Abstract
For over a decade, the skin sensitization Adverse Outcome Pathway (AOP) has served as a useful framework for development of novel in chemico and in vitro assays for use in skin sensitization hazard and risk assessment. Since its establishment, the AOP framework further fueled the existing efforts in new assay development and stimulated a plethora of activities with particular focus on validation, reproducibility and interpretation of individual assays and combination of assay outputs for use in hazard/risk assessment. In parallel, research efforts have also accelerated in pace, providing new molecular and dynamic insight into key events leading to sensitization. In light of novel hypotheses emerging from over a decade of focused research effort, mechanistic evidence relating to the key events in the skin sensitization AOP may complement the tools currently used in risk assessment. We reviewed the recent advances unraveling the complexity of molecular events in sensitization and signpost the most promising avenues for further exploration and development of useful assays.
1. Introduction
Efforts to speed up and facilitate the replacement of animal experiments with human relevant, mechanistic, non-animal data in assuring chemical safety have led to remarkable changes in recent decades. This process has allowed a careful examination of the existing knowledge about the adverse outcomes resulting from the exposure to chemicals and consolidation of that knowledge into useful tools including Adverse Outcome Pathways (AOPs). Over a decade ago, the Organisation for Economic Co-operation and Development (OECD) launched a program to develop AOPs with the purpose of utilizing them for development of novel approaches and tests, the data from which can be combined and considered together to reach a decision about chemical safety (https://www.oecd.org/chemicalsafety/testing/projects-adverse-outcome-pathways.htm#SectionA). The efforts underpinned by the skin sensitization AOP have resulted in development and validation of numerous assays that, when combined, can confidently assign sensitizing potential of chemicals (or lack thereof). For those chemicals identified as sensitizers, potency information can be obtained using defined approaches (DAs). For example, a derivation of human relevant point of departure and quantitation of uncertainty is achievable using SARA model (Reynolds et al. Citation2019). Skin sensitization risk assessment remains a tiered approach (Gilmour et al. Citation2022) and for those chemicals identified as sensitizers, additional mechanistic information will be useful in the higher tiers of that approach (Reynolds et al. Citation2021). Here, we have reviewed the current and emerging mechanistic knowledge of the induction of skin sensitization which may add value to risk assessment.
1.1. Adverse outcome pathway
An adverse outcome pathway (AOP) is a concept describing a sequence of events starting from exposure of an organism (or specific tissue) to a chemical via a series of critical molecular and cellular events, culminating in an adverse outcome for the exposed organism/tissue. Originally described by Ankley et al. (Citation2010) AOPs are structured to represent the available knowledge which causally links the molecular initiating event (MIE) and an adverse outcome via one or more series of key events (KE).
The skin sensitization AOP, describing induction and elicitation phases required for adverse outcome to occur (manifested as allergic contact dermatitis (ACD) in humans), is one of the earliest developed AOPs (OECD Citation2014). It is summarized in 11 steps, including 4 which are recognized as “Key Events” (KEs1-4, ).
Figure 1. Overview of the skin sensitization AOP. KE1: Covalent binding to skin proteins, the MIE for skin sensitization. Bioavailable, electrophilic chemical must bind covalently to skin proteins, forming a complete antigen recognised by the immune system. KE2: Keratinocytes activation, resulting in upregulation of inflammatory cytokines and chemokines, and induction of cytoprotective gene pathways. KE3: APCs activation and presentation of haptenated proteins to T cells, leading to migration of activated T cells into circulation, completing induction phase of sensitization. KE4: T cell proliferation in response to the haptenated protein presented by APCs, leading to the activation of memory T cells. Adverse outcome (ACD): the clinical manifestation of skin sensitization (elicitation) occurs on subsequent exposure to the same or a cross-reactive chemical. Each KE described above also occurs during elicitation, with some modification. The sensitizing chemical binds covalently to skin proteins, which are then processed and presented to the memory specific T cells. Memory T cells are attracted to the skin site of the exposure by the increased secretion of inflammatory cytokines by keratinocytes. The elicitation phase culminates in the inflammatory response local to the site of exposure to the same (or cross-reactive) chemical the individual has previously been sensitized to. Image: NEXU Science communication.
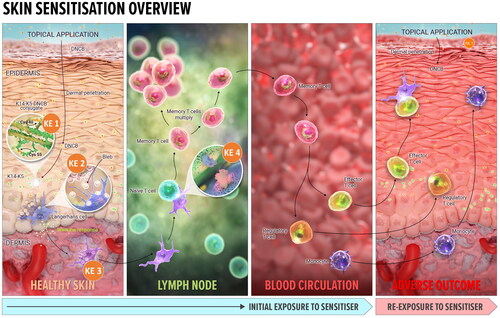
The skin sensitization AOP is focused solely on organic chemicals which are inherently electrophilic or can be transformed into electrophilic species via phase I skin metabolizing enzymes or on contact with air oxygen (i.e. pro- and pre-haptens). This AOP does not currently include the type of ACD which develops as a result of exposure to metals or chemicals activated by UV light exposure (reviewed recently by de Ávila et al. (Citation2023a)).
1.2. Current predictive assays
Prior to the development of the skin sensitization AOP, efforts were already underway to develop predictive non-animal assays for skin sensitization based on events that were well understood to occur during the induction of sensitization. Establishment of the AOP has, however, provided a framework together with a mechanistic knowledge base, which has further facilitated in chemico and in vitro assay development as well as their integration into “defined approaches” (DAs) for risk assessment of skin sensitization (OECD Citation2023a). This has also facilitated development and further refinement of several in silico tools, providing useful information on chemistry and mechanistic domain of sensitizers (e.g. DEREK Nexus, OECD QSAR Toolbox, TIMES, ToxTree).
To date, there are nine developed and validated New Approach Methodologies (NAMs, in chemico and in vitro tests) that have achieved OECD test guidelines (TG) status (). Each of the test methods/assays addresses a KE in the skin sensitization AOP. OECD TG 442 C describes the direct peptide reactivity assay (DPRA), amino acid derivative reactivity assay (ADRA) and the kinetic DPRA (kDPRA), which are test methods addressing KE1 (the binding of haptens to proteins in the skin (OECD Citation2023b)). OECD TG 442D describes the KeratinoSensTM and LuSens methods which address KE2 (the activation of keratinocytes (OECD Citation2022a)). OECD TG 442E describes the Human Cell Line Activation test (hCLAT), U937 cell line activation Test (USensTM), Interleukin-8 Reporter Gene Assay (IL 8 Luc assay) and Genomic Allergen Rapid Detection (GARD™) for assessment of skin sensitizers (GARDTMskin) which are test methods addressing KE3 (the activation of dendritic cells (DCs)) (OECD Citation2023c)). With the exception of the GARDTMskin assay in certain circumstances (JRC EC Citation2021), these NAMs should not be used alone for hazard and potency categorization, but the output should be combined with information from other NAMs within a DA.
