation (Fashina et al. Citation2013; Zhong et al. Citation2017), drug delivery systems (Mulla et al. Citation2017), and biotransformations of graphene oxide (Liu et al. Citation2018; Qi et al. Citation2018).
The effect of low pH has been evident; a greater solubility in ALF compared to SLFs of a higher pH has been shown for a number of metals including In (Andersen et al. Citation2017), Pu (Griffiths et al. Citation2016), Am (Griffiths et al. Citation2016), Fe (Hernandez-Pellon et al. Citation2018), Be (Huang et al. Citation2011), Mn in smoke haze (Huang et al. Citation2016), NiO NPs (Shinohara et al. Citation2017), ZnO NPs (Muller et al. Citation2010; Adamcakova-Dodd et al. Citation2014), and nano- and micro-particulate CuO (Semisch et al. Citation2014). However, it has been shown that it is not just the low pH that has the effect on solubility, but the composition of the ALF (Stebounova et al. Citation2018; Tan et al. Citation2020). When a simplified acidic solution of pH 4.5 was used to test the dissolution of Mn NPs, no solubility was observed, in comparison to steady dissolution in ALF. A similar result was seen for stone wool dissolution, where a citric acid buffer at pH 4 caused aggressive dissolution, this was not replicated with a potassium hydrogen phthalate buffer at the same pH (Morgensen Citation1984). However, the authors tested further conditions and found: i) no linear relationship between citric acid and fibre dissolution; ii) a solution at pH 6 was found to induce almost no fibre dissolution, when a pH 4 solution of equal citric acid concentration caused significant dissolution; and iii) a pH 5 solution was shown to cause less fibre dissolution than the pH 4 solution, even though the pH 5 solution contained a higher concentration of citrate (Morgensen Citation1984); these points indicate that the effect of ALF is likely a combination of both the acidic pH and presence of citric acid.
Although not using ALF, an interesting observation concerning citrate was made during testing of a standard test guideline outlined by Sebastian et al. (Citation2002) which included a modified low Ca Gamble’s solution at pH 7.4 and 4.5, used to reduce inter-laboratory differences. The standard deviation between dissolution rates measured by the nine laboratories ranged from 24 to 61%. Although the high variability was attributed to a number of key test parameters – including flow rate-to-surface area, liquid composition and maintenance of the pH – it was noted that higher citrate concentrations could be used to ensure a more consistent pH (at pH 4.5) and in turn, lower standard deviations. However, the authors indicated that this would not be preferable due to the associated increase in dissolution rates that would not comparable to in vivo observations. Another recent study also employed a modified Gamble’s solution, incorporating citric acid to buffer the pH at 4.5, to determine the dissolution rate of mineral fibres (chrysotile, amphibole asbestos and fibrous erionite) in the phagolysosome environment (Gualtieri et al. Citation2018). While not stated by the authors, the fluid was compositionally similar to ALF.
ALF in the study of particulate matter
Particulate matter and occupational samples have also been widely reported (Guney et al. Citation2017). In one study an enhanced solubility of Cu, Cr and Mn (PM1) and Pb, Cu and As (PM2.5) in particulate matter was observed in ALF compared to samples exposed to the more neutral Gamble’s solution (Wiseman and Zereini Citation2014). Similarly, ALF gave considerably higher metal releases from vehicle exhaust catalysts (Colombo et al. Citation2008), chromated copper arsenate (CCA)-contaminated soils (Gosselin and Zagury Citation2020), Pb-containing urban soils (Hu et al. Citation2019; Li et al. Citation2020), as well as increased rare earth element release from indoor air particulate matter (Gao et al. Citation2020), in comparison to Gamble’s solution. These results imply that inhaled particles would have to be phagocytised before significant dissolution occurs. However, in one specific study of Pt group elements the pH difference between the two fluids had less of a predicted effect. In ALF 23% of Pt and 17% Pd were extracted from the airborne particulate matter compared with 24% Pt and 11% Pd in Gamble’s (Zereini et al. Citation2012).
One particular study found a significant correlation between the bioaccessibility of metals in ALF and their relevant mobile fractions (i.e. soluble and exchangeable, or bound to carbonates) (Gosselin and Zagury Citation2020). Meza-Figueroa et al. (Citation2020) noted dissolution rates in ALF depend not only on mobility in solution but also on the grain size and chemical state of the metal, whereby metal oxides, chlorides and carbonates are known to be largely soluble in lung fluid, whereas metal sulphide, phosphate and silicate groups are generally insoluble, although there are some exceptions. In their study, bioaccessibility appeared to be greatest in samples with the highest content of particulates less than 2.5 µm, leading to the authors to speculate that lung bioaccessibility in ALF solution may depend on particle size distribution and morphology; although this may be expected, given dissolution is impacted considerably by particle surface area (Utembe et al. Citation2015). Nevertheless, Li et al. (Citation2020) gave a similar observation, concluding that lung bioaccessibility of metals in fine particle sizes (<1 µm) was higher than larger size fractions (50–250 µm, 5–50 µm, 1–5 µm), confirming the significance of particle surface area.
Mineralogy was also found to influence the dissolution and pH preferences. In a study of U-bearing dusts, those low in uraninite and high in kaolinite were more soluble in ALF, while those rich in uraninite and carnotite more soluble in Gamble’s solution (Hettiarachchi et al. Citation2019). For Ag NPs, the greater quantity of nitrogen donors, especially when modified to contain DTPA, lead to a greater Ag+ concentration in modified Gamble’s solution than ALF, even though pH is neutral (Zhong et al. Citation2019). The authors concluded this was due to nitrogen donors preventing the formation of AgCl precipitates. While the greater dissolution of Ag in Gamble’s solution appears contrary to the solubility of other metals discussed in this section, this same pH preference is noted for a number of other materials including Co metal and glass wool fibres.
Phagolysosomal simulant fluid (PSF)
An alternative to ALF is a fluid termed PSF; however, its use in the literature has not been as widespread until recent years. PSF uses different buffering reagents to ALF and as such does not contain citric acid, instead buffering the acid environment with potassium phthalate (Stefaniak et al. Citation2005). PSF is based on an early study investigating dissolution of various types of mineral wool, refractory ceramic fibres (RCF) and asbestos fibres (Guldberg et al. Citation1998). Guldberg et al. (Citation1998) sought to modify Gamble’s solution by applying different methods to correct the pH to 4.5 and buffer the solution. The formulations, including addition of hydrochloric acid, phthalate or citrate, appeared to be those already used by test facilities operating within this interlaboratory comparison, and the results of the study comparing these methods were relatively inconclusive; the impact of different fluid compositions was not equal for all fibre types. However, it was concluded that a buffering system was needed, and that the use of phthalate as the buffering system provided a fibre dissolution rate similar to that of the acidified Gamble’s solution, while the use of a citric acid buffering system resulted in a greater dissolution than the other fluids (Guldberg et al. Citation1998). In the design of PSF, constituents such as ammonium ions were removed as their initial purpose was to aid buffering at neutral pH.
PSF has been shown to quite precisely distinguish between dissolution rates across a range of soluble levels, including relatively insoluble TiO2 samples, moderately soluble samples such as kaolin, bentonite and BaSO4, and materials that are easily dissolvable (CuO, ZnO) (Keller et al. Citation2020). The use of PSF has grown considerably in recent years, including in studies examining the influence that fibre binder processes (the inherent addition of phenolic resin in generated insulation wools) have on fibre solubility (Wohlleben et al. Citation2017), as well as in testing the dissolution of Be aerosol compounds, namely Be metal and BeO (Stefaniak et al. Citation2005). It was found that PSF readily dissolves Be particles of 0.9–1.7 μm, with up to 50% dissolved during a 10-day incubation period; larger Be particles of 1.7–2.5 μm, applied at the same mass dose, were not soluble during the same 10-day incubation period (Stefaniak et al. Citation2005). This lack of larger Be particle dissolution was also demonstrated in a J774A.1 macrophage cell line, which implies that the PSF corresponded well with cellular responses. However, no comparative data of J774A.1-induced dissolution of the smaller particles were provided. A follow-up study investigated the dissolution of 20 different Be materials in PSF, including Be ores, hydroxide, oxide and Cu-Be fume. They found the materials associated with chronic beryllium disease to have faster dissolution rates than those with no association (Stefaniak et al. Citation2011). In addition to PSF, Be metal biodurability has also been studied in ALF and neutral Gamble’s solution (Strupp Citation2011). Solubility was faster at the lower pH of ALF than Gamble’s solution, and Be metal dissolution was found to be 3–40 times lower than BeCl2. It should be noted that Be metal, oxides and alloys are only used for very specific applications such as medical diagnostics, electronics and aerospace applications. While their use is not widespread, exposure can lead to sensitisation and dissolution studies have been conducted to investigate the development of chronic beryllium lung disease (Stefaniak et al. Citation2011).
Co metal dissolution in PSF has also been assessed, indicating a higher dissolution in neutral-extracellular fluid (SUF) than PSF (Stefaniak et al. Citation2009). When glycine and phosphate were excluded from the PSF composition, no effect on Co dissolution was observed (Stefaniak et al. Citation2009). This is contrary to the pattern observed with many other metals, however dissolution rates of W compounds – including W metal, WO3 and tungsten blue oxide – were also found to be up to four orders of magnitude slower in PSF compared to SUF (Stefaniak Citation2010). Cellulose nanomaterials and fibres showed little dissolution in either PSF or SUF, which the authors implied was due to the materials being particularly durable and not being cleared by dissolution or intracellular digestion if inhaled, and that mechanical methods of clearance would be required. This was found to closely correlate with other in vivo studies (Stefaniak et al. Citation2014).
In a recent study by Kastury et al. (Citation2018b) investigating the factors influencing metal(loid) dissolution from particulate matter, the extraction efficiencies of ALF and PSF were compared. At the 24 h time point both As and Pb bioaccessibility was significantly higher in ALF than PSF (P < 0.001) (Kastury et al. Citation2018b). This was attributed to the greater presence of chelators in ALF. Within PSF, glycine is the only component known to chelate metals, while ALF contains glycine, citrate, tartrate, lactate and pyruvate which are all known to contribute to a higher metal(loid) extraction capacity (Kastury et al. Citation2018b). In a similar study, chelating agents in ALF were found to have a key role in the degradation of zinc gallate NPs (Lecuyer et al. Citation2020). The NPs were placed in suspension in three different aqueous media for up to three months in order to evaluate their possible degradation (ALF, acetate buffer (pH 4.5) and Milli Q water). Monitoring of the hydrodynamic diameter, charge surface and remaining amount of coating indicated the important role of citrate and tartrate in stabilising the NPs. By stabilising the NPs from aggregating the chelating agents helped maintain a high surface contact with the medium, leading to more effective degradation of the particle core. This is in contrast to rapid aggregation when placed in water. The use of ALF therefore provides a more conservative estimation of inhalation bioaccessibility. Hence, it is possible that using this fluid for material hazard assessments may result in either under or over estimation of the potential hazard depending on the mode of toxicity (i.e. particle persistence or ion release, respectively).
Key components which enable biological accuracy
The main aim of using SBFs is to provide a suitable prediction of in vivo behaviour, and to mimic dissolution mechanisms such as those depicted in . This has been shown to be possible; in bone regeneration studies, for example, the ability of an apatite layer to form on the surface of the material in simulated body fluid (mimicking human blood plasma) has been demonstrated to be predictive of the in vivo bone bioactivity (Kokubo and Takadama Citation2006; Zadpoor Citation2014). Although the formulations of SLFs have been shown to match the biological environments to a degree, there are still disparities when compared with measurements taken in vivo or within cells grown in vitro. In general, few studies compared simulated lung lining fluids with biological systems. More examples are found for lysosomal fluids, primarily with ALF and PSF, showing varying degrees of predictive ability for biological effects. presents studies that have carried out such a comparison and indicates whether they were found to correlate well (+) or poorly (−) with the cellular system, as indicated by the authors.
Figure 3. Selected mechanisms that effect dissolution on the surface of a particle including: (a) complexation, (b,c) redox activities, (d) proton-promoted dissolution and (e) enzymatic action. The prevalence of each dissolution mechanism will be material specific, driven by the chemistry of the materials surface.
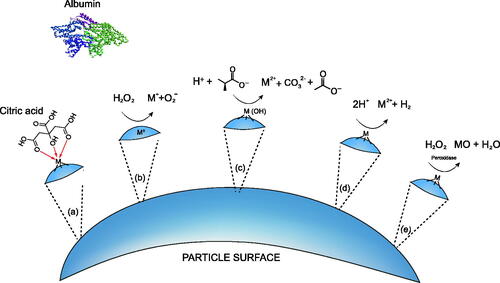
Table 3. Correlation of results from in vitro acellular assays with measurements taken in vivo or within cells grown in vitro.
For example, when Luoto et al. (Citation1994) exposed MMVF to alveolar macrophages they found the dissolution profiles to be different to those obtained in Gamble’s solution (pH 7) or CCM, including high Fe dissolution in alveolar macrophage cell cultures and minimal Fe release in the Gamble’s solution. Issues with precipitation have also been noted for Gamble’s solution, when Alexander et al. (Citation1994) incubated a number of ceramic and MMVF fibres in Gamble’s solution; calcium phosphate deposits were found on the fibres that were not observed in vivo, with modification and a variety of compositional changes including the addition of tris(hydroxymethyl)aminomethane (tris) and removal of some Ca and Mg salts, no deposits were formed and dissolution rates were unaffected. Similarly, Christensen et al. (Citation1994) reduced the amount of Ca in the Gamble’s solution to avoid precipitation of carbonates. A study by Lehuede et al. (Citation1997) compared the solubility of MMVF in rat inhalation studies with those obtained by in vitro dissolution in Gamble’s solution at pH 7.4. A range of fibres were used including historical insulation wools (MMVF 10, MMVF 11, MMVF 21, MMVF 22) as well as highly biosoluble glass and stone wool fibres. The compositional changes observed in the rat inhalation studies – namely a depletion of Na2O, CaO and MgO, and enrichment of SiO2 and Al2O3 – were also reflected in the in vitro Gamble’s solution. While this was the case, morphological changes were not consistent. The in vitro results from one glass wool fibre sample in Gamble’s solution at pH 4.5 was shown to not be indicative of in vivo behaviour. Nonetheless, the authors conclude that this study confirms that in vitro assays closely mirror the behaviour of the tested fibres during in vivo inhalation studies when using Gamble’s solution. Moreover, with an aim to determine compositional and structural influences in MMVF solubility, Guldberg et al. (Citation2002) compared the in vivo half-life (t½) of numerous stone wool fibres to the in vitro dissolution of the same fibres in a modified Gamble’s solution at pH 4.5 already described () (Sebastian et al. Citation2002). Measurements of the t½ after intratracheal instillation or short-term inhalation in rats demonstrated that fibres achieving the Note Q exoneration (HT stone wool fibres) were cleared approximately 10 times faster than a traditional, and biopersistent stone wool fibre, which corresponded well with the decreased dissolution rate (Kdis, in ng.cm−2.h−1) of the traditional stone wool compared to HT fibres, determined in vitro (Guldberg et al. Citation2002). This has also been reflected in studies using a subset of MMVF, HTIW. This category is also used for thermal insulation and includes RCFs and alkaline earth silicate (AES) wools. Unlike RCF, AES have been shown to demonstrate a low biopersistence in vivo (Bernstein et al. Citation1996), due to the presence of alkaline earth oxides, such as Ca and Mg (Brown and Harrison Citation2012). This behaviour has been reproduced using in vitro dissolution studies involving modified Gamble’s solution (Boyles et al. Citation2018; Cannizzaro et al. Citation2019).