Figure 2. Predictive tools for risk assessment aligned to skin sensitization AOP. Current set of non-animal methods (NAMs) developed for key events in skin sensitization, underpinned by the AOP framework include: the direct peptide reactivity assay (DPRA) (Gerberick et al. Citation2004; Citation2007), amino acid derivative assay (ADRA) (Fujita et al. Citation2014; Yamamoto et al. Citation2015; Fujita et al. Citation2019) as well as kinetic DPRA (kDPRA) (Roberts and Natsch Citation2009; Wareing et al. Citation2017; Natsch et al. Citation2020), all included in the OECD TG 442 C (OECD Citation2023b); KeratinoSens TM (Emter et al. Citation2010; Natsch and Emter Citation2016) and LuSens (Ramirez et al. Citation2014) included in the OECD TG 442D (OECD Citation2022a); human cell line activation test (hCLAT) (Ashikaga et al. Citation2006), U937 cell line activation Test (U-SENS™) (Piroird et al. Citation2015), Interleukin 8 Reporter gene assay (IL 8 Luc assay) (Takahashi et al. Citation2011) and Genomic allergen rapid detection for assessment of skin sensitizers (GARD™skin) (Johansson et al. Citation2011, Citation2013) included in the OECD TG 442E (OECD Citation2023c). In addition to the above tools, numerous predictive chemistry (in silico) tools are also available (dotted box). In vivo evidence (red box) comes from mouse local lymph node assay (LLNA) (OECD Citation2010a) and its variants (OECD Citation2010b, Citation2018) and Buehler and Guinea pig maximization Test (GPMT) (OECD Citation2022b). The LLNA, in fact, addresses KE4, measuring T cell proliferation in treated mice rather than established sensitization.
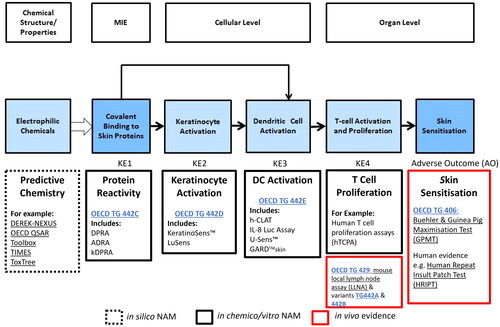
Three DAs are currently included in the OECD guideline 497 (OECD Citation2023a) which allow data from the individual assays to be integrated for chemical classification (either binary (sensitizer/non-sensitizer) or the United Nations Globally Harmonized System of Classification and Labelling of Chemicals (UN GHS) hazard subcategories 1A (strong sensitizer) or 1B (moderate or weak sensitizer) (UN Citation2021)); reviewed by (Ezendam et al. Citation2016; Kleinstreuer et al. Citation2018)). The outputs from the majority of predictive assays do contain a quantitative component, however, use of quantitative information within the DAs currently described in OECD 497 is somewhat limited. The most recent effort is utilization of quantitative output from reactivity assays to redefine thresholds for improved UN GHS classification (Alépée et al. Citation2023).
Better utilization of the quantitative outputs and more refined predictions of the skin sensitization potency has been achieved in recently developed DAs. The SARA model allows derivation of a human relevant point of departure with quantifiable uncertainty and a risk metric for use in quantitative risk assessment (Reynolds et al. Citation2019) and has recently been adapted in collaboration with NICEATM to address UN GHS classification (Reynolds et al. Citation2023, manuscript in preparation). Additional DAs include Bayesian Integrated Testing Strategy (ITS) (Jaworska et al. Citation2013), Artificial Neural Network (Tsujita-Inoue et al. Citation2015) and a series of linear regression models (Natsch and Gerberick Citation2022a, Citation2022b; Natsch Citation2023). The value of these DAs within a risk assessment for skin sensitization has been recently explored using case studies (Gilmour et al. Citation2020, Citation2022, Citation2023; Reynolds et al. Citation2021; OECD Citation2022c).
Currently, there are no NAMs addressing KE4 (the activation and proliferation of a T cell response), which are sufficiently progressed for implementation in OECD TG or for use in a Next Generation Risk Assessment (NGRA) (van Vliet et al. Citation2018).
SENS-IS and GARDskinTM dose response assays (Cottrez et al. Citation2015, Citation2016; Gradin et al. Citation2021), which cover multiple KEs, show some promise in providing more quantitative skin sensitizing potency assessment by predicting a skin sensitization class (strong/moderate/weak/non-sensitizing). However, it remains to be seen how these can be utilized in risk assessment.
1.3. Current mechanistic research and future possibilities
The current AOP, which serves as a backdrop for the development of predictive assays for sensitization, is somewhat linear in nature, implying that events occur one after the other in an orderly sequence. However, as most of the events described above directly result from the electrophilicity of a topically applied chemical, it is more likely that they happen concomitantly or that they significantly overlap. As evident from investigations of haptenation and metabolism of 2,4-dinitro-1-chlorobenzene (DNCB) in HaCaT cells, interactions with cell surface proteins, relevant detoxification mechanisms as well as cell defense mechanisms otherwise capable of undoing or ameliorating the damage from protein haptenation most likely occur concomitantly to antigen formation (Jacquoilleot et al. Citation2015; Parkinson et al. Citation2020b). This represents an opportunity to “branch” the AOP in certain areas to gain better mechanistic understanding of each KE and their potential overlap. It is possible that this could facilitate more meaningful data integration in the future and increase the biological relevance of sensitization potency predictions.
Currently, quantitative reactivity data appears to be the single most valuable parameter when predicting the potency of skin sensitizers (Natsch and Emter Citation2017). Increasing our understanding of how chemical reactivity “plays out” in the skin tissue may be of additional value in making further progress to improve the current risk assessment of a variety of chemicals with different chemical mechanisms. Determining the optimal ways to measure reactivity related to antigen generation as well as additional events associated with reactivity and deeper quantitative understanding of cell stress pathways involved represent research avenues that could be explored further. This would facilitate further discussion on each of the KEs and strengthen the evidence on the relevance of each parameter that may be useful in decision making. The value of reviewing and furthering the detailed understanding of KEs in skin sensitization AOP lies in keeping the information up to date and motivating discussion to use new scientific insights to constantly look to improve our safety assessments, rather than as a critique of the existing or emerging test methods or the DAs currently used or under development. While predictive assays need not be complicated and exactly replicate the entirety of events occurring in the skin, to be informative in the risk assessment, they should enable a reliable and confident assessment of the risk posed to individuals exposed to electrophilic chemicals and be used as a part of a tiered approach (Gilmour et al. Citation2022). Currently, in chemico/in vitro predictive assays used within DAs do not incorporate NAMs based on some of the additional mechanistic insights into the MIE in skin sensitization and this information might be valuable in risk assessment decisions.
We consider the current understanding of the KEs in the skin sensitization AOP, discuss the challenges posed by the linear interpretation of the AOP and introduce novel reasoning of how new and emerging hypotheses on individual KEs and their potential overlap could be investigated, measured, and potentially utilized in design of future predictive assays and DAs for potential use in future skin sensitization risk assessment.
2. Methods
An already existing body of literature the authors are familiar with was used as a starting point for this review. In particular, a journal article by Natsch and Emter (Citation2017) served as an inspiring body of work suggesting further mechanistic investigation of chemical reactivity may provide significant and useful insights into underlying factors affecting sensitizer potency of chemicals. An NIH PubMed (https://pubmed.ncbi.nlm.nih.gov/) search of peer reviewed journal articles was conducted up to 1 August 2023 using generic terms “skin allergy,” “contact sensitization,” “protein haptenation,” etc., with the intention to source suitable articles for review of the mechanistic research in skin sensitization over the past couple of decades, in particular focusing on the period since the publication of the skin sensitization AOP (OECD Citation2014). The search terms were widened to include journal articles which deal with phenomena associated with skin sensitization in some mechanistic aspects, in particular including the effects of electrophilic chemicals on biological systems and associated events (chemical metabolism, detoxification, lipid peroxidation etc.). The data and conclusions from the relevant journal articles were critically evaluated by the authors and used to support the discussion on key events of the AOP in sensitization. The focus remained on widening the knowledge of AOP key events and exploring the potential to develop novel and useful insights and assays for higher tier risk assessments.
3. Results
3.1. Reactivity and consequences
Electrophilic reactivity of a sensitizer, either direct (hapten) or acquired by air oxidation (pre-hapten) or metabolic activation (pro-hapten), has long been understood as a prerequisite for sensitization (Landsteiner and Jacobs Citation1935). Otherwise “invisible” to the immune system, the (pro/pre) electrophilic chemical forms a complete antigen by covalently binding to nucleophilic side chains of amino acids in skin proteins. This MIE starts the sequence of events which ultimately lead to sensitization and understanding this has enabled development of in chemico reactivity assays and their subsequent refinements. Reactivity assays today are an integral part of non-animal risk assessment of chemicals for skin sensitization potential (e.g. DPRA, ADRA, and kDPRA, see ).
The majority of reactivity assays (originally developed in 2004 and included in the OECD TG 442 C (OECD Citation2023b)) measure the depletion of the limited number of target nucleophile(s) at a single time point (typically 24 h), except kDPRA, which assesses kinetics of reactivity with Cys peptide at multiple time points. Importantly, nucleophile (peptide) depletion measures the level of haptenation indirectly. As such, it often captures oxidative depletion (of Cys peptide) rather than purely haptenation-induced depletion. The mechanisms of Cys peptide oxidative dimerization are not yet explained (Natsch and Emter Citation2017), but it is likely that this reaction also occurs in keratinocytes and potentially contributes toward overall oxidative stress. Additional reactivity assays developed since then and not included in the OECD TG 442 C can add valuable information for risk assessment. These involve use of multiple nucleophiles, investigating reaction mechanism(s) and/or measurement of the initial rate of reaction (Natsch et al. Citation2007; Natsch and Gfeller Citation2008; Aleksic et al. Citation2009; Chipinda et al. Citation2010, Citation2014; Sanderson et al. Citation2016). Perhaps the most valuable development is classification of sensitizers into mechanistic reactivity domains (Roberts et al. Citation2007) which can be used for prediction of sensitization potency using reaction kinetics and hydrophobicity parameters (within the constraints of Michael acceptor (MA) and some parts of Schiff base (SB) reactivity mechanistic domains (Roberts and Natsch Citation2009; Roberts et al. Citation2017)). However, complex reactivity mechanisms and reactions with multiple nucleophiles, including crosslinking, are not easily captured in reactivity assays and remain difficult to consider in decision making.
Since the original design of the now widely used reactivity assays in 2004, the understanding of the complex nature of protein haptenation and some aspects of how the inherent reactivity of the chemicals results in generation of specific complete antigens when bound to proteins has considerably improved. Relevant mechanistic studies and emerging hypotheses are discussed below.
Utilizing fluorescence of bromobimanes’ adducts, two studies have confidently identified epidermal keratin complexes as major haptenation targets (keratin 5 (K5) in mouse skin (Simonsson et al. Citation2011) and both K5 and K14 in human epidermal keratinocytes (Bauer et al. Citation2011)). Keratins are highly abundant heterodimeric pairs of type I and type II keratins which make up the cytoskeletal keratin intermediate filament network in epithelia. In human epidermis, K5 and K14 are found in the basal layers, whereas suprabasal layers express another heterodimeric keratin pair, K1 and K10, which are extensively crosslinked and far less likely to have available Cys residues for haptenation (Moll et al. Citation2008). Cys 54 of K5 was shown as one of the main bromobimane targets. These two remarkable studies have shown that haptenation in living cells and tissues is relatively rare event, given that protein concentration far exceeds that of the hapten. Another study located a specific fluorescent tetramethylrhodamine isothiocyanate (TRITC) modification on unusually reactive N-terminal proline of migration inhibitory factor (MIF) in lymph nodes and blood of mice topically treated by TRITC, suggesting MIF may have an important immunomodulatory role in sensitization (Karlsson et al. Citation2018; Ndreu et al. Citation2022). This additionally demonstrates utility of the fluorescent adduct approach for studying haptenation of sensitizers which react with amine based nucleophiles (Patlewicz et al. Citation2002), but remains applicable only to electrophiles which make fluorescent adducts.
Studying correlation between reactivity and sensitization potency, Natsch and colleagues investigated the anti-inflammatory effect Michael acceptors are exhibiting via inhibition of nuclear factor-kappa B (NFκB) signaling, which appears to dampen their pro-inflammatory sensitizing effect and appear less potent. Cyclopentenone prostaglandins (Natsch et al. Citation2011), 4-hydroxy-2-nonenal (4HNE) (reactive carbonyl species (RCS), which are end product of lipid peroxidation (Ji et al. Citation2001)) and parthenolide (Kwok et al. Citation2001) modify IκB kinase (IKK), whereas sesquiterpene lactones directly target p65 of NFκB (Lyss et al. Citation1998; García-Piñeres et al. Citation2001). This is in contrast to SN2 and SNAr reactive sensitizers, which are not inhibiting this signaling cascade (Natsch et al. Citation2011). Clearly, studying specific haptenation targets may help us interpret the reactivity of sensitizers more accurately in the context of sensitization potency.
Elbayed et al. (Citation2013) showed the utility of the high-resolution magic angle spinning nuclear magnetic resonance (HR MAS NMR) to compare haptenation of human serum albumin (HSA) and reconstituted human epidermis (RHE) by a model sensitizer methyldodecanesulphonate. The critical comparison of haptenation “in solution” using a model protein Vs haptenation in a 3D model of human epidermis showed a considerably faster haptenation in tissue (detectable by 24 h compared to days with HSA). Chemoselectivity for the protein nucleophiles differed significantly between models, with Lys being targeted on HSA, and His, Met, and Cys found modified in RHE. Two further studies using this approach (with α-methylene-γ-butyrolactone (Debeuckelaere et al. Citation2015) as well as 2-methylisothiazolin-3-one (MI) and 5-chloro-2-methylsothiazolin-3-one (MCI) (Debeuckelaere et al. Citation2016)) confirmed the initial kinetic and chemoselectivity findings of Elbayed and colleagues and demonstrated the importance of reactivity toward multiple nucleophilic residues, namely His. While the HR MAS NMR studies detect the nucleophilic residues and do not identify haptenated proteins as such, they clearly demonstrate that nucleophile targeting and the dynamics of the haptenation are not fully represented in the current in chemico reactivity assays. However, previous efforts to include His in reactivity assays, have demonstrated poor sensitivity of His peptide depletion as well as poor predictivity compared to Cys and Lys peptides (Gerberick et al. Citation2004).