In an assessment of Ni metal dissolution, both nano- and micron-sized particles were shown to rapidly dissolve in ALF (80–100 wt%), however this was not replicated in cells (ca. 1–3 wt%) (Latvala et al. Citation2016). Although it should also be noted that the cells used were alveolar epithelial cells (A549), and not the phagocytes which are the cells being modelled when using ALF. A 2018 study did use macrophages and demonstrated that dissolution of some NP in PSF (acquired using abiotic flow-through) were consistent with dissolution within these cell phenotypes, as well as with data from short-term inhalation studies (Koltermann-Jülly et al. Citation2018). The solubility ranking of BaSO4<SrCO3<ZnO was demonstrated in both the acellular and cellular model (using rat NR8383 cells). Moreover, in vivo clearance rates of BaSO4, CeO2, CuO, ZnO and SiO2_amino corresponded to the acellular dissolution in PSF. However, this was not true for SiO2_untreated or TiO2, where a disparity was observed and clearance was far greater than solubility (Koltermann-Jülly et al. Citation2018). Also using macrophages, Gray et al. (Citation2018) found correlation between MoO3 nanoribbon stability in PSF and in intracellular compartments. When incubated in neutral pH fluids (including PBS, SLF or cell culture medium) MoO3 nanoribbons rapidly dissolve, while when in PSF or once internalised by cells MoO3 nanoribbon remain stable and unchanged. A study which observed an increased release of various heavy metals, including Pt and Pd, from road side dust samples (containing Pt(OH)2, Pd(OH)2) in ALF compared to incubation in Gamble’s solution, demonstrated a good correlation to in vivo dissolution (Artelt et al. Citation1999; Colombo et al. Citation2008). A 30 day study of the dissolution of Pt group elements from vehicle emissions identified that approximately 36% Pt was released when incubated in ALF (Colombo et al. Citation2008). This corresponded well with a nose-only inhalation study conducted in Lewis rats. The animals were exposed to 4 mg/m3 for 5 h/day, 5 days/week for 90 days, during which time 31.4% of Pt was bioavailable (Artelt et al. Citation1999). However, when a higher inhalation concentration was used, or when intratracheal instillation was used, the bioavailability of Pt was found to be far lower, demonstrating that uncertainty still exists in the corroboration of in vitro assessment using current SBFs (Artelt et al. Citation1999). This implies there are other crucial factors in the replication of biological systems that are not accounted for in the current in chemico methods for assessing dissolution and it is therefore understandable why uncertainties remain with the strength of association to in vivo responses. What these might be is yet undetermined, but it may be helpful to assess the relationship between attaining biological relevance with the degree of SBF complexity.
A recent study gave a useful comparison of four frequently used SLFs and their ability to mimic in vivo results (Zhong et al. Citation2020). In the attempt to optimise in vivo-in vitro correlation, intranasal instillation experiments were compared with in vitro bioaccessibilities obtained from a variety of SLFs. Balb/c mice were exposed through intranasal instillation (20 µg/µl) to PM2.5 suspensions spiked with a variety of Pb compounds (PbSO4, Pb3O4, PbS, PbCrO4, PbO, PbCO3 and Pb(NO3)2) and the relative bioavailability of Pb was determined 48 h later. These were compared to in vitro Pb bioacessiliblities determined through static dissolution experiments in Gamble’s solution, SELF, ALF and SLF. Large variations were found between the four fluids, even when influencing parameters such as the surface-to-liquid ratio was kept constant. All in vitro bioaccessibilities were far below the relative bioavailability determined in vivo. Of the four fluids tested, Gamble’s solution provided the closest correlation and this was further improved with the addition of DTPA, a strong chelator, leading to a formulation similar to that of SUF as has been described above. The authors suggested DTPA to be a key component that acts as a substitute for chelators present in real lung fluid, implying an increase in the complexity of Gamble’s solution is desirable. Further discussion on the presence of complexations agents, antioxidants, enzymes, and organic content in general follows below.
Organic content
Some state that the typical formulations for SLFs indicate sufficient salt content but lack a representative mix of organic molecules that are native to the respiratory tract environment (Boisa et al. Citation2014). The presence of chloride, sodium and a number of salts (including sodium bicarbonate, magnesium chloride hexahydrate and sodium sulphate) in most SLFs are a suitable match in both identity and concentration to a healthy human (Joris et al. Citation1993; Jayaraman et al. Citation2001a). However, components such as organic acids, proteins and surfactant lipids are not incorporated into most simulants, even though the in vivo concentration of these components are known. Physiological relevance has been a central issue in the use and modification of SBFs over the years and the lack of accurate representation of the organic molecules found in vivo can lead to the use of fluids that are starkly different from that of real lung surfactants. Macromolecules such as catalase (Cantin et al. Citation1990) and phospholipids (e.g. phosphatidylcholine), are abundant within in vivo lung lining fluid and smaller organics such as ascorbate, urate, glutathione and α-tocopherol (van der Vliet et al. Citation1999) are also found, exhibiting antioxidant properties. A number of studies have made progress to replicate these organic constituents with the inclusion of the major proteins and lipids such as urate, ascorbic acid, DPPC, albumin and mucin (Berlinger et al. Citation2008; Son and McConville Citation2009; MacCuspie et al. Citation2011; Boisa et al. Citation2014).
Coufalik et al. (Citation2016) examined the effect of DPPC addition to Gamble’s solution, in a comparison of a variety of fluids including ALF, a saline solution and a new SLF termed simulated alveoli fluid (SAF). The presence of DPPC has been shown to reduce the surface tension of SLF, acting as a surfactant; a desirable trait due to the low surface tension of native lung surfactant in normal lungs (Hassoun et al. Citation2018; Leiby et al. Citation2020). For hydrophobic materials, the inclusion of DPPC can increase the wettability and prevent aggregation, as demonstrated in studies of inhaled pharmaceutical formulations (Davies and Feddah Citation2003; Son and McConville Citation2009). In these, a concentration-dependent increase in solubility was observed with the addition of DPPC (Davies and Feddah Citation2003; Son and McConville Citation2009). Likewise, DPPC was shown to stabilise Ag NPs against aggregation, without significantly effecting the total Ag release (Leo et al. Citation2013). This was consistent with results from Maccuspie et al. (Citation2011) who noted a subtle stabilisation of Ag NPs accredited to DPPC, however higher stability was found with the inclusion of bovine serum albumin (BSA). This stabilisation of particles as a result of proteins has also been noted elsewhere, and with this, associated effects upon dissolution have also been observed. It is possible that the binding of proteins to particle surfaces will inhibit the potential for dissolution; this has been observed for AgNPs with the addition of BSA (Holmes et al. Citation2016) and of serum proteins (Huk et al. Citation2014). As described earlier, the association of particles with proteins will result in protein corona formation. The composition of this corona has been shown to vary dependent on protein source and condition (Lesniak et al. Citation2010), as well as on particle parameters such as size (Lesniak et al. Citation2010) or surface charge (Boyles et al. Citation2015a). It is therefore prudent to consider which proteins are likely to be present within the environment being replicated, and how abundant they may be, not only for their ability to induce dissolution, but also for their ability to reduce dissolution. Intriguing is the possibility that even the harsh conditions of the phagolysosome may not disrupt this protein coating. The measured transition of particles through an endocytic route of internalisation has allowed mapping of how bound proteins may behave within these environments; while the soft corona proteins bound to silica-coated magnetic nanoparticles were shown to change dependent on location, i.e. dependent on organelle, the hard corona was consistent before internalisation and throughout the intracellular trafficking (Bertoli et al. Citation2014).
For hydrophilic materials, the effects of DPPC are limited; as demonstrated in the investigation of pulmonary delivery of insulin. Inclusion of DPPC in the SLF had no effect on poly(lactide-co-glycolide) (PLGA) solubility, due to its hydrophilic nature (Ungaro et al. Citation2009).
In addition to the organic content, other factors such as pH, ionic strength, temperature, precipitation, hydrolysis and presence of complexing agents all influence the dissolution behaviour of the fluid. When relevant to the simulation of biofluids these have been discussed below.
Chelating agents
The importance of other specific properties of the SLF on material solubility have been studied, including which chelating agents are present (and at what concentrations), and the presence of precipitate forming constituents (e.g. phosphates, carbonates), and non-reacting ionic materials (Ansoborlo et al. Citation1999).
Citrate, oxalate, tartrate, and EDTA
Citrate is used in many SLFs as a replacement for proteins, and more specifically, proteins that act in the mobilisation of Fe; the activity of organic ligands is to bind to Fe reducing its ability to form HOOH, with citrate being the dominant ligand for Fe (Charrier et al. Citation2014; Hernandez-Pellon et al. Citation2018). It also has a practical purpose of reducing the foaminess which occurs when proteins are introduced to SLFs (Marques et al. Citation2011), and is already known to be naturally present in biological systems; Collier et al. (Citation1992) notes physiological conditions generally fall between 0.1 and 0.3 mM. This gives an upper range of 0.058 g/L (citrate) and 0.077 g/L (sodium citrate), and shows that many of the existing simulant fluids already adhere to this range (including Gamble’s solution, SUF, SELF, AAF and SILF). However, due to its known activity as a complexing agent, the appropriateness of using high concentrations of citric acid can be questioned, as there may be concerns with overestimation of solubility. Generally, an increase in material solubility is the behaviour most often observed when citric acid is used within an SLF, and concentrations as low as 1 mM have been demonstrated to fractionate various metals from particulate matter (Mukhtar and Limbeck Citation2013). One study noted a direct correlation between increasing citrate concentrations and in vitro Co2O3 dissolution rates (p < 0.02) (Collier et al. Citation1992). The same was not seen for increasing bicarbonate concentrations (p > 0.32), which may indicate that bicarbonate did not coordinate to Co as citrate did in enhancing dissolution.
The complexing activity of citric acid was further confirmed by Rozalen et al. (Citation2013) in the assessment tremolite asbestos solubility a simple “modified Gamble’s solution” (NaCl and Na2SO4). The pH was controlled with either sodium bicarbonate or nitric acid to attain pH 4, pH 7.4, and pH 5.5, and a strong relationship between pH and dissolution was observed, with a reduction in pH leading to an increase in dissolution. With the addition of organic ligands, either citrate or oxalate, at concentrations of 0.15, 1.5 and 15 mM, dissolution was enhanced. However, for citrate this was only observed for the acidic pH solutions, which caused a strong and citrate-dose-dependent effect on dissolution. This implies a two-step process; first acid-aided dissolution of the tremolite releases free metal ions and these are subsequently chelated by the citrate species. The effect of oxalate was also strong when included in acidic conditions, but was not found to be dose-dependent. However, a strongly oxalate-dose-dependent effect was observed at the neutral pH conditions (Rozalen et al. Citation2013). EDTA, another complexation agent, was also found to encourage asbestos dissolution due to its chelation of Fe (Lund and Aust Citation1992). For Mg-containing materials such as tremolite, these ligand-specific differences may be due to speciation. In the presence of oxalate, there is a consistent formation of a single magnesium oxalate species which remains stable from pH 4–12. However, for citrate, not only is there an enhanced and stable formation of magnesium citrate (MgCit−) from pH 5 onwards, numerous other species (e.g. MgHCit and MgH2Cit+) form, peaking at pH 3–4 and falling by pH 6 (Rozalen et al. Citation2013). Barly et al. (Citation2019) also attributed high stone wool fibre dissolution in acidic-modified Gamble’s solution to the complexation effects of citrate and tartrate, namely on Fe and Al, and determined that dissolution rates were significantly faster than in solutions of similar pH where no complexing agents were present. Likewise, Steenberg et al. (Citation2001) found that citric acid greatly increased dissolution of stone wool fibres, which was not observed with the addition of acetic acid.
Many other examples of this phenomenon can be found in the literature (Hedberg et al. Citation2010; Rozalen et al. Citation2013; Boisa et al. Citation2014; Utembe et al. Citation2015; Barly et al. Citation2019; Zhong et al. Citation2019). In fact, an excellent correlation was shown between ALF and a sodium citrate buffer, suggesting that no other components of ALF contribute significantly to the extraction efficiency (Mukhtar et al. Citation2015). Therefore, the use of a sodium citrate buffer could be a simple and suitable alternative to ALF. When separated into the individual components of ALF, the effect of citric acid solution at the same pH has been shown to cause equal dissolution of various metals from stainless steel powders when compared to complete ALF solution; with differences in the dissolution kinetics induced by equal quantities of sodium citrate, –tartrate and –lactate found to be dependent on functional group availability (Hedberg et al. Citation2011). This highlighted the importance of complexation capacity, as dissolution was shown to decrease as the complexing agent (citric acid) was reduced. However, these effects were shown to be selective and dependent on which metal was assessed; the dissolution of Fe, Cr and Ni varied according to the citric acid concentration, while dissolution of Mn did not and was shown to dissolve readily in the non-organic components of ALF (Hedberg et al. Citation2011).
Beeston et al. (Citation2010) also highlighted the competing effects of citrate (and minor influences from tartrate) in ALF, attributing them to a high level of PbS (galena) NP dissolution at pH 4.2. When a similar study of PbS was carried out in Gamble’s solution (pH 7.4) no Pb leaching was observed and the authors specifically attributed this to insufficient citrate concentrations, however, the impact of pH cannot be ruled out here since metal sulphides can react with acid. Similarly, a recent study by Chakraborty et al. (Citation2018) associated a high proportion of organic Cu complexes in ALF and SBF with significant Cu dissolution from CuO NPs. The authors concluded that ligand-promoted dissolution was the primary mechanism responsible for CuO dissolution. In a study of UO3 breakdown, complexing agent sodium citrate caused a high level of dissolution up to 60 times faster than Gamble’s solution (Ansoborlo et al. Citation1990, Citation1992).
Glycine
In the extraction of Ag from contaminated soils using both ALF and Gamble’s solution, Gamble’s was shown to induce greater Ag release. It was proposed that a higher glycine concentration, acting as a chelating agent within Gamble’s solution, was responsible for this, and moreover, that in the case of Ag, citric acid within ALF may have acted in a stabilising role (Cruz et al. Citation2015). The capacity of glycine to act as a metal chelator is, however, very much metal-dependent; there are other amino acids, including histidine, which have been shown to leach metals such as Fe, Cr, Zn and V from coal fly ash more effectively than glycine, while glycine was found equal to in its ability to leach Al, Mn, Cu, and Ni when compared to other metal chelators (Harris and Silberman Citation1983).
Lactate
The chelation of cations by lactate has also been demonstrated in the study of lithium intercalation compounds (complex metal oxides containing Li, Ni, Mn, Co). Dissolution in bacterial medium with lactate, compared to the lactate-free alternative, noted an increased dissolution of transition metal components. For example, dissolved Ni was 2.5 time greater in the presence of lactate (Buchman et al. Citation2020).
It is important to note that complexation is not unique to simulated environments and is actually a phenomenon that is observed in vivo in the binding of metals by glycoproteins such as albumin and mucin (Cooper et al. Citation1985; Duranti et al. Citation2001; Bal et al. Citation2013). Albumin has been shown to be important in the transport of Cu ions (Bal et al. Citation2013) and both albumin and mucin have been shown to increase the leaching capacity of a biofluid simulation for a number of metals (Berlinger et al. Citation2008). Therefore, the effect of citric acid, or indeed other complexing agents, should not be treated as biologically irrelevant and instead should be evaluated to ensure the extent of complexation matches that occurring within the lung, particularly when multiple complexing agents are present. In particular, consideration should be given as to whether citric acid at high levels is the best simulant for biologically relevant complexation, particularly due to the discussed concerns around aggressive dissolution (Morgensen Citation1984), non-linear or selective effects (Morgensen Citation1984; Hedberg et al. Citation2011; Barly et al. Citation2019), and how these impact predictivity and robustness; the level of predictivity that have been observed is considered later.
Presence of antioxidants
Citrate can also function as an antioxidant when included in SLFs, providing a direct replacement of the numerous antioxidants present within the extracellular fluid of the respiratory system, including mucin, uric acid, protein (mainly albumin), ascorbic acid, and reduced glutathione (GSH) (Cross et al. Citation1994). However, the activities of antioxidants may not be addressed in such a simplistic model, as both the extracellular space and intracellular compartments of the lung are under conditions that readily promote ROS production (Kirkham and Rahman Citation2006). Lung lining fluid is known to contain antioxidants (Charrier et al. Citation2014) which are recognised to aid in the detoxification of inhaled material before it interacts with cells and tissues (Krawic et al. Citation2017), raising the question of whether this should be a standard component in any SLF, especially as many materials have the capacity to generate ROS themselves, including transition metals such as Cu and Fe (Charrier et al. Citation2014; Kuang et al. Citation2017). It is hypothesised that Fe has a contributory role in oxidative damage (Greenwell et al. Citation2003), suggesting that particles or fibres rich in Fe may increase the production of ROS. The mechanism involved in adverse health effects caused by inhalation of materials is thought to be related to these metal-mediated generation of ROS which can lead to oxidative stress within cells or tissues by oxidation of nucleic acids, proteins and lipids (da Silva et al. Citation2015). One example demonstrated Fe(II) (from FeSO4) to be especially involved in the generation of the highly reactive hydroxyl radical (*OH) in SLF (Vidrio Citation2009). Similarly, Gosselin and Zagury (Citation2020) found a highly significant correlation between bioaccessible Cu and the oxidative potential of PM20 in CCA-contaminated soils (containing CuO), leading to the conclusion that the mobile Cu fractions are particularly important when considering ROS. However, this has yet to be verified by in vivo studies. Observations by Stefaniak et al. (Citation2009) indicated that production of free radicals (*OH and O2*) depended not only on the materials surface chemistry but also the biological liquid media, highlighting the importance for accurate simulants for hazard classification. However, a study of oxidation-dependent pyrite dissolution demonstrated that addition of a modified bovine pulmonary surfactant (Survanta®, containing (phospholipids, neutral lipids, fatty acids, and bovine surfactant proteins) to the SLF did not affect or reduce the rate of dissolution (Harrington et al. Citation2012). This was somewhat surprising as it was expected that the inclusion of redox active biomolecules would provide additional pathways for ROS to react and therefore less ROS would be present and a decrease in ROS-aided dissolution would be found, this was not the case. However, when compared to water, the dissolution rate in simple SLF significantly decreased, and concentrations of hydrogen peroxide were observed to be higher. The authors concluded that components of the simple SLF (such as phosphate, glycine, citrate) may be sufficient to determine the biopersistence of pyrite (Harrington et al. Citation2012).
If concerns still exist around the use of citric acid, alternatives can be used to maintain the lower pH of ALF. Alternatives that have been reported include acetic acid (Steenberg et al. Citation2001), glycine (Stefaniak et al. Citation2005) and lactate (Ramos et al. Citation2011). Acetic acid was shown to have no impact on the dissolution of stone wool fibres (Steenberg et al. Citation2001). For an alternative solution buffer, potassium hydrogen phthalate has been used, as in the aforementioned PSF. This was demonstrated in the dissolution of Be metal and oxide in potassium hydrogen phthalate-buffered ALF, where results reflected the dissolution rate in mouse macrophage-like cells (Stefaniak et al. Citation2005). This formulation has also been suggested in an ISO technical report for assessment of nanomaterial biodurability (ISO Citation2017).
Enzymatic activity
Cell-derived peroxidase enzymes have been shown to contribute to redox-related dissolution. Although macrophages are resident within the alveolar space of the lung and are therefore “first responders” to exogenous material, it is another phagocytic cell type (neutrophil) which is rapidly recruited upon exposure. These cells are highly redox active and have been shown to be important in the degradation of materials including carbon nanotubes (CNTs), in the lung. Macrophages were shown to be less proficient than neutrophils at degrading the single-wall CNTs, and this was considered to be due to macrophages containing the lower amount of peroxidase enzymes (mainly myeloperoxidase (MPO)) than neutrophils (Andón et al. Citation2013). There are several studies which have been designed to model the action of neutrophils and redox activities. One study demonstrated the incubation of CNTs with natural horseradish peroxidase (HRP) and hydrogen peroxide (H2O2) leads to increased degradation of the nanotube structure (Allen et al. Citation2008). Liu et al. (Citation2010) supplemented PSF with HRP/H2O2 and observed degradation of the CNTs to short tubes. No such shortening was observed for PSF medium without H2O2. This observation indicated that the CNT structure could have been oxidised and hence weakened, leading to breaking down into shorter tubes. H2O2 was used as it is an oxidant produced in phagocytes (Hampton et al. Citation1998). HRP is plant-derived, however, human peroxidases, such as MPO have been shown to be just as effective, and in the presence of H2O2 can induce the degradation of single-walled CNTs, whereas individual components were ineffective (Kagan et al. Citation2010). The relevance and impact of MPO on CNT dissolution has not only been shown in chemico, MPO-knockout mice have been used to demonstrate a decline in CNT clearance after instillation within the lungs associated with a lack of MPO (Shvedova et al. Citation2012). These effects were observed in partially oxidised carboxylated CNTs, however, pristine CNT, with no functionalisation are also shown to be soluble, but not solely through the activity of peroxidase. Instead, Fenton-chemistry related processing of H2O2 forms further oxygen radicals that can induce oxidation (Allen et al. Citation2009). Once oxidised, in the presence of H2O2, CNTs can be broken down by peroxidases (Andón et al. Citation2013). The activity of peroxidases is through redox cycling (O'Brien Citation2000; Kotchey et al. Citation2013), and as such, CNTs can be considered particularly prone to degradation by peroxidases, through the direct targeting of oxygen moieties present of CNT surfaces (Kotchey et al. Citation2013). Functional surface groups may be present upon synthesis, increased through acid wash, or removed with treatments such as high-temperature annealing (Boyles et al. Citation2015b). As a result peroxidase activity may vary for different CNT sources, and this has been shown to be the case when investigating pristine CNTs which do not present surface defects and were not susceptible to peroxidase degradation (Allen et al. Citation2009). This is not to say that other materials would not be vulnerable to peroxidase activity, but it would focus on materials with oxygenated surface groups such as carboxyl, carbonyl, or hydroxyl groups.
Further evidence highlighting the impact of enzymatic activity on dissolution, other than peroxidase activity, can be found in the assessment of para-aramid fibres. This was first noted by Searl and Cullen (Citation1997) when performing routine tissue digestion during in vivo fibre counting studies. With para-aramid being reactive to traditional digestion techniques (such as use of sodium hypochlorite, potassium and sodium hydroxide, hydrochloric acid and hydrogen peroxide), enzymatic digestion was applied, and was shown to be unsuccessful as it appeared to cause dissolution of para-aramid fibres. This was later followed up by Warheit et al. (Citation2000), who identified that exposure to the enzyme cocktail is not sufficient alone to cause biodegradation, but it is a component of lung lining fluid that is required as a prerequisite to enzymatic digestion of para-aramid fibres, and provides a “priming” of fibres making them susceptible to enzymatic activity. A follow up study demonstrated that it is potentially the protein components of lung lining fluid (using heat inactivation of proteins in BAL fluid) that play some (but not all) of the role in this “priming” activity (Warheit et al. Citation2001). The enzyme cocktail used in these studies was collagenase, papain, DNase and lipase. An important material distinction in these studies was evident, in that cellulose fibres were consistently shown to be more durable in response to these treatments than para-aramid fibres. This was also confirmed using in vitro macrophage experiments (Warheit et al. Citation2001), and highlights again the risk in assuming operational validation can be transferred to untested material, and provides some evidence in the need to achieve adequate biological replication to always have confidence in in chemico findings. In doing so however, consideration of appropriate enzymes and conditions of said enzymes is important. For example, the collagenase, lipase, and DNase mentioned above are active at different pH levels, pH 6, 4.2–4.5, and 4.5, respectively (Tappel Citation1969).
Mechanisms that effect dissolution
summarises the main mechanisms that effect dissolution at the surface of a material, as has been highlighted in the reviewed literature: namely complexation (mechanism (a)), redox activities (mechanism (b,c)), proton-promoted dissolution (mechanism (d)) and enzymatic action (mechanism (e)). Examples from the literature have been discussed in the relevant sections above. While this is the case, a number of important distinctions are necessary. Firstly, the term proton-promoted dissolution has been used to describe the increased release induced by low pH (Rozalen et al. Citation2013). This has previously been referred to as “protonation” (Hedberg et al. Citation2011), however this is not strictly true as most cases do not involve the direct transfer of a proton onto an “acceptor”. Instead, with pure metals the proton acts as an electron-acceptor, increasing the metals oxidation state (oxidation) and evolving hydrogen gas, as shown in . For metal oxide(-containing) materials, the reaction is simply neutralisation between an acid and a weak base (metal oxide) evolving metal ions and water. Secondly, it is important to note that the examples given are generalised and the prevalence of each dissolution mechanism will be material specific, driven by the chemistry of the materials surface. Ag has been shown to be particularly susceptible to redox reactions due to the less stable Ag2O (mechanism (b), ). However, this will not be the case for all metals (Sigg and Lindauer Citation2015), and the metal ion release mechanisms differ depending on the material chemistry. The ion release from metal (zero valent) particles is different from metal oxide particles, as a redox reaction is first involved in the acid dissolution of metal particles. One classic example is that Cu metal particles should have no reaction with a non-oxidative acid (such as HCl and weak organic acids, citric/acetic acid) but could be dissolved in an acidic medium by first being oxidised with dissolved air (accelerated by the increased surface area for small particles), producing a metal oxide which then reacts with acid in an acid–base reaction.
Is fluid complexity desirable?
From the literature already discussed, it is evident that no single factor is responsible for dissolution within SLFs. There is no clear consensus as to whether a more complex fluid, accounting for multiple influencing factors, is desirable or not. Ideally, such an analysis would be based on how well the data correlates with biological systems; however, the availability of appropriate in vivo data for comparison is limited, particularly for extracellular fluids. In its absence, presents studies that compare complex fluids with their simpler counterpart, and indicates whether the more complex fluid caused less (−), equal (0), or greater (+) dissolution. The results appear to be material specific; however, there is a general preference from increased dissolution in more complex fluids. This is likely related to the increased organic content and complexation discussed above.
Table 4. Studies comparing “simple” and complex fluids, including an indication of whether the more complex fluid caused less (−), equal (0), or greater (+) dissolution.
The decision to utilise a simple or complex fluid may in fact be largely dependent on another factor however, and this relates to the dissolution testing system being employed, as each the static, flow-through, and tangential flow systems each have unique requirements and complications. In short, flow-through and tangential flow systems can be used to alleviate issues of saturation and solubility limits that are present in static test conditions. This has recently been shown critical to allow the correlation between in vivo pulmonary clearance and acellular dissolution testing of BaSO4. Static testing of BaSO4 in PSF was shown to maintain relatively unchanged particles over relatively long periods of time (up to 2 years), while using either a flow-through or flow-by system resulted in the close correlation between dissolution in vitro and in vivo clearance (Keller et al. Citation2020). These dynamic systems allow the SLF to be constantly replenished, thus removing any dissolved ions and therefore shifting the local equilibrium around the test materials, potentially increasing dissolution. For this reason, even if the same SLF is used, the results will be influenced by the methods employed; however full consideration of methodological influences is out of the scope of this review.
Practicalities of performing the assessment of material solubility
Precipitation
In addition to fluid complexities, there are practical issues relating to how biofluids are prepared, stored, delivered and tested. In a large proportion of the literature, especially relating to Gamble’s solution, the preparation of SLF is carried out in a specific order, where salts of Ca are added at a later time to prevent premature precipitation. Bohner and Lemaitre (Citation2009) discussed the procedure for the preparation of SBFs and highlighted a number of factors that may limit the reproducibility of results. These primarily focussed around measures that may influence or promote the formation of solution precipitates. For example, preparing the solution at 37 °C can increase the risk of premature calcium phosphate precipitation. In addition, some components in the SBF (e.g. CaCl2) have the ability to complex with materials that are bioactive, for example generating hydroxyapatite (HA), as demonstrated in bone regeneration studies. A study by Gosselin and Zagury (Citation2020) found low recovery for Cr, Zn, Fe and Pb in spiked Gamble’s fluid due to precipitation of solubilised compounds, highlighting a possible issue in using this SBF for inhalation bioaccessibility assessments for certain metals. However, it was suggested that this may be alleviated by maintaining the pH at 7.4 throughout the duration of the experiment; hence, use of a flow-through system may be preferable in these instances. In other studies the presence of phosphate has been shown to prevent dissolution due to the formation of an insoluble salt, for example cobalt phosphate in the study of cobalt carbide particles (Stefaniak et al. Citation2009), or lead phosphate from mining activity (Wragg and Klinck Citation2007).
Precipitation has also been reported by Suetake et al. (Citation2019) who noted a calcium phosphate layer forming upon extended contact of cesium-rich microparticles with the SLF. This was attributed to the super saturation of SBF towards apatite formation. Bohner and Lemaitre (Citation2009) also pointed to the fact that solutions are rarely filtered at any point, despite insoluble contaminants being known to have a significant effect on. The carbonate content in solutions is rarely controlled even though it can affect both the pH and saturation levels of phosphate and carbonates. Wiseman et al. (Citation2018) noted the pH of Gamble’s solution to be pH 7.4 when bubbled with oxygen or carbon dioxide, however in ambient conditions this increased to 8.2–8.7. Another author discussed using nitric acid for pH adjustment, instead of sodium hydroxide, in order to keep the original composition of the SLF (Hernandez-Pellon et al. Citation2018).