The sparse protein haptenation was detected using sensitive stable isotope labeling, initially with single proteins and relevant protein mixtures (HaCaT cell and human skin lysates) (Parkinson et al. Citation2014, Citation2018, Citation2020a). This method was further utilized with living HaCaT cells treated with DNCB, adding the quantitative analysis of proteome (Parkinson et al. Citation2020b). Minimal changes in overall protein differential expression were observed in HaCaT cells while DNCB haptenated approximately 0.25% of all available nucleophiles when applied at a subtoxic concentration (10 μM) for 4 h. This vanishingly low level of haptenation by an extremely potent sensitizer is in contrast with observations from reactivity assays, where DNCB depletes 100% of target peptides. The sites of modification on individual proteins were detected, however, haptenation could not be directly quantified. While reactive with most nucleophiles in reactivity assays, DNCB showed a preference here for Cys residues, despite the considerably higher concentration of amine-based nucleophiles. Although a proportion of highly abundant proteins were haptenated, including K5 and K14 (seen haptenated by bromobimanes previously), haptenation was also detected on low abundant proteins. Interestingly, maximum haptenation occurred at 2 h and was negligible at the end of the time course (48 h), indicating that DNCB haptenation may have been reversed. This is in contrast to the current consensus opinion that the covalent modifications are irreversible (OECD Citation2014). Similar dynamics were mirrored by a study using DNCB at the identical subtoxic concentration and same cell line which quantitatively investigated time course of the main phase II metabolism of DNCB, binding to glutathione (GSH) (Jacquoilleot et al. Citation2015). In the glutathione-s-transferase (GST) catalyzed process, 2 h timepoint showed maximal depletion of GSH, with levels recovering from 4 h onward. A more complex study of GSH cycle upon repeated DNCB treatment was using RHE model and has shown a complete recovery of the levels of GSH after three consecutive daily doses of DNCB (Spriggs et al. Citation2016).
Despite only a few distinct chemicals having been investigated in the complex studies described above, new hypotheses are emerging from these insights. It is evident that haptenation develops immediately post-exposure to the electrophilic chemical which concomitantly triggers a variety of associated events in epidermal cells. Some of this knowledge has already been utilized for development of predictive assays (e.g. KeratinoSensTM (Natsch and Emter Citation2016; OECD Citation2022a)). Other associated events, such as damage to proteins by elevated reactive oxygen species (ROS), lipid peroxidation (LP) and secondary damage to proteins from end products of LP (RCS) have not had as much attention.
Three emerging areas of interest which are either a direct consequence of chemical reactivity of sensitizers or are events associated with the initial external electrophilic stress are discussed below with suggestions how exploring these events mechanistically and quantitatively may be useful in predicting sensitizer potency ().
Figure 3. Molecular initiating event (MIE) in skin sensitization by 1,4-dinitro-2-chlorobenzene (DNCB). Besides antigen formation ((a) covalent binding of DNCB to specific cell proteins), additional concomitant and associated events ((b) detoxification, (c) oxidative balance disturbance, (d) lipid peroxidation, (e) covalent modification reversal, (f) activation of the Nrf2 pathway) and (g) covalent protein damage from reactive carbonyl species (RCS, end products of lipid peroxidation) are shown. Image: NEXU Science communication.
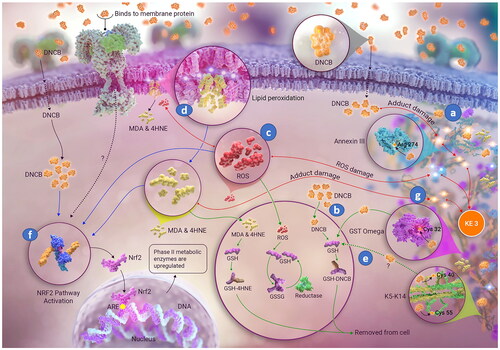
3.1.1. Skin metabolism
Metabolism research in skin sensitization has been focused on phase I metabolic processes which may be responsible for activation of pro-haptens. Conceptually, the formation of reactive metabolites by phase I metabolism in human skin is well established, although in vitro demonstration is still relatively limited (Niklasson et al. Citation2014; Reynolds et al. Citation2021).
However, current understanding of phase I skin metabolism and abiotic activation (e.g. air oxidation) have been used in the adaptations to existing reactivity assays, aiming to incorporate “activation” of nonelectrophilic chemicals to their electrophilic metabolites which then bind to the model nucleophiles (e.g. Peroxidase Peptide Reactivity Assay (PPRA) (Gerberick et al. Citation2009; Troutman et al. Citation2011; Ryan et al. Citation2020). The utility of current in chemico and in vitro assays for detection of pre- and pro-electrophiles has been a subject of reviews (Patlewicz et al. Citation2016; Urbisch et al. Citation2016). To date, little emphasis has been placed on the activity of phase II metabolizing enzymes in epidermis which are responsible for effective “removal” of a variety of electrophilic molecules by either binding them to substrates generating non-reactive products or converting the electrophiles to related unreactive molecules.
Oesch and colleagues updated their comprehensive review of skin metabolizing enzymes in 2014 (Oesch et al. Citation2014), reviewing and comparing numerous studies which investigated either mRNA levels, protein levels or activity of a vast array of metabolizing enzymes (including non-CYP metabolizing enzymes, such as aldehyde oxidases/dehydrogenase (AO/ALDH), NAD(P)H quinone reductase (NQR), hydrolases (epoxide hydrolase (EH), esterases/amidases), and conjugating enzymes, such as glutathione-s-transferases (GSTs), UDP-glucuronosyl transferases (UGTs), sulfotransferases (SULTs) and N-acetyl transferases (NATs)). The broader role of detoxifying enzymes in drug hypersensitivity was subject of a review (Sánchez-Gómez et al. Citation2016), highlighting the evidence supporting the involvement of GSTs and aldo-keto reductases in development of a variety of allergic responses as well as interaction of these key detoxifying enzymes with oxidative stress molecules, both endogenous and external electrophiles and inflammatory mediators. While it can be challenging to confidently detect and measure activity of some metabolizing enzymes in human skin, 3D skin/epidermal models and skin relevant cell lines, it is accepted that majority of relevant metabolizing enzymes are present and detoxification is observable in vitro and ex vivo (Manevski et al. Citation2015; Kazem et al. Citation2019). However, although stable in models, levels of protein expression of certain metabolizing enzymes and consequently their metabolic activity may vary in individuals as well as at a population level (Spriggs et al. Citation2018; Buratti et al. Citation2021).