Commercially available fluids
An alternative to preparing and storing these complex solutions within a laboratory that operates dissolution studies is to purchase solutions that are commercially available. These can be either complex salt solutions or solutions that are used specifically for the maintenance of mammalian cells in culture. One consideration when assessing SLFs is their robustness and ability to provide reproducible results in known test methods. In this respect, commercially available fluids are advantageous as they allow fluids of known and consistent compositions to be used, removing the potential negative impact of this variable from the test preparation.
The use of these commercially available products has previously been applied in dissolution studies. For example, Hank’s balanced salt solution (HBSS) has been used in testing dissolution of clay materials (Baek et al. Citation2011), and phosphate-buffered saline for numerous metals (Pelfrene et al. Citation2017), while various CCM solutions have been used to assess solubility of CuO NPs (Semisch et al. Citation2014; Boyles et al. Citation2016), workplace dust containing UF4 (Chazel et al. Citation2000), Hg mine-waste calcine (Gray et al. Citation2010), and UO3 particles (Ansoborlo et al. Citation1992). In the static solubility testing of UF4-containing particles generated in a fuel factory, Chazel et al. (Citation2000) compared Gamble’s solution to CCM that contained 10% foetal calf serum (FCS). Although the type of CCM was not identified, it was shown that particles dissolved more rapidly within Gamble’s solution than in CCM during the first three days of testing. However, by day seven the dissolution in CCM equalled that in Gamble’s, and then surpassed it during the further three weeks of testing (Chazel et al. Citation2000). Conversely to this, breakdown of UO3 has been shown to be vastly greater in CCM when compared to Gamble’s solution, with a half-time of 4 days in Eagle’s basal medium (EBM) compared to 70 days in Gamble’s solution (Ansoborlo et al. Citation1992). The contrast in dissolution rates within CCM and Gamble’s was further illustrated by Gray et al. (Citation2010), who demonstrated that Hg mine-waste calcine may leach greater or lesser Hg in supplemented RPMI-1640 compared to Gamble’s, dependent on source of mining. CuO nano- and micro-particles, with average diameters of 55 nm and 1.3 µm respectively, were assessed in different fluids all at pH 7.4, including artificial alveolar fluid (AAF), H2O and CCM (DMEM); CCM was tested with and without FCS (Semisch et al. Citation2014). Regardless of particle size, the same pattern of dissolution was observed, with dissolution in DMEM greatly surpassed that in other fluids, second to this was in CCM containing FCS, then in H2O, and lowest dissolution was observed in the AAF. The literature on the use of commercially available fluids is inconclusive, and does not indicate it, or components of, to be particular advantageous compared to other SLF compositions. However, if fluid robustness was considered a priority in the future design and refinement of SLFs, the use of such fluids provides a simple and available method to do so.
Element detection
The application of physiological fluids has also created problems during trace element determination (Mukhtar et al. Citation2015). This is particularly true in fluids where organic components have been added to better mimic human biological conditions. Some have reported the SLF to be too saline for measurement with inductively coupled plasma spectrometry, including ICP-AES and ICP-MS (da Silva et al. Citation2015). This is particularly true when components such as Na are present in high concentrations and it can be difficult to obtain a good resolution for detection (Foy et al. Citation2003). One study employed a lower saline fluid that was approximately 3.5 times lower than Gamble’s solution (2922 mgL−1 total dissolved salts compared with 10 200 mgL−1). This was used for metal bioaccessibility studies and compromised sodium chloride, ascorbic acid and sodium citrate (pH 7.4). The results indicated the bioaccessibility of Cd and Co to align with literature values, however other metals (including Cu, Mn, Pb and Zn) were underestimated in a lower saline solution (da Silva et al. Citation2015).
Conclusions
This review provides an overview of SBFs that are used to simulate the extracellular and intracellular compartments inhaled materials are likely to encounter; namely lung lining fluid and lysosomal fluid. Considering the range of fluids reported in the past 60 years, we have identified specific components, where possible, that play a central role in material dissolution behaviour, such as organic molecules, redox-active species and chelating agents. An emphasis is placed on biological relevance and specific fluids that differ from, or suitably replicate, the in vivo environment have been examined.
The current body of literature has identified that particle and fibre dissolution is not always driven by pH, and likewise it is not only driven by fluid composition. When elucidated, there have been specific materials and specific formulations which correspond with specific dissolution mechanisms. For example, the presence of phosphate in the fluid has prevented the dissolution of specific materials due to the formation of insoluble salts such as cobalt phosphate (Stefaniak et al. Citation2009) and lead phosphate (Wragg and Klinck Citation2007). The influence of oxygen was particularly important in UO3 breakdown and similarly, the presence of peroxidase enzymes for CNT degradation. Fe(II) has been demonstrated to be especially involved in the generation of the highly reactive hydroxyl radicals, and therefore the antioxidant potential of the SLF will be important for Fe-containing materials (Vidrio Citation2009). Due to these material specific results, it would be pragmatic for further SLF developments to focus on how well the fluid matches the biological environment. With better biological mimicry, results are more likely to reflect in vivo behaviour regardless of the material under investigation. However, such efforts would result in a fluid of far greater complexity; and as eluded to above, this may affect the practicalities of performing the test in the current testing systems. Therefore, a rational approach is needed to ensure a balance between bio-relevance and usability.
One aim of this review was to establish what would be the most useful approach in simulating biological fluids for predicting a hazard posed by exogenous materials, including whether to focus on biological mimicry, operational validation, or method robustness. While these goals are distinct, they are inherently interlinked – and often conflicting – and therefore need to be considered holistically. For example, the design of a robust fluid does not necessarily ensure its ability to be predictive. Likewise, while the assumption that improving biological replication will enhance in vivo correlation may hold true, the associated procedural and analytical implications need to be considered if a SLF is to be practically useful. It is clear from the literature that there have already been numerous attempts to make SLFs more physiologically relevant; however, the lack of systematic comparisons (between fluids mimicking the same environment) and in vivo corroborations, such as that of Zhong et al. (Citation2020), makes it difficult to determine if better “realism” is actually achieved. For this reason, validating against historic in vivo findings should remain central in the assessment and development of these fluids. Only with clear in vivo correlation will such methods be successful in standardisation and regulatory contexts. While certain SLFs have shown promising correlations, for example ALF (Colombo et al. Citation2008; Hamilton et al. Citation2018; MilosevicAc et al. Citation2019) or PSF (Stefaniak et al. Citation2005; Gray et al. Citation2018; Koltermann-Jülly et al. Citation2018; Keller et al. Citation2020), disparities still exist making it difficult to confidently recommend certain fluids or components, over others. We therefore conclude that further research in this area is needed and should be done systematically, using materials and methods that facilitate comparison against in vivo, or cellular in vitro, results.
Another option could be the development of a sequential testing strategy, as has been done to achieve robust GIT models. The conceptual and practical elements used in these approaches (such as the UBM or FOREhST models) could be considered when discussing how to develop a robust model for dissolution within the respiratory system. One such element is the use of sequential exposures of the material to mimic the sequential passage of material through the GIT. This approach could be emulated when developing a lung model. Versions of this approach have already started to be reported, including an inhalation-ingestion bioaccessibility assay (Kastury et al. Citation2018b, Citation2020) and mucus-oral extraction test (Alpofead et al. Citation2017). While promising, the implications of a sequential strategy on the calculation of a dissolution coefficient would have to be considered as this may limit the comparability of the data obtained to historical information. Likewise, while the environments and parameters are known – for example, the change from lung lining fluid to intracellular compartments, and changes of pH or in fluid composition – there remains many other factors to consider such as internalisation speeds, residence time and mucociliary removal. Such a strategy would be complex, and may not be a priority considering the promising in vivo-in vitro correlations that have been reported, and noted within this review.
Overall, to function as a method to access bioaccessibility or biopersistence, it is important that SLFs used are reliable and robust, to allow for confidence when making comparisons over time and against different studies.
Glossary
Bioaccessibility – The fraction of a substance that dissolves under surrogate physiological conditions and is therefore potentially available for absorption into systemic circulation or interaction with local sites
Bioavailability – The proportion of a substance considered to be extracted in the gastrointestinal tract or lungs compared with the total substance that has been ingested or inhaled.
Biodurability – The tendency to resist chemical and biochemical alteration through dissolution and enzymatic biodegradation or chemical disintegration within biological media leading to structural alteration
Bioelution – As a measure of the bioaccessibility of a substance, bioelution refers to in vitro extraction methods that are used to assess the extent to which a substance is released into artificial biological fluids
Biopersistence – The ability of a material to persist in the body due to its biodurability and in spite of physiological clearance mechanisms
Clearance – Removal of deposited particles from the respiratory tract by various processes depending on both the physical and chemical characteristics of the material.
Degradation (EFSA, in relation to nanomaterials) – as used herein is the process by which a nanomaterial is converted to degradation products in the form of a nanomaterial or to solutes with the loss of nano features. Oxidative dissolution of silver nanoparticles with the release of Ag + ions is a relevant example.
Dissolution (EFSA, in relation to nanomaterials) – as used herein is the process by which a soluble nanomaterial in an aqueous medium or biological environment is converted to the constituent ions or molecules with the loss of nano features.
Dissolution (OECD) – The release of ions or molecules from the surface of a solid and the subsequent distribution of the ions or molecules throughout the available liquid volume as a result of entropy.
Acknowledgements
The authors would like to acknowledge and thank the European Insulation Manufacturers Association (EURIMA) for funding this work, and Chris Bolton (Institute of Occupational Medicine) for constructing the figures within this review. We also would like to thank the following people for providing valuable feedback on an early version of this review, and sharing their own experience with dissolution testing: Mette Solvang (ROCKWOOL International A/S), Christoph Koch (ROCKWOOL International A/S), Eric Pezennec (Knauf Insulation), Natasha Drnovsek (Knauf Insulation), and Nera Mascaraque (Ursa). This manuscript was also improved by insightful comments from the two reviewers selected by the Editor and anonymous to the authors. Their peer-review comments are gratefully acknowledged.
Declaration of interest
The authors report no conflicts of interest and have sole responsibility for the content and opinions of the paper. The author’s affiliations are as shown on the cover page. An early version of this manuscript was reviewed by industrial members of EURIMA, as indicated in the acknowledgments section, however we stress that the views and opinions expressed in this manuscript are solely that of the authors.
This work was financially supported by European Insulation Manufacturers Association (EURIMA), an association that represents the interest of mineral wool producers throughout Europe. The Institute of Occupational Medicine (IOM) – an independent occupational and environmental health research institute – were hired by EURIMA as external consultants with expertise in particle and fibre toxicology, and with the practical knowledge of the dissolution testing methods addressed in this review. The IOM was compensated by EURIMA for the preparation of this review. HHP Yiu (Heriot-Watt University) was asked to contribute to the writing of this paper by the authors at IOM and received no financial payment.
References
- Abollino O, Giacomino A, Paparella G, Magi E, Conca E, Malandrino M. 2018. Potentially toxic elements in ayurvedic formulations: total and bioaccessible content. Microchem J. 136:236–243.
- Adamcakova-Dodd A, Stebounova LV, Kim J, Vorrink SU, Ault AP, O’Shaughnessy PT, Grassian VH, Thorne PS. 2014. Toxicity assessment of zinc oxide nanoparticles using sub-acute and sub-chronic murine inhalation models. Part Fibre Toxicol. 11:15.
- Aladova EE, Romanov SA, Guilmette RA, Khokhryakov VF, Suslova KG. 2007. In vitro dissolution study of plutonium in aerosol particles from the Mayak PA: a tool for individualised dose estimates. Radiat Prot Dosimetry. 127:60–63.
- Alexander IC, Brown RC, Jubb GA, Pickering P, Hoskins JA. 1994. Durability of ceramic and novel man-made mineral fibers. Environ Health Perspect. 102:67–71.
- Allen BL, Kichambare PD, Gou P, Vlasova II, Kapralov AA, Konduru N, Kagan VE, Star A. 2008. Biodegradation of single-walled carbon nanotubes through enzymatic catalysis. Nano Lett. 8:3899–3903.
- Allen BL, Kotchey GP, Chen Y, Yanamala NVK, Klein-Seetharaman J, Kagan VE, Star A. 2009. Mechanistic investigations of horseradish peroxidase-catalyzed degradation of single-walled carbon nanotubes. J Am Chem Soc. 131:17194–17205.
- Alpofead JAH, Davidson CM, Littlejohn D. 2017. A novel two-step sequential bioaccessibility test for potentially toxic elements in inhaled particulate matter transported into the gastrointestinal tract by mucociliary clearance. Anal Bioanal Chem. 409:3165–3174.
- Andersen JC, Cropp A, Paradise DC. 2017. Solubility of indium-tin oxide in simulated lung and gastric fluids: pathways for human intake. Sci Total Environ. 579:628–636.
- Andón FT, Kapralov AA, Yanamala N, Feng W, Baygan A, Chambers BJ, Hultenby K, Ye F, Toprak MS, Brandner BD, et al. 2013. Biodegradation of single-walled carbon nanotubes by eosinophil peroxidase. Small. 9:2721–2729. 2720.
- Ansoborlo E, Chalabreysse J, Escallon S, Henge-Napoli MH. 1990. In vitro solubility of uranium tetrafluoride with oxidizing medium compared with in vivo solubility in rats. Int J Radiat Biol. 58:681–689.
- Ansoborlo E, Chalabreysse J, Henge-Napoli MH, Pujol E. 1992. In vitro chemical and cellular tests applied to uranium trioxide with different hydration states. Environ Health Perspect. 97:139–143.
- Ansoborlo E, Henge-Napoli MH, Chazel V, Gibert R, Guilmette RA. 1999. Review and critical analysis of available in vitro dissolution tests. Health Phys. 77:638–645.
- Artelt S, Creutzenberg O, Kock H, Levsen K, Nachtigall D, Heinrich U, Ruhle T, Schlogl R. 1999. Bioavailability of fine dispersed platinum as emitted from automotive catalytic converters: a model study. Sci Total Environ. 228:219–242.
- Artelt S, Kock H, Nachtigall D, Heinrich U. 1998. Bioavailability of platinum emitted from automobile exhaust. Toxicol Lett. 96-97:163–167.
- Baek M, Kim IS, Yu J, Chung HE, Choy JH, Choi SJ. 2011. Effect of different forms of anionic nanoclays on cytotoxicity. J Nanosci Nanotechnol. 11:1803–1806.
- Bal W, Sokołowska M, Kurowska E, Faller P. 2013. Binding of transition metal ions to albumin: sites, affinities and rates. Biochim Biophys Acta. 1830:5444–5455.
- Balakumaran MD, Ramachandran R, Jagadeeswari S, Kalaichelvan PT. 2016. In vitro biological properties and characterization of nanosilver coated cotton fabrics – An application for antimicrobial textile finishing. Int Biodeterior Biodegrad. 107:48–55.
- Bandyopadhyay D, Cyphersmith A, Zapata JA, Kim YJ, Payne CK. 2014. Lysosome transport as a function of lysosome diameter. PLoS One. 9:e86847.
- Barly SHQ, Okhrimenko DV, Solvang M, Yue Y, Stipp SLS. 2019. Dissolution of stone wool fibers with phenol-urea-formaldehyde binder in a synthetic lung fluid. Chem Res Toxicol. 32:2398–2410.
- Beeston MP, van Elteren JT, Selih VS, Fairhurst R. 2010. Characterization of artificially generated PbS aerosols and their use within a respiratory bioaccessibility test. Analyst. 135:351–357.
- Benjaminsen RV, Mattebjerg MA, Henriksen JR, Moghimi SM, Andresen TL. 2013. The Possible “Proton sponge" effect of polyethylenimine (PEI) does not include change in lysosomal pH”. Mol Ther. 21:149–157.
- Berlinger B, Ellingsen DG, Naray M, Zaray G, Thomassen Y. 2008. A study of the bio-accessibility of welding fumes. J Environ Monit. 10:1448–1453.
- Berlinger B, Skogen U, Meijer C, Thomassen Y. 2019. Workplace exposure to particulate matter, bio-accessible, and non-soluble metal compounds during hot work processes. J Occup Environ Hyg. 16:378–386.
- Berlinger B, Weinbruch S, Ellingsen DG, Zibarev E, Chashchin V, Chashchin M, Thomassen Y. 2019. On the bio-accessibility of 14 elements in welding fumes. Environ Sci Process Impacts. 21:497–505.
- Bernstein DM, Morscheidt C, Grimm HG, Thévenaz P, Teichert U. 1996. Evaluation of soluble fibers using the inhalation biopersistence model, a nine-fiber comparison. Inhalation Toxicol. 8:345–385.
- Bertoli F, Davies GL, Monopoli MP, Moloney M, Gun'ko YK, Salvati A, Dawson KA. 2014. Magnetic nanoparticles to recover cellular organelles and study the time resolved nanoparticle-cell interactome throughout uptake. Small. 10:3307–3315.
- Bicer EM. 2015. Compositional characterisation of human respiratory tract lining fluids for the design of disease specific simulants. London (UK): King’s College London.
- Bidani A, Brown SE, Heming TA, Gurich R, TD, Dubose J. 1989. Cytoplasmic pH in pulmonary macrophages: recovery from acid load is Na + independent and NEM sensitive. Am J Physiol. 257:C65–C76.
- Birmili W, Allen AG, Bary F, Harrison RM. 2006. Trace metal concentrations and water solubility in size-fractionated atmospheric particles and influence of road traffic. Environ Sci Technol. 40:1144–1153.
- Bodem CR, Lampton LM, Miller DP, Tarka EF, Everett ED. ED. 1983. Endobronchial pH. Am Rev Respir Dis. 127:39–41.
- Bohner M, Lemaitre J. 2009. Can bioactivity be tested in vitro with SBF solution? Biomaterials. 30:2175–2179.
- Boisa N, Elom N, Dean JR, Deary ME, Bird G, Entwistle JA. 2014. Development and application of an inhalation bioaccessibility method (IBM) for lead in the PM10 size fraction of soil. Environ Int. 70:132–142.
- Bourliva A, Papadopoulou L, da Silva EF, Patinha C. 2020. In vitro assessment of oral and respiratory bioaccesibility of trace elements of environmental concern in Greek fly ashes: assessing health risk via ingestion and inhalation. Sci Total Environ. 704:135324.
- Boyles MSP, Brown D, Knox J, Horobin M, Miller MR, Johnston HJ, Stone V. 2018. Assessing the bioactivity of crystalline silica in heated high-temperature insulation wools. Inhal Toxicol. 30:255–272.
- Boyles MSP, Poland CA, Raftis J, Duffin R. 2019. Assessment of the physicochemical properties of chrysotile-containing brake debris pertaining to toxicity. Inhal Toxicol. 31:325–342.
- Boyles MSP, Ranninger C, Reischl R, Rurik M, Tessadri R, Kohlbacher O, Duschl A, Huber CG. 2016. Copper oxide nanoparticle toxicity profiling using untargeted metabolomics. Part Fibre Toxicol. 13:49.
- Boyles MSP, Kristl T, Andosch A, Zimmermann M, Tran N, Casals E, Himly M, Puntes V, Huber CG, Lütz-Meindl U, et al. 2015a. Chitosan functionalisation of gold nanoparticles encourages particle uptake and induces cytotoxicity and pro-inflammatory conditions in phagocytic cells, as well as enhancing particle interactions with serum components. J Nanobiotechnology. 13:84.
- Boyles MSP, Young L, Brown DM, MacCalman L, Cowie H, Moisala A, Smail F, Smith PJW, Proudfoot L, Windle AH, et al. 2015b. Multi-walled carbon nanotube induced frustrated phagocytosis, cytotoxicity and pro-inflammatory conditions in macrophages are length dependent and greater than that of asbestos. Toxicol In Vitro. 29:1513–1528.
- Breitner EK, Burns KE, Hussain SM, Comfort KK. 2018. Implementation of physiological fluids to provide insight into the characterization, fate, and biological interactions of silver nanoparticles. Nanotechnology. 29:254001.
- Broadway A, Cave MR, Wragg J, Fordyce FM, Bewley RJ, Graham MC, Ngwenya BT, Farmer JG. 2010. Determination of the bioaccessibility of chromium in Glasgow soil and the implications for human health risk assessment. Sci Total Environ. 409:267–277.
- Brown AD, Barrett JES, Bennett M, Potgieter-Vermaak S. 2019. An investigation into the use of < 38 microm fraction as a proxy for < 10 microm road dust particles. Environ Geochem Health. 42:1117–1126.
- Brown RC, Harrison PTC. 2012. Alkaline earth silicate wools – a new generation of high temperature insulation. Regul Toxicol Pharmacol. 64:296–304.
- Buchman JT, Bennett EA, Wang C, Abbaspour Tamijani A, Bennett JW, Hudson BG, Green CM, Clement PL, Zhi B, Henke AH, et al. 2020. Nickel enrichment of next-generation NMC nanomaterials alters material stability, causing unexpected dissolution behavior and observed toxicity to S. oneidensis MR-1 and D. magna. Environ Sci Nano. 7:571–587.
- Cannizzaro A, Angelosanto F, Barrese E, Campopiano A. 2019. Biosolubility of high temperature insulation wools in simulated lung fluids. J Occup Med Toxicol. 14:15.
- Cantin AM, Fells GA, Hubbard RC, Crystal RG. 1990. Antioxidant macromolecules in the epithelial lining fluid of the normal human lower respiratory tract. J Clin Invest. 86:962–971.
- Cantin AM, North SL, Hubbard RC, Crystal RG. 1987. Normal alveolar epithelial lining fluid contains high levels of glutathione. J Appl Physiol. 63:152–157.
- Cathe DS, Whitaker JN, Breitner EK, Comfort KK. 2017. Exposure to metal oxide nanoparticles in physiological fluid induced synergistic biological effects in a keratinocyte model. Toxicol Lett. 268:1–7.
- Chakraborty S, Nair A, Paliwal M, Dybowska A, Misra SK. 2018. Exposure media a critical factor for controlling dissolution of CuO nanoparticles. J Nanopart Res. 20:331.
- Charrier JG, McFall AS, Richards-Henderson NK, Anastasio C. 2014. Hydrogen peroxide formation in a surrogate lung fluid by transition metals and quinones present in particulate matter. Environ Sci Technol. 48:7010–7017.
- Chazel V, Gerasimo P, Dabouis V, Laroche P, Paquet F. 2003. Characterisation and dissolution of depleted uranium aerosols produced during impacts of kinetic energy penetrators against a tank. Radiat Prot Dosim. 105:163–166.
- Chazel V, Houpert P, Paquet F, Ansoborlo E, Heng-Napoli MH. 2000. Experimental determination of the solubility of industrial UF4 particulates. Radiat Prot Dosim. 92:289–294.
- Cheng YS, Dahl AR, Jow HN. 1997. Dissolution of metal tritides in a simulated lung fluid. Health Phys. 73:633–638.
- Cheng YS, Guilmette RA, Zhou Y, Gao J, Labone T, Whicker JJ, Hoover MD. 2004. Characterization of plutonium aerosol collected during an accident. Health Phys. 87:596–605.
- Cheng YS, Zhou Y, Wang YS, Inkret WC, Wermer JR. 2002. Dose estimate of inhaled hafnium tritide using the ICRP 66 lung model. Health Phys. 82:817–824.
- Cheyne SE, McClintock LF, Blackman AG. 2006. Stabilization of coordinated carbonate in aqueous acidic solution: steric inhibition of protonation in Co(III) complexes containing chelated carbonate. Inorg Chem. 45:2610–2618.
- Christensen VR, Jensen SL, Guldberg M, Kamstrup O. 1994. Effect of chemical composition of man-made vitreous fibers on the rate of dissolution in vitro at different pHs. Environ Health Perspect. 102 Suppl 5:83–86.
- Coakley RD, Grubb BR, Paradiso AM, Gatzy JT, Johnson LG, Kreda SM, Neal WK, Boucher RC. 2003. Abnormal surface liquid pH regulation by cultured cystic fibrosis bronchial epithelium. Proc Natl Acad Sci U S A. 100:16083–16088.
- Collier CG, Pearce MJ, Hodgson A, Ball A. 1992. Factors affecting the in vitro dissolution of cobalt oxide. Environ Health Perspect. 97:109–113.
- Colombo C, Monhemius AJ, Plant JA. 2008. Platinum, palladium and rhodium release from vehicle exhaust catalysts and road dust exposed to simulated lung fluids. Ecotoxicol Environ Saf. 71:722–730.
- Cooper B, Creeth JM, Donald AS. 1985. Studies of the limited degradation of mucus glycoproteins. The mechanism of the peroxide reaction. Biochem J. 228:615–626.
- Costabile G, d'Angelo I, Rampioni G, Bondì R, Pompili B, Ascenzioni F, Mitidieri E, d'Emmanuele di Villa Bianca R, Sorrentino R, Miro A, et al. 2015. Toward repositioning niclosamide for antivirulence therapy of Pseudomonas aeruginosa lung infections: development of inhalable formulations through nanosuspension technology. Mol Pharm. 12:2604–2617.
- Coufalik P, Matousek T, Krumal K, Vojtisek-Lom M, Beranek V, Mikuska P. 2019. Content of metals in emissions from gasoline, diesel, and alternative mixed biofuels. Environ Sci Pollut Res. 26:29012–29019.
- Coufalik P, Mikuska P, Matousek T, Vecera Z. 2016. Determination of the bioaccessible fraction of metals in urban aerosol using simulated lung fluids. Atmos Environ. 140:469–475.
- Creuwels LAJM, van Golde LMG, Haagsman HP. 1997. The pulmonary surfactant system: biochemical and clinical aspects. Lung. 175:1–39.
- Cross CE, van der Vliet A, O'Neill CA, Louie S, Halliwell B. 1994. Oxidants, antioxidants, and respiratory tract lining fluids. Environ Health Perspect. 102:185–191.
- Cruz N, Rodrigues SM, Tavares D, Monteiro RJ, Carvalho L, Trindade T, Duarte AC, Pereira E, Romkens PF. 2015. Testing single extraction methods and in vitro tests to assess the geochemical reactivity and human bioaccessibility of silver in urban soils amended with silver nanoparticles. Chemosphere. 135:304–311.
- Cui XY, Xiang P, He RW, Juhasz A, Ma LQ. 2016. Advances in in vitro methods to evaluate oral bioaccessibility of PAHs and PBDEs in environmental matrices. Chemosphere. 150:378–389.
- da Silva LID, Yokoyama L, Maia LB, Monteiro MIC, Pontes FVM, Carneiro MC, Neto AA. 2015. Evaluation of bioaccessible heavy metal fractions in PM10 from the metropolitan region of Rio de Janeiro city, Brazil, using a simulated lung fluid. Microchem J. 118:266–271.
- Dargaville PA, South M, Vervaart P, McDougall PN. 1999. Validity of markers of dilution in small volume lung lavage. Am J Respir Crit Care Med. 160:778–784.
- Dartey E, Berlinger B, Thomassen Y, Ellingsen DG, Odland JO, Nartey VK, Yeboah FA, Weinbruch S. 2014. Bioaccessibility of lead in airborne particulates from car battery repair work. Environ Sci Process Impacts. 16:2782–2788.
- Davies NM, Feddah MR. 2003. A novel method for assessing dissolution of aerosol inhaler products. Int J Pharm. 255:175–187.
- Dean JR, Elom NI, Entwistle JA. 2017. Use of simulated epithelial lung fluid in assessing the human health risk of Pb in urban street dust. Sci Total Environ. 579:387–395.
- Deubner DC, Sabey P, Huang W, Fernandez D, Rudd A, Johnson WP, Storrs J, Larson R. 2011. Solubility and chemistry of materials encountered by beryllium mine and ore extraction workers: relation to risk. J Occup Environ Med. 53:1187–1193.
- Dörger M, Münzing S, Allmeling AM, Messmer K, Krombach F. 2001. Differential Responses of Rat Alveolar and Peritoneal Macrophages to Man-Made Vitreous Fibers in Vitro. Environ Res. 85:207–214.
- Duranti M, Scarafoni A, Di Cataldo A, Sessa F. 2001. Interaction of metal ions with lupin seed conglutin gamma. Phytochemistry. 56:529–533.
- EC. 2011. Commision Recommendation of 18 October 2011 on the definition of nanomaterial (Text with EEA relevance) (2011/696/EU). OJ, L 275:38–40.
- ECHA. 2016. New approach methodologies in regulatory science: proceedings of a scientific workshop. [accessed April 2020]. https://echa.europa.eu/documents/10162/22816069/scientific_ws_proceedings_en.pdf
- Eidson AF. 1994. The effect of solubility on inhaled uranium compound clearance: a review. Health Phys. 67:1–14.
- Eidson AF, Griffith WC. Jr. 1984. Techniques for yellowcake dissolution studies in vitro and their use in bioassay interpretation. Health Phys. 46:151–163.
- Eidson AF, Mewhinney JA. 1980. In vitro solubility of yellowcake samples from four uranium mills and the implications for bioassay interpretation. Health Phys. 39:893–902.
- Ellingsen DG, Chashchin M, Berlinger B, Fedorov V, Chashchin V, Thomassen Y. 2017. Biological monitoring of welders’ exposure to chromium, molybdenum, tungsten and vanadium. J Trace Elem Med Biol. 41:99–106.
- Ellingsen DG, Zibarev E, Kusraeva Z, Berlinger B, Chashchin M, Bast-Pettersen R, Chashchin V, Thomassen Y. 2013. The bioavailability of manganese in welders in relation to its solubility in welding fumes. Environ Sci Process Impacts. 15:357–365.
- Fashina A, Antunes E, Nyokong T. 2013. Silica nanoparticles grafted with phthalocyanines: photophysical properties and studies in artificial lysosomal fluid. New J Chem. 37:2800–2809.
- Figueroa-Lara JJ, Murcia-Gonzalez JM, Garcia-Martinez R, Romero-Romo M, Torres RM, Mugica AV. 2019. Effect of platform subway depth on the presence of Airborne PM2.5, metals, and toxic organic species. J Hazard Mater. 377:427–436.
- Foy JWD, Collier C, Swauger JE. 2003. A comparison of mathematical methods for the determination of in vitro dissolution constants for glass fibers. Inhal Toxicol. 15:167–179.