The phase II metabolism of electrophilic molecules is closely linked to the reactivity mechanism. For example, SN2 chemicals and MAs are conjugated to GSH, removed out of the cell and processed to the relevant mercapturic acid conjugate for excretion, whereas SB formers and acylators are metabolized by AO/ALDH (). When a sensitizer has more than one electrophilic center, there may be more than one mechanism involved in completely removing the sensitizing potential of that molecule. For example, cinnamaldehyde reacts via MA to thiols and via SB formation to amine nucleophiles. Thus, cinnamaldehyde α,β-unsaturated moiety undergoes conjugation to GSH catalyzed by GSTs and its carbonyl moiety is dealt with by an AO/ALDH converting it to cinnamic acid.
Table 1. Likely phase II metabolic fate of different mechanistic groups of sensitizers.
The phase II metabolic reactions are inevitably faster than spontaneous protein haptenation, due to enzyme catalysis and high concentrations of cofactors (e.g. cellular GSH concentration is approx. 5 mM in most cells (Pizzorno Citation2014)). Most electrophiles are subject to these cellular defense mechanisms, effectively preventing the damage they may exert by covalently modifying proteins. This can explain the vanishingly low and, until recently, almost undetectable level of haptenation by non-cytotoxic concentration of even a very potent sensitizer, such as DNCB (Parkinson et al. Citation2020b).
Considering the reactivity of sensitizer in the context of detoxification may help explain extreme potency of certain sensitizers. For example, DNCB is an extreme sensitizer and depletes 100% of Cys peptide in standard reactivity assays (Gerberick et al. Citation2004). However, DNCB is found to covalently modify the active center of GST-ɷ (, b), the enzyme which catalyzes the phase II metabolism of DNCB, possibly disabling the enzyme (Bailey et al. Citation2021). Another extreme sensitizer, 5-Chloro-2-methylisothiazol-3-one (MCI), is activated by thiols, including GSH and two further highly reactive intermediates are generated in this process (Alvarez-Sánchez et al. Citation2004a; Citation2004b). Thus, instead of effective detoxification, the GSH acts as a catalyst, increasing the reactivity of MCI. These two extremely potent sensitizers appear to impede their removal from the cell. Further instances of sulphydryl based (e.g. GSH) activation of sensitizers have recently been shown for isothiocyanates (Karlsson et al. Citation2016) and 2-bromo-2-(bromomethyl)glutaronitrile (MDBGN) (Ndreu et al. Citation2020). It is also known that some GSH conjugates can be converted into reactive, toxic intermediates (Anders Citation2008; Cooper and Hanigan Citation2018).
Main phase II metabolic mechanisms apply to both externally applied and endogenously generated electrophilic molecules (e.g. RCS). These are mainly aldehydes, dialdehydes or α,β-unsaturated aldehydes, and it is likely that their increased level would impact the capacity of GST and AO/ALDH detoxification mechanisms. It may be important to determine the level of RCS generated by exposure to sensitizers to be able to fully assess the detoxification capacity and how this influences the potency for skin sensitizers competing for the same detoxification mechanisms.
Quantifying the phase II metabolic capacity of the human keratinocytes for the main mechanistic domains of reactive chemicals should enable understanding of how this process competes with protein haptenation and at which concentration the electrophilic chemical could overwhelm the cellular defense mechanisms. Reactivity rates of reaction of sensitizers with simple nucleophiles are already available in chemico (e.g. aldehydes reactivity with butylamine (Natsch et al. Citation2018) and electrophiles with glutathione (Schultz et al. Citation2005)), although inclusion of enzymatic activity (in skin extracts or using isolated enzymes) might give a more relevant estimation of detoxification in vivo. This can be applied to the chemicals with a single mechanistic domain as well as those with more than one reaction mechanism.
3.1.2. Oxidative stress and end products of lipid peroxidation (LP)
Oxidative stress is part of keratinocyte inflammation, currently considered under the KE2 in the AOP. This is a direct consequence of electrophilicity of sensitizers, and a molecular basis for secondary inflammatory effects also described in KE2. Electrophile presence in keratinocytes disturbs the redox balance, either by direct binding to cellular antioxidants (such as GSH), oxidative dimerization of thiols (e.g. GSH to GS-SG) or via radical mechanisms (directly or following activation, e.g. hydroperoxides). Disruption of redox balance by engaging the main cellular antioxidant inevitably leads to increase in ROS (, c), which, in turn, disturbs cellular homeostasis via: (1) irreversibly damaging cellular proteins (attacking the nucleophilic sites and the protein backbone); (2) attacking the polyunsaturated fatty acids (PUFAs) (including disrupting the cell membrane) and initiating LP (, d) which results in formation of endogenous electrophiles (or RCS) as well as (3) additionally activating the nuclear factor erythroid 2-related factor 2 (Nrf2) pathway (, f)), ultimately increasing the transcription of the phase II metabolizing enzymes in the cell.
Increased ROS results in the accumulation of the unfolded proteins in the endoplasmic reticulum (ER) and activation of the unfolded protein response (UPR) (Read and Schröder Citation2021). This ROS dependent phenomenon has been demonstrated in a THP-1 cell line treated with a potent skin sensitizer 1-fluoro-2,4-dinitrobenzene (DNFB) (Luís et al. Citation2014). Increase in ROS is, therefore, likely to speed up the processing of haptenated proteins.
Redox balance disruption deepens the damaging effect by enabling elevated ROS to interact with cellular and organelle membranes, where a phospholipid bilayer is made up of hydrophilic head group and hydrophobic acyl chains of saturated, monounsaturated, and polyunsaturated fatty acids. Epidermis and keratinocyte cell cultures contain a large proportion of saturated fatty acids (FAs), and of the polyunsaturated fatty acids (PUFAs), only lineloic acid (LA, 18:2 ɷ-6) and arachidonic acid (AA, 20:4 ɷ-6) are detected at higher levels and vary considerably between freshly isolated epidermis and cultured keratinocytes (Ponec et al. Citation1988; Schürer et al. Citation1993). LP involves ROS attack on the carbon-carbon double bonds of the PUFAs (, d), often in the phospholipid bilayer of membranes (Pamplona Citation2011; Łuczaj et al. Citation2017). ROS initiates LP, however, the progression of this reaction is not further impacted by ROS levels. It is, instead, driven by the proximity of the peroxidizable fatty acid chains (Zimniak Citation2011). Self-propagating fatty acid peroxyl radicals of ɷ-6, but also ɷ-3 PUFAs, ultimately produce, via series of cleavages, a collection of over 30 biologically active structurally related electrophilic molecules known as RCS (end products of LP) () (Kawai et al. Citation2007; Negre-Salvayre et al. Citation2008). Unlike ROS, RCS have longer half-life and are capable of covalent modification of other biomolecules, in particular proteins, causing “endogenous electrophilic stress” (Marnett et al. Citation2003).
Figure 4. Structures of some reactive carbonyl species (RCS), end products of lipid peroxidation (LP). The most abundant and most often studied RCS are malondialdehyde (MDA) and 4-hydroxynonenal (4HNE). A large body of literature details extensive investigations of generation, known metabolic routes and effect on certain signaling events, cellular Pool of protein and pathologies associated with production of MDA and 4HNE (e.g. Alzheimer’s disease, Parkinson disease, liver disease, diabetes, cardiovascular disease and cancer) (reviewed by but not limited to) (Ayala et al. (Citation2014)).