- Frohlich E. 2017. Toxicity of orally inhaled drug formulations at the alveolar barrier: parameters for initial biological screening. Drug Delivery. 24:891–905.
- Gamble JL. 1976. Chemical anatomy, physiology and pathology of extracellular fluid: a lecture syllabus. Cambridge (MA): Harvard University Press.
- Gao P, Guo H, Wang S, Guo L, Xing Y, Yao C, Jia L, Fan Q, Hang J. 2019. In Vitro investigations of high molecular weight polycyclic aromatic hydrocarbons in winter airborne particles using simulated lung fluids. Atmos Environ. 201:293–300.
- Gao P, Jian H, Xing Y, Tianxing X, Chen X, Jia L, Hang J. 2020. Bioaccessiblity and exposure assessment of PM2.5- and PM10-bound rare earth elements in Oil City, Northeast China. J Hazard Mater. 396:122520.
- Garger EK, Meisenberg O, Odintsov O, Shynkarenko V, Tschiersch J. 2013. Solubility of hot fuel particles from Chernobyl-influencing parameters for individual radiation dose calculations. Talanta. 115:40–46.
- Garger EK, Sazhenyuk AD, Odintzov AA, Paretzke HG, Roth P, Tschiersch J. 2004. Solubility of airborne radioactive fuel particles from the Chernobyl reactor and implication to dose. Radiat Environ Biophys. 43:43–49.
- Giordani M, Cametti G, Di Lorenzo F, Churakov SV. 2019. Real-time observation of fibrous zeolites reactivity in contact with simulated lung fluids (SLFs) obtained by atomic force microscope (AFM). Minerals. 9:83.
- Goerke J. 1998. Pulmonary surfactant: functions and molecular composition. Biochim Biophys Acta. 1408:79–89.
- Gomez MA, Alisaraie L, Shio MT, Berghuis AM, Lebrun C, Gautier-Luneau I, Olivier M. 2010. Protein tyrosine phosphatases are regulated by mononuclear iron dicitrate. J Biol Chem. 285:24620–24628.
- Gosselin M, Zagury GJ. 2020. Metal(loid)s inhalation bioaccessibility and oxidative potential of particulate matter from chromated copper arsenate (CCA)-contaminated soils. Chemosphere. 238:124557.
- Gray EP, Browning CL, Wang M, Gion KD, Chao EY, Koski KJ, Kane AB, Hurt RH. 2018. Biodissolution and cellular response to MoO3 nanoribbons and a new framework for early hazard screening for 2D materials. Environ Sci Nano. 5:2545–2559.
- Gray JE, Plumlee GS, Morman SA, Higueras PL, Crock JG, Lowers HA, Witten ML. 2010. In vitro studies evaluating leaching of mercury from mine waste calcine using simulated human body fluids. Environ Sci Technol. 44:4782–4788.
- Greenwell LL, Moreno T, Richards RJ. 2003. Pulmonary antioxidants exert differential protective effects against urban and industrial particulate matter. J Biosci. 28:101–107.
- Griffiths NM, Coudert S, Moureau A, Laroche P, Angulo JF, Van der Meeren A. 2016. Forecasting the in vivo behavior of radiocontaminants of unknown physicochemical properties using a simple in vitro test. Health Phys. 111:93–99.
- Gualtieri AF, Pollastri S, Bursi Gandolfi N, Gualtieri ML. 2018. In vitro acellular dissolution of mineral fibres: a comparative study. Sci Rep. 8:7071.
- Guldberg M, Christensen VR, Perander M, Zoitos B, Koenig AR, Sebastian K. 1998. Measurement of in-vitro fibre dissolution rate at acidic pH. Ann Occup Hyg. 42:233–243.
- Guldberg M, Jensen SL, Knudsen T, Steenberg T, Kamstrup O. 2002. High-alumina low-silica HT stone wool fibers: a chemical compositional range with high biosolubility. Regul Toxicol Pharmacol. 35:217–226.
- Guldberg M, Madsen AL, Sebastian K, Fellman J, Potter R, Bauer J, Searl A, Maquin B, Jubb G. 2003. In-vitro dissolution of vitreous silicate fibres according to EURIMA test guideline – results of two Round Robins. Glass Sci Technol. 76:199–205.
- Guldberg M, de Meringo A, Kamstrup O, Furtak H, Rossiter C. 2000. The development of glass and stone wool compositions with increased biosolubility. Regul Toxicol Pharmacol. 32:184–189.
- Guney M, Bourges CM, Chapuis RP, Zagury GJ. 2017. Lung bioaccessibility of As, Cu, Fe, Mn, Ni, Pb, and Zn in fine fraction (<20mum) from contaminated soils and mine tailings. Sci Total Environ. 579:378–386.
- Gupta V, Ahsan F. 2011. Influence of PEI as a core modifying agent on PLGA microspheres of PGE₁, a pulmonary selective vasodilator. Int J Pharm. 413:51–62.
- Hamilton RF, Wu Z, Thakkar M, Holian A, Mitra S. 2018. Modification of nano-silver bioactivity by adsorption on carbon nanotubes and graphene oxide. Inhal Toxicol. 30:429–438.
- Hampton MB, Kettle AJ, Winterbourn CC. 1998. Inside the neutrophil phagosome: oxidants, myeloperoxidase, and bacterial killing. Blood. 92:3007–3017.
- Harrington AD, Hylton S, Schoonen MA. 2012. Pyrite-driven reactive oxygen species formation in simulated lung fluid: implications for coal workers’ pneumoconiosis. Environ Geochem Health. 34:527–538.
- Harris WR, Silberman D. 1983. Time-dependent leaching of coal fly ash by chelating agents. Environ Sci Technol. 17:139–145.
- Hassoun M, Royall PG, Parry M, Harvey RD, Forbes B. 2018. Design and development of a biorelevant simulated human lung fluid. J Drug Deliv Sci Technol. 47:485–491.
- Hatch GE. 1992. Comparative biochemistry of airway lining fluid. In: Parent RA, editor. Treatise on Pulmonary Toxicology. Florida: CRC Press; Vol. 1. p. 617–632.
- Hedberg Y, Gustafsson J, Karlsson HL, Moller L, Wallinder IO. 2010. Bioaccessibility, bioavailability and toxicity of commercially relevant iron- and chromium-based particles: in vitro studies with an inhalation perspective. Part Fibre Toxicol. 7:23.
- Hedberg Y, Hedberg J, Liu Y, Wallinder IO. 2011. Complexation- and ligand-induced metal release from 316L particles: importance of particle size and crystallographic structure. Biometals. 24:1099–1114.
- Heffernan TE, Lodwick JC, Spitz H, Neton J, Soldano M. 2001. Solubility of airborne uranium compounds at the Fernald Environmental Management Project. Health Phys. 80:255–262.
- Heim KE, Danzeisen R, Verougstraete V, Gaidou F, Brouwers T, Oller AR. 2020. Bioaccessibility of nickel and cobalt in synthetic gastric and lung fluids and its potential use in alloy classification. Regul Toxicol Pharmacol. 110:104549.
- Heming TA, Bidani A. 1995. Effects of myristate phorbol ester on V-ATPase activity and Na+-H + exchange in alveolar macrophages. J Leukoc Biol. 57:600–608.
- Henderson RG, Verougstraete V, Anderson K, Arbildua JJ, Brock TO, Brouwers T, Cappellini D, Delbeke K, Herting G, Hixon G, et al. 2014. Inter-laboratory validation of bioaccessibility testing for metals. Regul Toxicol Pharmacol. 70:170–181.
- Hermans C, Aly O, Nyberg BI, Peterson C, Bernard A. 1998. Determinants of Clara cell protein (CC16) concentration in serum: a reassessment with two different immunoassays. Clin Chim Acta. 272:101–110.
- Hernandez-Pellon A, Nischkauer W, Limbeck A, Fernandez-Olmo I. 2018. Metal(loid) bioaccessibility and inhalation risk assessment: a comparison between an urban and an industrial area. Environ Res. 165:140–149.
- Herting G, Odnevall Wallinder I, Leygraf C. 2006. Factors that influence the release of metals from stainless steels exposed to physiological media. Corros Sci. 48:2120–2132.
- Herting G, Wallinder IO, Leygraf C. 2007. Metal release from various grades of stainless steel exposed to synthetic body fluids. Corros Sci. 49:103–111.
- Herting G, Wallinder IO, Leygraf C. 2008. Metal release rate from AISI 316L stainless steel and pure Fe, Cr and Ni into a synthetic biological medium-a comparison. J Environ Monit. 10:1092–1098.
- Hettiarachchi E, Paul S, Cadol D, Frey B, Rubasinghege G. 2019. Mineralogy controlled dissolution of uranium from airborne dust in simulated lung fluids (SLFs) and possible health implications. Environ Sci Technol Lett. 6:62–67.
- Holmes AM, Lim J, Studier H, Roberts MS. 2016. Varying the morphology of silver nanoparticles results in differential toxicity against micro-organisms, HaCaT keratinocytes and affects skin deposition. Nanotoxicology. 10:1503–1514.
- Holter JF, Weiland JE, Pacht ER, Gadek JE, Davis WB. 1986. Protein permeability in the adult respiratory distress syndrome. Loss of size selectivity of the alveolar epithelium. J Clin Invest. 78:1513–1522.
- Hu X, Zhang N, Liu YT. 2019. In vitro ingestion and inhalation bioaccessibility of soilborne lead, cadmium, arsenic and chromium near a chemical industrial park for health risk assessment. Env Pollut Bioavail. 31:316–322.
- Huang X, Betha R, Tan LY, Balasubramanian R. 2016. Risk assessment of bioaccessible trace elements in smoke haze aerosols versus urban aerosols using simulated lung fluids. Atmos Environ. 125:505–511.
- Huang W, Fernandez D, Rudd A, Johnson WP, Deubner D, Sabey P, Storrs J, Larsen R. 2011. Dissolution and nanoparticle generation behavior of Be-associated materials in synthetic lung fluid using inductively coupled plasma mass spectroscopy and flow field-flow fractionation. J Chromatogr A. 1218:4149–4159.
- Huang H, Jiang Y, Xu X, Cao X. 2018. In vitro bioaccessibility and health risk assessment of heavy metals in atmospheric particulate matters from three different functional areas of Shanghai, China. Sci Total Environ. 610–611:546–554.
- Huk A, Izak-Nau E, Reidy B, Boyles M, Duschl A, Lynch I, Dušinska M. 2014. Is the toxic potential of nanosilver dependent on its size? Part Fibre Toxicol 11:65.
- ICRP. 1994. Human respiratory tract model for radiological protection. ICRP Publication. 66:1–3.
- Inkret WC, Schillaci ME, Boyce MK, Cheng YS, Efurd DW, Little TT, Miller G, Musgrave JA, Wermer JR. 2001. Internal dosimetry for inhalation of hafnium tritide aerosols. Radiat Prot Dosimetry. 93:55–60.
- ISO. 2017. ISO/TR 19057 Nanotechnologies – use and application of acellular in vitro tests and methodologies to assess nanomaterial biodurability.
- Jang Y, Owuor D, Waterman JT, White L, Collins B, Sankar J, Gilbert TW, Yun Y. 2014. Effect of mucin and bicarbonate ion on corrosion behavior of AZ31 magnesium alloy for airway stents. Materials. 7:5866–5882.
- Jayaraman S, Song Y, Verkman AS. 2001a. Airway surface liquid pH in well-differentiated airway epithelial cell cultures and mouse trachea. Am J Physiol Cell Physiol. 281:C1504–C1511.
- Jayaraman S, Song Y, Vetrivel L, Shankar L, Verkman AS. 2001b. Noninvasive in vivo fluorescence measurement of airway-surface liquid depth, salt concentration, and pH. J Clin Invest. 107:317–324.
- Johansson A, Lundborg M, Skold CM, Lundahl J, Tornling G, Eklund A, Camner P. 1997. Functional, morphological, and phenotypical differences between rat alveolar and interstitial macrophages. Am J Respir Cell Mol Biol. 16:582–588.
- Johnson NF. 1994. Phagosomal pH and glass fiber dissolution in cultured nasal epithelial cells and alveolar macrophages: a preliminary study. Environ Health Perspect. 102 Suppl 5:97–102.
- Joris L, Dab I, Quinton PM. 1993. Elemental composition of human airway surface fluid in healthy and diseased airways. Am Rev Respir Dis. 148:1633–1637.
- Jurinski JB, Rimstidt JD. 2001. Biodurability of talc. Am Mineral. 86:392–399.
- Kagan VE, Konduru NV, Feng W, Allen BL, Conroy J, Volkov Y, Vlasova II, Belikova NA, Yanamala N, Kapralov A, et al. 2010. Carbon nanotubes degraded by neutrophil myeloperoxidase induce less pulmonary inflammation. Nat Nanotechnol. 5:354–359.
- Kahn MC, Anderson GJ, Anyan WR, Hall SB. 1995. Phosphatidylcholine molecular species of calf lung surfactant. Am J Physiol. 269:L567–L573.
- Kalina M, Blau H, Riklis S, Kravtsov V. 1995. Interaction of surfactant protein A with bacterial lipopolysaccharide may affect some biological functions. Am J Physiol. 268:L144–L151.
- Kanapilly GM. 1977. Alveolar microenvironment and its relationship to the retention and transport into blood of aerosols deposited in the alveoli. Health Phys. 32:89–100.
- Karote D, Walker B, Dai HE, Krishnamoorthi R, Voo J, Rajagopalan S. 2013. Chemical warfare agent simulants in Gamble’s fluid: is the fluid toxic? Can it be made safer by inclusion of solid nanocrystalline metal oxides? J Chem. 2013:641620.
- Kastury F, Ritch S, Rasmussen PE, Juhasz AL. 2020. Influence of household smoking habits on inhalation bioaccessibility of trace elements and light rare earth elements in Canadian house dust. Environ Pollut. 262:114132.
- Kastury F, Smith E, Karna RR, Scheckel KG, Juhasz AL. 2018a. An inhalation-ingestion bioaccessibility assay (IIBA) for the assessment of exposure to metal(loid)s in PM10. Sci Total Environ. 631-632:92–104.
- Kastury F, Smith E, Karna RR, Scheckel KG, Juhasz AL. 2018b. Methodological factors influencing inhalation bioaccessibility of metal(loid)s in PM2.5 using simulated lung fluid. Environ Pollut. 241:930–937.
- Keller JG, Peijnenburg W, Werle K, Landsiedel R, Wohlleben W. 2020. Understanding dissolution rates via continuous flow systems with physiologically relevant metal ion saturation in lysosome. Nanomaterials. 10:311.
- Kelly FJ, Mudway IS. 2003. Protein oxidation at the air-lung interface. Amino Acids. 25:375–396.
- Kim S, Shim JJ, Burgel PR, Ueki IF, Dao-Pick T, Tam DCW, Nadel JA. 2002. IL-13-induced Clara cell secretory protein expression in airway epithelium: role of EGFR signaling pathway. Am J Physiol Lung Cell Mol Physiol. 283:L67–L75.
- Kirkham P, Rahman I. 2006. Oxidative stress in asthma and COPD: antioxidants as a therapeutic strategy. Pharmacol Ther. 111:476–494.