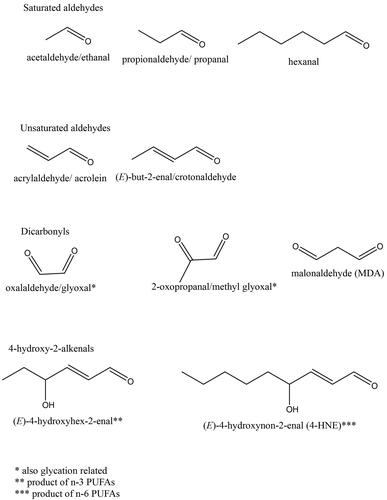
RCS have multiple roles, ranging from cell signaling and preventing irreversible oxidative protein damage at low concentrations to damage inducing at high concentrations (Domingues et al. Citation2013; Castro et al. Citation2017; Mol et al. Citation2017; Zhang and Forman Citation2017). RCS covalently modify proteins via MA reactions and SB formation, much like some externally applied sensitizers, which they resemble. Proteins covalently modified by RCS (carbonylated proteins), are often processed by the proteasome 20S, but can be ubiquitinated and processed by the proteasome 26S or, indeed, by lysosomes where, due to low pH and active acid hydrolases, SB adducts can be reversed (Zhang and Forman Citation2017).
From a structure and reactivity perspective, RCS resemblance of well-known skin sensitizers raises the obvious question of the immunogenicity of their protein adducts. Indeed, there is accumulating evidence that malondialdehyde (MDA) modifications of certain proteins act as oxidation-specific epitopes capable of triggering both innate and adaptive immune response (Papac-Milicevic et al. Citation2016). Only two RCS molecules have been tested in sensitization assays and classified as human sensitizers (glyoxal (Gerberick et al. Citation2005; Basketter et al. Citation2014) and hexanal in DPRA (Kawakami et al. Citation2020)). It is easy to assume that, if tested, RCS could be determined as topical sensitizing chemicals, however, realistically, the sensitizing concentrations should far exceed those that are generated endogenously. Under oxidative stress conditions, keratinocytes are likely producing a variety of RCS, which are collectively damaging, but individually should not reach the concentration required to provoke a specific immune response. Additionally, certain LP end products are metabolized and routed into other cellular processes (e.g. MDA and acetaldehyde are converted to acetate; methyl glyoxal is converted into pyruvate and both enter the citric acid cycle (Negre-Salvayre et al. Citation2008; Ayala et al. Citation2014)), potentially lowering the chances of eliciting a skin reaction in an individual topically sensitized to an LP end product.
The type and level of individual RCS generated under oxidative stress conditions is entirely dependent on the PUFA composition of the cells (Zimniak Citation2011). The susceptibility of PUFAs to peroxidation increases proportionately with their level of unsaturation and can be determined by calculating the “peroxidation index” for a known PUFA composition (Hulbert et al. Citation2014). This could be one of the important sources of variability in individual sensitization thresholds.
All cells, including keratinocytes, have adapted and developed defense mechanisms to rapidly sequester RCS or metabolize them into other useful molecules and repair the damage they cause (Marnett et al. Citation2003; Sousa et al. Citation2017). However, it is important to consider the level and type of RCS generated during the induction of sensitization, given that their reactivity is resulting in cellular interactions chemically mirroring those of sensitizers (, g). Dependent on the type and level of RCS generated, they may act as competition for detoxification mechanisms, impairing the cellular ability to remove the sensitizer effectively; contribute to further activation of the UPR and “speed up” the processing of haptenated proteins and/or as additional signal to increase the cellular ability to remove the electrophiles, for example by activating the Nrf2 pathway.
3.1.3. Stability of covalent adducts
We explored the literature for evidence of (in)stability and reversibility of covalent modifications both in skin sensitization field and beyond, namely in studies examining reactions of LP end products, RCS.
Time course studies of DNCB haptenation (Parkinson et al. Citation2020b) and GSH cycle in DNCB treated HaCaT cells (Spriggs et al. Citation2016) show that protein haptenation peaks at 2 h, coinciding with maximum depletion of GSH. The level of haptenation is negligible at 48 h, indicating that either the previously haptenated proteins have been removed somehow from the samples or that the modifications have been reversed. The latter scenario seems more plausible for several reasons: (1) the haptenation experiments were conducted in a closed system; (2) the whole sample was lysed at a given time point and, thus, it contains the entire proteome, including proteins that might have been “expelled” from the cells; (3) there was no obvious way to systematically remove the haptenated proteins; and (4) it is energetically more favorable for the cell to repair the damage to haptenated proteins by removing the covalently bound hapten than to undergo de novo protein synthesis. While none of the above reasons constitutes a proof that covalent modifications by DNCB are reversed, it points us strongly in the direction of exploring the possibility of this occurring (, e).
Natsch and Emter exemplified occurrences of “sequential” reactivity of electrophiles with different amino acid residues (Natsch and Emter Citation2017). Using MCI, methyl isothiazolinone (MI), oxazolone, isocyanate, isothiocyanate, and an anhydride as case studies, an initial reaction with thiol (protein Cys residue or GSH) is described, followed by a final, more stable adduct detected on an amine (Lys or His residues on proteins). The article does not explicitly discuss haptenation reversibility in the context of hapten removal and ultimate detoxification, however, it does point out lack of full understanding of the complex reactivity of sensitizers.
Outside of skin sensitization field, experimental reversal of SB adducts is found in literature reporting measurements of “free” and “total” levels of RCS where acidification is often used experimentally to remove the SB conjugated MDA and subsequently capture it by thiobarbituric acid (TBA) (e.g. Spickett et al. Citation2010). Haptenated proteins are processed in an acidic environment of lysosomes, where acid hydrolases are active at low pH (Cooper Citation2000), likely reversing SB adducts. Furthermore, to prevent the known spontaneous reversibility of SB adducts in biological samples, sample preparation (e.g. for proteomic analyses) often includes “stabilization” step (e.g. Han et al. Citation2007; Eggink et al. Citation2008). This involves a reaction with sodium borohydride ((Billman and Diesing Citation1957)), which reduces the -C = N- bond in SB to a more stable -CH2-NH- making the adduct less likely to be hydrolyzed and, therefore, more likely to be detected.
Reversibility of several types of GSH adducts has been discussed as a spontaneous event (Baillie and Kassahun Citation1994). A recent study investigating 4-hydroxynonenal (4HNE) site specific binding in human colon carcinoma cell line treated with an analogue of 4HNE, showed that MA adducts rapidly disappear in intact cells (Yang et al. Citation2015). The mechanism of this potential reversal remains unknown, however, sporadic evidence shows that some MA adducts can be reversed by excess thiol (often GSH) (Mol et al. Citation2017). Some reports detail design of reversible MA electrophiles (Lee and Grossmann Citation2012; Krishnan et al. Citation2014). Another important component of cellular defense against ROS, thioredoxin (Trx) and its associated enzyme thioredoxin reductase (TrxR) comprise a system capable of reducing the disulfide bonds of oxidized Trx, but also other chemical entities, such as lipid hydroperoxides (Nordberg and Arnér Citation2001). There is, however, no direct evidence that this system can reduce S-C bonds formed during MA reaction.