- Knowles MR, Robinson JM, Wood RE, Pue CA, Mentz WM, Wager GC, Gatzy JT, Boucher RC. 1997. Ion composition of airway surface liquid of patients with cystic fibrosis as compared with normal and disease-control subjects. J Clin Invest. 100:2588–2595.
- Kokubo T, Ito S, Shigematsu M, Sanka S, Yamamuro T. 1987. Fatigue and life-time of bioactive glass-ceramic A-W containing apatite and wollastonite. J Mater Sci. 22:4067–4070.
- Kokubo T, Takadama H. 2006. How useful is SBF in predicting in vivo bone bioactivity? Biomaterials. 27:2907–2915.
- Koltermann-Jülly J, Keller JG, Vennemann A, Werle K, Müller P, Ma-Hock L, Landsiedel R, Wiemann M, Wohlleben W. 2018. Abiotic dissolution rates of 24 (nano)forms of 6 substances compared to macrophage-assisted dissolution and in vivo pulmonary clearance: grouping by biodissolution and transformation. NanoImpact. 12:29–41.
- Kotchey GP, Zhao Y, Kagan VE, Star A. 2013. Peroxidase-mediated biodegradation of carbon nanotubes in vitro and in vivo. Adv Drug Deliv Rev. 65:1921–1932.
- Krawic C, Luczak MW, Zhitkovich A. 2017. Variation in extracellular detoxification is a link to different carcinogenicity among chromates in rodent and human lungs. Chem Res Toxicol. 30:1720–1729.
- Kreyling WG. 1992. Intracellular particle dissolution in alveolar macrophages. Environ Health Perspect. 97:121–126.
- Kuang XM, Scott JA, da Rocha GO, Betha R, Price DJ, Russell LM, Cocker DR, Paulson SE. 2017. Hydroxyl radical formation and soluble trace metal content in particulate matter from renewable diesel and ultra low sulfur diesel in at-sea operations of a research vessel. Aerosol Sci Technol. 51:147–158.
- Kumar A, Bicer EM, Morgan AB, Pfeffer PE, Monopoli M, Dawson KA, Eriksson J, Edwards K, Lynham S, Arno M, et al. 2016. Enrichment of immunoregulatory proteins in the biomolecular corona of nanoparticles within human respiratory tract lining fluid. Nanomedicine. 12:1033–1043.
- Kumar A, Terakosolphan W, Hassoun M, Vandera KK, Novicky A, Harvey R, Royall PG, Bicer EM, Eriksson J, Edwards K, et al. 2017. A biocompatible synthetic lung fluid based on human respiratory tract lining fluid composition. Pharm Res. 34:2454–2465.
- Lammel G, Kitanovski Z, Kukucka P, Novak J, Arangio AM, Codling GP, Filippi A, Hovorka J, Kuta J, Leoni C, et al. 2020. Oxygenated and nitrated polycyclic aromatic hydrocarbons in ambient air-levels, phase partitioning, mass size distributions, and inhalation bioaccessibility. Environ Sci Technol. 54:2615–2625.
- Lang CJ, Postle AD, Orgeig S, Possmayer F, Bernhard W, Panda AK, Jürgens KD, Milsom WK, Nag K, Daniels CB. 2005. Dipalmitoylphosphatidylcholine is not the major surfactant phospholipid species in all mammals. Am J Physiol Regul Integr Comp Physiol. 289:R1426–R1439.
- Latvala S, Hedberg J, Di Bucchianico S, Moller L, Odnevall Wallinder I, Elihn K, Karlsson HL. 2016. Nickel release, ROS generation and toxicity of Ni and NiO micro- and nanoparticles. PLoS One. 11:e0159684.
- Lecuyer T, Durand MA, Volatron J, Desmau M, Lai-Kuen R, Corvis Y, Seguin J, Wang G, Alloyeau D, Scherman D, et al. 2020. Degradation of ZnGa2O4:Cr3+ luminescent nanoparticles in lysosomal-like medium. Nanoscale. 12:1967–1974.
- Lee MC, Penland CM, Widdicombe JH, Wine JJ. 1998. Evidence that Calu-3 human airway cells secrete bicarbonate. Am J Physiol. 274:L450–L453.
- Lehuede P, deMeringo A, Bernstein DM. 1997. Comparison of the chemical evolution of MMVF following inhalation exposure in rats and acellular in vitro dissolution. Inhalation Toxicol. 9:495–523.
- Leiby KL, Raredon MSB, Niklason LE. 2020. Bioengineering the blood-gas barrier. Compr Physiol. 10:415–452.
- Leo BF, Chen S, Kyo Y, Herpoldt KL, Terrill NJ, Dunlop IE, McPhail DS, Shaffer MS, Schwander S, Gow A, et al. 2013. The stability of silver nanoparticles in a model of pulmonary surfactant. Environ Sci Technol. 47:11232–11240.
- Lesniak A, Campbell A, Monopoli MP, Lynch I, Salvati A, Dawson KA. 2010. Serum heat inactivation affects protein corona composition and nanoparticle uptake. Biomaterials. 31–36:9511–9518.
- Li X, Gao Y, Zhang M, Zhang Y, Zhou M, Peng L, He A, Zhang X, Yan X, Wang Y, et al. 2020. In vitro lung and gastrointestinal bioaccessibility of potentially toxic metals in Pb-contaminated alkaline urban soil: the role of particle size fractions. Ecotoxicol Environ Saf. 190:110151.
- Li Y, Juhasz AL, Ma LQ, Cui X. 2019. Inhalation bioaccessibility of PAHs in PM2.5: implications for risk assessment and toxicity prediction. Sci Total Environ. 650:56–64.
- Liu X, Hurt RH, Kane AB. 2010. Biodurability of single-walled carbon nanotubes depends on surface functionalization. Carbon. 48:1961–1969.
- Liu XL, Ji R, Shi Y, Wang F, Chen W. 2019. Release of polycyclic aromatic hydrocarbons from biochar fine particles in simulated lung fluids: implications for bioavailability and risks of airborne aromatics. Sci Total Environ. 655:1159–1168.
- Liu Y, Qi Y, Yin C, Wang S, Zhang S, Xu A, Chen W, Liu S. 2018. Bio-transformation of graphene oxide in lung fluids significantly enhances its photothermal efficacy. Nanotheranostics. 2:222–232.
- Lund LG, Aust AE. 1992. Iron mobilization from crocidolite asbestos greatly enhances crocidolite-dependent formation of DNA single-strand breaks in phi X174 RFI DNA. Carcinogenesis. 13:637–642.
- Lundborg M, Lind B, Camner P. 1984. Ability of rabbit alveolar macrophages to dissolve metals. Exp Lung Res. 7:11–22.
- Luo X, Zhao Z, Xie J, Luo J, Chen Y, Li H, Jin L. 2019. Pulmonary bioaccessibility of trace metals in PM2.5 from different megacities simulated by lung fluid extraction and DGT method. Chemosphere. 218:915–921.
- Luoto K, Holopainen M, Karppinen K, Perander M, Savolainen K. 1994. Dissolution of man-made vitreous fibers in rat alveolar macrophage culture and Gamble’s saline solution: influence of different media and chemical composition of the fibers. Environ Health Perspect. 102 Suppl 5:103–107.
- MacCuspie RI, Allen AJ, Hackley VA. 2011. Dispersion stabilization of silver nanoparticles in synthetic lung fluid studied under in situ conditions. Nanotoxicology. 5:140–156.
- Marques MRC, Loebenberg R, Almukainzi M. 2011. Simulated biological fluids with possible application in dissolution testing. Dissolution Technol. 18:15–28.
- Menon JU, Ravikumar P, Pise A, Gyawali D, Hsia CC, Nguyen KT. 2014. Polymeric nanoparticles for pulmonary protein and DNA delivery. Acta Biomater. 10:2643–2652.
- Metzger R, Cole L. 2004. Solubility characterization of airborne uranium from a uranium recycling plant. Health Phys. 87:89–91.
- Metzger R, Wichers D, Vaselin J, Velasquez P. 1997. Solubility characterization of airborne uranium from an in situ uranium processing plant. Health Phys. 72:418–422.
- Meza-Figueroa D, Barboza-Flores M, Romero FM, Acosta-Elias M, Hernández-Mendiola E, Maldonado-Escalante F, Pérez-Segura E, González-Grijalva B, Meza-Montenegro M, García-Rico L, et al. 2020. Metal bioaccessibility, particle size distribution and polydispersity of playground dust in synthetic lysosomal fluids. Sci Total Environ. 713:136481.
- Midander K, Pan J, Wallinder IO, Leygraf C. 2007. Metal release from stainless steel particles in vitro-influence of particle size. J Environ Monit. 9:74–81.
- MilosevicAc A, Bourquin J, Burnand D, Lemal P, Crippa F, Monnier CA, Rodriguez-Lorenzo L, Petri-Fink A, Rothen-Rutishauser B. 2019. Artificial lysosomal platform to study nanoparticle long-term stability. Chimia. 73:55–58.
- Monopoli MP, Walczyk D, Campbell A, Elia G, Lynch I, Baldelli BF, Dawson KA. 2011. Physical-chemical aspects of protein corona: relevance to in vitro and in vivo biological impacts of nanoparticles. J Am Chem Soc. 133:2525–2534.
- Morgensen G. 1984. Durability of mineral fibers in various buffer solutions. Riv Stn Sper Vetro. 14:135.
- Moss O. 1979. Simulants of lung interstitial fluid. Health Phys. 36:447–448.
- Mukhtar A, Limbeck A. 2013. Recent developments in assessment of bio-accessible trace metal fractions in airborne particulate matter: a review. Anal Chim Acta. 774:11–25.
- Mukhtar A, Mohr V, Limbeck A. 2015. The suitability of extraction solutions to assess bioaccessible trace metal fractions in airborne particulate matter: a comparison of common leaching agents. Environ Sci Pollut Res Int. 22:16620–16630.
- Mulla JAS, Mabrouk M, Choonara YE, Kumar P, Chejara DR, Du Toit LC, Pillay V. 2017. Development of respirable rifampicin-loaded nano-lipomer composites by microemulsion-spray drying for pulmonary delivery. J Drug Delivery Sci Technol. 41:13–19.
- Muller KH, Kulkarni J, Motskin M, Goode A, Winship P, Skepper JN, Ryan MP, Porter AE. 2010. pH-dependent toxicity of high aspect ratio ZnO nanowires in macrophages due to intracellular dissolution. ACS Nano. 4:6767–6779.
- Nel A, Xia T, Mädler L, Li N. 2006. Toxic potential of materials at the nanolevel. Science. 311:622–627.
- Ng AW, Bidani A, Heming TA. 2004. Innate host defense of the lung: effects of lung-lining fluid pH. Lung. 182:297–317.
- Nielson DW, Goerke J, Clements JA. 1981. Alveolar subphase pH in the lungs of anesthetized rabbits. Proc Natl Acad Sci U S A. 78:7119–7123.
- Nyberg K, Johansson A, Camner P. 1989a. Intraphagosomal pH in alveolar macrophages studied with fluorescein-labeled amorphous silica particles. Exp Lung Res. 15:49–62.
- Nyberg K, Johansson U, Rundquist I, Camner P. 1989b. Estimation of pH in individual alveolar macrophage phagolysosomes. Exp Lung Res. 15:499–510.
- Oberdörster G. 1993. Lung dosimetry: pulmonary clearance of inhaled particles. Aerosol Sci Technol. 18:279–289.
- O'Brien P. 2000. Peroxidases. Chem Biol Interact. 129:113–139.
- OECD. 2018. Assessment of biodurability of nanomaterials and their surface ligands (ENV/JM/MONO(2018)11). Series on the Safety of Manufactured Nanomaterials No. 86. Paris.
- OECD. 2020. Guidance document for the testing of dissolution and dispersion stability of nanomaterials and the use of the data for further environmental testing and assessment strategies (ENV/JM/MONO(2020)9). Series of Testing and Assessment No. 318. Paris.
- Osmond-McLeod MJ, Poland CA, Murphy F, Waddington L, Morris H, Hawkins SC, Clark S, Aitken R, McCall MJ, Donaldson K. 2011. Durability and inflammogenic impact of carbon nanotubes compared with asbestos fibres. Part Fibre Toxicol. 8:15.
- Oyane A, Kim HM, Furuya T, Kokubo T, Miyazaki T, Nakamura T. 2003. Preparation and assessment of revised simulated body fluids. J Biomed Mater Res A. 65:188–195.
- Pelfrene A, Cave MR, Wragg J, Douay F. 2017. In vitro investigations of human bioaccessibility from reference materials using simulated lung fluids. IJERPH. 14:112.
- Pellosi DS, d'Angelo I, Maiolino S, Mitidieri E, Bianca REdV, Sorrentino R, Quaglia F, Ungaro F. 2018. In vitro/in vivo investigation on the potential of Pluronic® mixed micelles for pulmonary drug delivery. Eur J Pharm Biopharm. 130:30–38.
- Pilcer G, Amighi K. 2010. Formulation strategy and use of excipients in pulmonary drug delivery. Int J Pharm. 392:1–19.
- Plumlee GS, Morman SA, Ziegler TL. 2006. The toxicological geochemistry of earth materials: an overview of processes and the interdisciplinary methods used to understand them. Rev Mineral Geochem. 64:5–57.
- Plumlee GS, Ziegler TL. 2003. The medical geochemistry of dusts, soils, and other earth materials. In: Holland HD, Turekian KK, editors. Environmental geochemistry. Amsterdam: Elsevier; Vol. 9. p. 263–310.
- Polezer G, Oliveira A, Potgieter-Vermaak S, Godoi AFL, de Souza RAF, Yamamoto CI, Andreoli RV, Medeiros AS, Machado CMD, Dos Santos EO, et al. 2019. The influence that different urban development models has on PM2.5 elemental and bioaccessible profiles. Sci Rep. 9:14846.
- Qi Y, Liu Y, Xia T, Xu A, Liu S, Chen W. 2018. The biotransformation of graphene oxide in lung fluids significantly alters its inherent properties and bioactivities toward immune cells. NPG Asia Mater. 10:385–396.
- Radivojev S, Zellnitz S, Paudel A, Fröhlich E. 2019. Searching for physiologically relevant in vitro dissolution techniques for orally inhaled drugs. Int J Pharm. 556:45–56.
- Raesch SS, Tenzer S, Storck W, Rurainski A, Selzer D, Ruge CA, Perez-Gil J, Schaefer UF, Lehr CM. 2015. Proteomic and lipidomic analysis of nanoparticle corona upon contact with lung surfactant reveals differences in protein, but not lipid composition. ACS Nano. 9:11872–11885.
- Ramos ME, Cappelli C, Rozalen M, Fiore S, Huertas F. 2011. Effect of lactate, glycine, and citrate on the kinetics of montmorillonite dissolution. Am Mineral. 96:768–780.
- Reif RH. 1994. Evaluation of in vitro dissolution rates of thorium in uranium mill tailings. Health Phys. 67:545–547.
- Rennard SI, Ghafouri M, Thompson AB, Under J, Vaughan W, Jones K, Ertl RF, Christensen K, Prince A, Stahl MG, et al. 1990. Fractional processing of sequential bronchoalveolar lavage to separate bronchial and alveolar samples. Am Rev Respir Dis. 141:208–217.
- Rozalen M, Ramos ME, Huertas FJ, Fiore S, Gervilla F. 2013. Dissolution kinetics and biodurability of tremolite particles in mimicked lung fluids: Effect of citrate and oxalate. J Asian Earth Sci. 77:318–326.
- Schmaljohann D. 2006. Thermo- and pH-responsive polymers in drug delivery. Adv Drug Deliv Rev. 58:1655–1670.
- Searl A, Cullen RT. 1997. An enzymatic tissue digestion method for fibre biopersistence studies. Ann Occup Hyg. 41:721–723.
- Sebastian K, Fellman J, Potter R, Bauer J, Manville J, Searl A, Meringo A, Maquin B, de Reydellet A, Jubb G, et al. 2002. EURIMA test guideline: In-vitro acellular dissolution of man-made vitreous silicate fibres. Glass Sci Technol. 75:263–270.
- Semisch A, Ohle J, Witt B, Hartwig A. 2014. Cytotoxicity and genotoxicity of nano - and microparticulate copper oxide: role of solubility and intracellular bioavailability. Part Fibre Toxicol. 11:10.
- Serrano AG, Pérez-Gil J. 2006. Protein-lipid interactions and surface activity in the pulmonary surfactant system. Chem Phys Lipids. 141:105–118.
- Shinohara N, Zhang G, Oshima Y, Kobayashi T, Imatanaka N, Nakai M, Sasaki T, Kawaguchi K, Gamo M. 2017. Kinetics and dissolution of intratracheally administered nickel oxide nanomaterials in rats. Part Fibre Toxicol. 14:48.
- Shvedova AA, Kapralov AA, Feng WH, Kisin ER, Murray AR, Mercer RR, St Croix CM, Lang MA, Watkins SC, Konduru NV, et al. 2012. Impaired clearance and enhanced pulmonary inflammatory/fibrotic response to carbon nanotubes in myeloperoxidase-deficient mice. PLoS One. 7:e30923.
- Sigg L, Lindauer U. 2015. Silver nanoparticle dissolution in the presence of ligands and of hydrogen peroxide. Environ Pollut. 206:582–587.
- Slade R, Crissman K, Norwood J, Hatch G. 1993. Comparison of antioxidant substances in bronchoalveolar lavage cells and fluid from humans, guinea pigs, and rats. Exp Lung Res. 19:469–484.
- Sleigh MA, Blake JR, Liron N. 1988. The propulsion of mucus by cilia. Am Rev Respir Dis. 137:726–741.
- Son YJ, McConville JT. 2009. Development of a standardized dissolution test method for inhaled pharmaceutical formulations. Int J Pharm. 382:15–22.
- Sosnowski TR. 2018. Particles on the lung surface – physicochemical and hydrodynamic effects. Curr Opin Colloid Interface Sci. 36:1–9.
- Spitler G, Spitz H, Glasser S, Hoffman MK, Bowen J. 2015. In vitro dissolution of uranium-contaminated soil in simulated lung fluid containing a pulmonary surfactant. Health Phys. 108:336–343.
- Stebounova LV, Gonzalez-Pech NI, Peters TM, Grassian VH. 2018. Physicochemical properties of air discharge-generated manganese oxide nanoparticles: Comparison to welding fumes. Environ Sci Nano. 2018:696–707.
- Steenberg T, Hjenner HK, Jensen SL, Guldberg M, Knudsen T. 2001. Dissolution behaviour of biosoluble HT stone wool fibres. Glass Sci Technol. 74:97–105.
- Stefaniak AB. 2010. Persistence of tungsten oxide particle/fiber mixtures in artificial human lung fluids. Part Fibre Toxicol. 7:38.
- Stefaniak AB, Guilmette RA, Day GA, Hoover MD, Breysse PN, Scripsick RC. 2005. Characterization of phagolysosomal simulant fluid for study of beryllium aerosol particle dissolution. Toxicol In Vitro. 19:123–134.
- Stefaniak AB, Harvey CJ. 2006. Dissolution of materials in artificial skin surface film liquids. Toxicol In Vitro. 20:1265–1283.
- Stefaniak AB, Harvey CJ, Bukowski VC, Leonard SS. 2010. Comparison of free radical generation by pre- and post-sintered cemented carbide particles. J Occup Environ Hyg. 7:23–34.
- Stefaniak AB, Leonard SS, Hoover MD, Virji MA, Day GA. 2009. Dissolution and reactive oxygen species generation of inhaled cemented tungsten carbide particles in artificial human lung fluids. J Phys Conf Ser. 151:012045.
- Stefaniak AB, Seehra MS, Fix NR, Leonard SS. 2014. Lung biodurability and free radical production of cellulose nanomaterials. Inhalation Toxicol. 26:733–749.
- Stefaniak AB, Virji MA, Day GA. 2011. Dissolution of beryllium in artificial lung alveolar macrophage phagolysosomal fluid. Chemosphere. 83:1181–1187.
- Stockley RA. 1984. Measurement of soluble proteins in lung secretions. Thorax. 39:241–247.
- Stopford W, Turner J, Cappellini D, Brock T. 2003. Bioaccessibility testing of cobalt compounds. J Environ Monit. 5:675–680.
- Strupp C. 2011. Beryllium metal I. experimental results on acute oral toxicity, local skin and eye effects, and genotoxicity. Ann Occup Hyg. 55:30–42.
- Suetake M, Nakano Y, Furuki G, Ikehara R, Komiya T, Kurihara E, Morooka K, Yamasaki S, Ohnuki T, Horie K, et al. 2019. Dissolution of radioactive, cesium-rich microparticles released from the Fukushima Daiichi Nuclear Power Plant in simulated lung fluid, pure-water, and seawater. Chemosphere. 233:633–644.
- Sutinen S, Riska H, Backman R, Sutinen SH, Fröseth B. 1995. Alveolar lavage fluid (ALF) of normal volunteer subjects: cytologic, immunocytochemical, and biochemical reference values. Respir Med. 89:85–92.
- Sysalova J, Szakova J, Tremlova J, Kasparovska K, Kotlik B, Tlustos P, Svoboda P. 2014. Methodological aspects of in vitro assessment of bio-accessible risk element pool in urban particulate matter. Biol Trace Elem Res. 161:216–222.
- Tam A, Wadsworth S, Dorscheid D, Man SFP, Sin DD. 2011. The airway epithelium: more than just a structural barrier. Ther Adv Respir Dis. 5:255–273.
- Tan Z, Bai Q, Yin Y, Zhang Y, Chen Q, Moon MH, Liu J. 2020. On-line determination of soluble Zn content and size of the residual fraction in PM2.5 incubated in various aqueous media. Sci Total Environ. 724:138309.
- Tappel AL. 1969. Lysosomal enzymes and other components. In: Dingle JT, Fell HB, editors. Lysosomal in biology and pathology. Amsterdam: Wiley. p. 1.
- Thelohan S, de Meringo A. 1994. In vitro dynamic solubility test: influence of various parameters. Environ Health Perspect. 102 :91–96.
- Thompson AB, Bohling T, Payvandi F, Rennard SI. 1990. Lower respiratory tract lactoferrin and lysozyme arise primarily in the airways and are elevated in association with chronic bronchitis. J Lab Clin Med. 115:148–158.
- Ungaro F, d'Emmanuele di Villa Bianca R, Giovino C, Miro A, Sorrentino R, Quaglia F, La Rotonda MI. 2009. Insulin-loaded PLGA/cyclodextrin large porous particles with improved aerosolization properties: in vivo deposition and hypoglycaemic activity after delivery to rat lungs. J Control Release. 135:25–34.
- Utembe W, Potgieter K, Stefaniak AB, Gulumian M. 2015. Dissolution and biodurability: important parameters needed for risk assessment of nanomaterials. Part Fibre Toxicol. 12
- van der Vliet A, O’Neill CA, Cross CE, Koostra JM, Volz WG, Halliwell B, Louie S. 1999. Determination of low-molecular-mass antioxidant concentrations in human respiratory tract lining fluids. Am J Physiol. 276:L289–296.
- Van Iwaarden JF, Pikaar JC, Storm J, Brouwer E, Verhoef J, Oosting RS, van Golde LMG, van Strijp JAG. 1994. Binding of surfactant protein A to the lipid A moiety of bacterial lipopolysaccharides. Biochem J. 303:407–411.
- Van Iwaarden JF, Shimizu H, Van Golde PHM, Voelker DR, Van Golde LMG. 1992. Rat surfactant protein D enhances the production of oxygen radicals by rat alveolar macrophages. Biochem J. 286:5–8.
- Vidrio E, Phuah CH, Dillner AM, Anastasio C. 2009. Generation of hydroxyl radicals from ambient fine particles in a surrogate lung fluid solution. Environ Sci Technol. 43:922–927.
- Vigliaturo R, Della Ventura G, Choi J, Marengo A, Lucci F, O'Shea M, Pérez-Rodríguez I, Gieré R. 2018. Mineralogical characterization and dissolution experiments in Gamble’s solution of tremolitic amphibole from Passo di Caldenno (Sondrio, Italy). Minerals. 8:557.
- Vitarella D, Moss O, Dorman DC. 2000. Pulmonary clearance of manganese phosphate, manganese sulfate, and manganese tetraoxide by CD rats following intratracheal instillation. Inhal Toxicol. 12:941–957.
- Warheit DB, Hartsky MA, Reed KL, Webb TR. 2001. Biodegradability of inhaled para-aramid respirable-sized fiber-shaped particulates: mechanistic in vivo and in vitro studies. Toxicol Appl Pharmacol. 174:78–88.
- Warheit DB, Hartsky MA, Webb TR. 2000. Biodegradability of inhaled p-aramid respirable fibre-shaped particulates: Representative of other synthetic organic fibre-types? Int Arch Occup Environ Health. 73:S75–S78.
- Webber SE, Widdicombe JG. 1989. The transport of albumin across the ferret in vitro whole trachea. J Physiol. 408:457–472.
- WHO. 1997. Determination of airborne fibre number concentrations; a recommended method by phase-contrast microscopy (membrane filter method).
- Widdicombe JH, Widdicombe JG. 1995. Regulation of human airway surface liquid. Respir Physiol. 99:3–12.
- Wiseman CLS, Niu J, Levesque C, Chenier M, Rasmussen PE. 2018. An assessment of the inhalation bioaccessibility of platinum group elements in road dust using a simulated lung fluid. Environ Pollut. 241:1009–1017.
- Wiseman CLS, Zereini F. 2014. Characterizing metal(loid) solubility in airborne PM10, PM2.5 and PM1 in Frankfurt, Germany using simulated lung fluids. Atmos Environ. 89:282–289.
- Witt EC, Shi HL, Wronkiewicz DJ, Pavlowsky RT. 2014. Phase partitioning and bioaccessibility of Pb in suspended dust from unsurfaced roads in Missouri-A potential tool for determining mitigation response. Atmos Environ. 88:90–98.
- Wohlleben W, Waindok H, Daumann B, Werle K, Drum M, Egenolf H. 2017. Composition, respirable fraction and dissolution rate of 24 stone wool MMVF with their binder. Part Fibre Toxicol. 14:29.
- Woods A, Andrian T, Sharp G, Bicer EM, Vandera KA, Patel A, Mudway I, Dailey LA, Forbes B. 2020. Development of new in vitro models of lung protease activity for investigating stability of inhaled biological therapies and drug delivery systems. Eur J Pharm Biopharm. 146:64–72.
- Wragg J, Klinck B. 2007. The bioaccessibility of lead from Welsh mine waste using a respiratory uptake test. J Environ Sci Health A. 42:1223–1231.
- Wragg J, Cave M, Basta N, Brandon E, Casteel S, Denys S, Gron C, Oomen A, Reimer K, Tack K, et al. 2011. An inter-laboratory trial of the unified BARGE bioaccessibility method for arsenic, cadmium and lead in soil. Sci Total Environ. 409:4016–4030.
- Wu J, Kobayashi M, Sousa EA, Liu W, Cai J, Goldman SJ, Dorner AJ, Projan SJ, Kavuru MS, Qiu Y, et al. 2005. Differential proteomic analysis of bronchoalveolar lavage fluid in asthmatics following segmental antigen challenge. Mol Cell Proteomics. 4:1251–1264.
- Xie JJ, Yuan CG, Shen YW, Xie J, He KQ, Zhu HT, Zhang KG. 2019a. Bioavailability/speciation of arsenic in atmospheric PM2.5 and their seasonal variation: a case study in Baoding city, China. Ecotoxicol Environ Saf. 169:487–495.
- Xie JJ, Yuan CG, Xie J, Shen YW, He KQ, Zhang KG. 2019b. Speciation and bioaccessibility of heavy metals in PM2.5 in Baoding city, China. Environ Pollut. 252:336–343.
- Yoneda K. 1976. Mucous blanket of rat bronchus. Am Rev Respir Dis. 114:837–842.
- Zadpoor AA. 2014. Relationship between in vitro apatite-forming ability measured using simulated body fluid and in vivo bioactivity of biomaterials. Mater Sci Eng C Mater Biol Appl. 35:134–143.
- Zadrapova D, Titera A, Szakova J, Cadkova Z, Cudlin O, Najmanova J, Tlustos P. 2019. Mobility and bioaccessibility of risk elements in the area affected by the long-term opencast coal mining. J Environ Sci Health A. 54:1159–1169.
- Zereini F, Wiseman CL, Puttmann W. 2012. In vitro investigations of platinum, palladium, and rhodium mobility in urban airborne particulate matter (PM10, PM2.5, and PM1) using simulated lung fluids. Environ Sci Technol. 46:10326–10333.
- Zhong L, Hu X, Cao Z, Wang H, Chen Y, Lian HZ. 2019. Aggregation and dissolution of engineering nano Ag and ZnO pretreated with natural organic matters in the simulated lung biological fluids. Chemosphere. 225:668–677.
- Zhong MS, Jiang L. 2017. Refining health risk assessment by incorporating site-specific background concentration and bioaccessibility data of Nickel in soil. Sci Total Environ. 581-582:866–873.
- Zhong LJ, Liu XL, Hu X, Chen YJ, Wang HW, Lian HZ. 2020. In vitro inhalation bioaccessibility procedures for lead in PM2.5 size fraction of soil assessed and optimized by in vivo-in vitro correlation. J Hazard Mater. 381:121202.
- Zhong L, Yu Y, Lian HZ, Hu X, Fu H, Chen YJ. 2017. Solubility of nano-sized metal oxides evaluated by using in vitro simulated lung and gastrointestinal fluids: implication for health risks. J Nanopart Res. 19:375.
- Zhou Y, Cheng YS, Wang Y. 2010. Dissolution rate and biokinetic model of zirconium tritide particles in rat lungs. Health Phys. 98:672–682.
- Zoitos BK, DeMeringo A, Rouyer E, Thelohan S, Bauer J, Law B, Boymel PM, Olson JR, Christensen VR, Guldberg M, et al. 1997. In vitro measurement of fiber dissolution rate relevant to biopersistence at neutral pH: an interlaboratory round robin. Inhalation Toxicol. 9:525–540.