Certain strong or extremely potent sensitizers (Gerberick et al. Citation2005) have more than one reaction mechanism or react via complex mechanisms. It is plausible that complex adducts and crosslinks are less likely to be reversed (or are energetically “costly” to reverse) and that this could explain their high sensitization potency. For example, glutaraldehyde is a strong sensitizer capable of formation of strong crosslinks between (and within) proteins (Bowes and Cater Citation1968), making it a very useful reagent in many experimental procedures (Migneault et al. Citation2004). Another potent sensitizer, formaldehyde, makes initial methylol adduct with amine nucleophiles which is dehydrated to a SB and known to be unstable. However, formaldehyde adducts continue to react beyond this initial step with other amino acids within close proximity forming methylene bridges and imidazolinone adducts (Metz et al. Citation2004, Citation2006). Further complex crosslinks following interaction with formaldehyde were recently described (Michiels et al. Citation2020). An extreme sensitizer, MCI, mentioned earlier, reacts via complex mechanism to protein nucleophiles (Alvarez-Sánchez et al. Citation2004a, Citation2004b).
Mechanistically, it is unclear how covalent adducts could be reversed in skin for majority of reactions in sensitization and whether this phenomenon has a quantitative impact on kinetics of antigen formation. It is evident that some adducts are more readily reversible than others and plausible that reversing covalent adducts is, in fact, an “extended arm” of cellular detoxification mechanism.
3.1.4. Quantitative combination of early reactivity-related events
The impact of skin exposure to reactive chemicals reaches beyond antigen generation, triggering the additional events described above. Concomitant nature of these events may explain how sensitization occurs only when cellular defense systems are overwhelmed (). Developing assays to quantify certain events (e.g. detoxification, redox balance disturbance and generation of endogenous electrophiles, reversibility of covalent modifications) in addition to existing reactivity assays may help determine the true relationship between reactivity and sensitization potency of a chemical.
Figure 5. Simplified diagram showing how electrophilic chemicals may interact with cell defense systems, which act in concert to prevent a threshold for sensitization being reached. Electrophilic molecule is likely to encounter cell detoxification mechanisms (k4) faster than be able to covalently modify proteins (k1). It is likely that some type of covalent modifications can be reversed (k3) and subsequently removed by cellular detoxification mechanisms (k4). However, detoxification (k4) may lead to disturbance of redox balance and increase lipid peroxidation (LP, k2), which, in turn, also covalently modifies cellular proteins, potentially speeding up antigen processing and activating unfolded protein response (UPR).
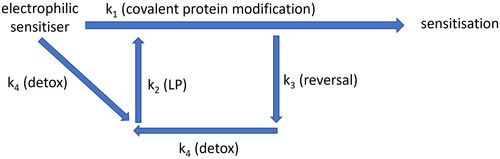
3.2. Epidermal inflammation
Keratinocytes and antigen presenting cells (APCs) are triggered via innate immune mechanisms during induction of skin sensitization (OECD Citation2014; Martin Citation2017), resulting in epidermal inflammation. Often referred to as “danger signal” (Ainscough et al. Citation2013), molecules released in response to sensitizer-induced cellular injury act as the link between the innate and the adaptive arms of the immune system. Current validated assays addressing KE2 have focused on the activation of the key inflammatory pathway in keratinocytes as a readout of the epidermal inflammation (KeratinoSensTM (Emter et al. Citation2010; Natsch and Emter Citation2016) and LuSens (Ramirez et al. Citation2014), included in the OECD TG 442D (OECD Citation2022a )). The assays rest on the principle that electrophiles modify Cys residues on Kelch-like ECH-associated protein (Keap 1) which leads to release of the Nrf2. Released Nrf2, no longer targeted for ubiquitination and proteosomal degradation, migrates to the nucleus and binds to the electrophile/antioxidant response element (EpRE/ARE), thus activating the transcription of cytoprotective genes, mainly encoding for metabolic phase II enzymes (Natsch and Emter Citation2016). Nrf2 pathway activation represents a cumulative effect of electrophilic sensitizer, ROS, GSH, and endogenous electrophiles (RCS), all of which react with Cys residues of Keap 1 (, f). Subject of much investigation, it has been proposed most recently that the potency of a chemical may be inversely proportional to the amount of Nrf2 protein available (Vallion and Kerdine-Römer Citation2022).
Besides Nrf2 pathway activation, epidermal inflammation in skin sensitization is a culmination of multiple molecular signals from both keratinocytes and APCs (Kimber et al. Citation2002, Citation2011). The entire set of molecular components of epidermal inflammation is not completely understood, but it is evident that a substantial overlap in the inflammatory signaling pathways exists in these two cell types. Recent advances in understanding inflammation in both keratinocytes and APCs as well as potential ways of utilizing this information are discussed below.
3.2.1. Cytokines in keratinocytes and APCs
Cytokine secretion is a hallmark of epidermal inflammation in response to skin sensitizer exposure and is regulated at both transcriptional and post translational levels. Increased serum levels of IL 18 and enhanced expression of IL 1β, IL 1Ra, IL 36a, IL 36b, IL 36c, and IL 33 in the involved skin of ACD patients have been observed (Mattii et al. Citation2013). Both human keratinocytes and APCs significantly increased the production of IL 1β, IL 18 as well as Interleukin Converting Enzyme (ICE) (Zepter et al. Citation1997; Matos et al. Citation2005). ICE or Caspase 1, which cleaves both IL 1β and IL 18 into their biologically active forms, is regulated by inflammasome in both keratinocytes and APCs (Naik et al. Citation1999; Mee et al. Citation2000). Pro-inflammatory cytokines also induced in both cell types include tumor necrosis factor alpha (TNF α) and IL 8 (Barker et al. Citation1991; Rambukkana et al. Citation1996; Nukada et al. Citation2008). Interestingly, the IL 8 assay, which is the only OECD validated cytokine assay (Watanabe et al. Citation2007; Sand et al. Citation2018) was developed as a marker for APC activation (KE3) rather than keratinocyte inflammation (KE2), possibly due to the cell type used in the assay (OECD Citation2023c).
3.2.2. Damage associated molecular patterns (DAMPs)
Both intracellular and extracellular danger signals contribute to the epidermal inflammation via cytokine regulation (Schaefer Citation2014). Upstream to the cytokine expression and processing, extracellular DAMPs such as Low Molecular Weight Hyaluronic Acid (LMWHA) fragments signal through the Toll-like Receptors (TLRs) to trigger inflammatory responses (Scheibner et al. Citation2006; Jiang et al. Citation2011). Electrophiles increase the degradation of High Molecular Weight HA (HMWHA) to LMWHA fragments by either modulating the expression of Hyaluronidase isoforms or Hyaluronan Synthase levels in human keratinocytes (Nikitovic et al. Citation2015). Electrophiles also upregulate TLR 4 and NFκB leading to further modulation of inflammatory cytokines, such as IL 18 and IL 8 (Galbiati et al. Citation2014; van der Veen et al. Citation2016; Kavasi et al. Citation2019). High Mobility Group Protein B1 (HMGB1), a non-histone chromatin binding protein, is an agonist of TLR 4 that is released from keratinocytes on exposure to electrophilic chemicals (Galbiati et al. Citation2014). LMWHA fragments have also been shown to activate the TLR 4 signaling pathway in DCs, including facilitating phosphorylation of p38/p42/44 MAP kinases and nuclear translocation of NFκB (Termeer et al. Citation2002). However, covalent modification of either IKK or p65 of NFκB by MA sensitizers mentioned earlier has been shown to inhibit this signaling pathway and have an anti-inflammatory effect (Natsch et al. Citation2011). Signaling through MAPK is another key component that regulates inflammation through various touch points in both keratinocytes and DCs (Trompezinski et al. Citation2008).
Adenosine triphosphate (ATP) contributes to inflammation, but also to the maturation of APCs. Chemically injured keratinocytes release ATP and in the presence of extracellular ATP, APCs show a transient increase in endocytosis, up-regulation of CD86, CD54, and major histocompatibility complex (MHC) class II, secretion of IL 12 as well as exhibit an improved stimulatory capacity for allogeneic T cells (Schnurr et al. Citation2000; Mizumoto et al. Citation2003).
Both keratinocytes and APCs elevate ROS upon exposure to skin sensitizers (Bruchhausen et al. Citation2003; Mehrotra et al. Citation2005; Martin et al. Citation2011). This can lead to the activation of inflammasome and release of active IL 1β and IL 18 in keratinocytes (Galbiati et al. Citation2014). ROS-induced oxidative breakdown of the extracellular matrix results in generation LMWHA, which activate the TLR and NFκB signaling cascade (Martin et al. Citation2011). As discussed earlier, ROS additionally activates the Nrf2 pathway, which mounts an antioxidant response. Nrf2 pathway cross talks with the NFκB and inflammasome signaling which contributes to the dynamics of pro and anti-inflammatory effects (Ade et al. Citation2009; Helou et al. Citation2019). ROS are generated during mitochondrial oxidative metabolism, which implies a key mitochondrial function in the sensitizer-induced inflammatory response and a further burden to the cellular detoxification systems.
Additional protective systems, upregulated in response to protein damage by electrophiles, have not received much attention (e.g. heat shock inducible factor (HSF1) and hypoxia inducible transcription factor (HIF1α), controlling heat shock and hypoxia responses, respectively (Cyran and Zhitkovich Citation2022)). A recent study has suggested a specific mechanistic role for the heat shock protein 90 (Hsp90) in sensitization to DNCB, identifying several DNCB modified Hsp90 peptide fragments (Kim et al. Citation2022).
3.3.3. Biomarker signatures
Genes downstream of the Nrf2 pathway and other cellular stress markers have been evaluated as signatures for sensitizer-induced transcriptional changes in novel in vitro assays, such as SenCeeTox®, SENS-IS, EpiSensA, and VITOSENS (Hooyberghs et al. Citation2008; Lambrechts et al. Citation2010; McKim et al. Citation2012; Cottrez et al. Citation2015; Saito et al. Citation2017), as well as ex vivo skin (van der Veen et al. Citation2015). In addition to Nrf2 targets, the SENS-IS assay also considers select biomarkers with redox/detox functions within the set of ARE genes (24 biomarkers) and an additional set of 42 genes with varied functionalities (apoptosis, cell death, heat shock, cell stress, inflammation, MHC, proteasome, and tissue repair pathways). Specific criteria applied to a combination of gene expression from both sets is used to classify sensitizers of varying potency. In MUTZ3 CD34+ DC progenitors treated with sensitizers, Nrf2 and ARE genes were found to be part of the predictive gene signature (Johansson et al. Citation2011). This has been explored further through the development of GARDTMskin assay platform and indicates the role of genes related to metabolism and cell cycle in addition to oxidative stress response (Albrekt et al. Citation2014; Zeller et al. Citation2017; Johansson et al. Citation2019; Gradin et al. Citation2021).
3.3.4. Combining multiple inflammatory signals from keratinocytes and APCs
It is difficult to envisage how any measurement of single components of the inflammation process in keratinocytes and/or APCs could provide a useful readout for improving our ability to predict potency of sensitizers. Given the numerous signaling pathways and markers characteristic of the sensitizer-induced inflammatory response and the substantial overlap of these cascades between keratinocytes and APCs, there is ample opportunity to explore how combined inflammatory parameters may influence sensitizer potency. Additionally, understanding how reactivity may modulate inflammation response (directly (e.g. MA sensitizers inhibiting NFkB signaling) or by proxy (increase in ROS and initiation of LP, forming RCS)) may be vital in improving our ability to predict sensitizing potency. A potential approach to quantitatively combine key inflammatory signals from keratinocytes and APCs is suggested in .
Figure 6. (A) A Snapshot of unique and overlapping signaling components of keratinocytes (KCs) and dendritic cells (DCs) (B) Schematic showing possible dose-dependent interplay and additive effects of inflammatory signals from KCs and DCs feeding into different levels of potency of sensitizing compounds.
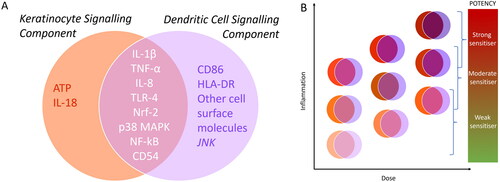
3.4. Keratinocyte-immune cells communication
In addition to the unique and overlapping components of signaling that takes place within keratinocytes and DCs, there is evidence of “cell-to-cell” communication that generally takes place in the epidermis as well as specifically during allergic sensitization. This intercellular communication takes place via a variety of mechanisms.
3.4.1. Exosomes
Exosomes have been shown to aid intercellular communication by transporting key information in the form of mRNA, miRNA, DNA and proteins (Bang and Thum Citation2012; Jella et al. Citation2018) as well as mitochondria (Hough et al. Citation2018). Exosomes from murine keratinocyte cell line MPEK were readily taken up by bone marrow-derived DC in vitro resulting in a matured phenotype, accompanied by increased CD40 expression as well as by the production of large amounts of IL 6, IL 10, and IL 12 (Kotzerke et al. Citation2013). HaCaT cells can induce T cell proliferation via indirect contact through exosomes that contain major histocompatibility complex (MHC) I and II (Cai et al. Citation2017). In addition to cells using a systemic transit of exosome-like nanovesicles to deliver a chosen inhibitory miRNA to target effector T cells in a contact hypersensitivity model, functional cell targeting by free extracellular RNA has also been shown to take place by transfecting companion cell exosomes that then transfer RNA cargo to the acceptor cells (Bryniarski et al. Citation2013; Citation2015). Such mechanisms may play a role in modulating cellular/tissue homeostasis and the host immune system in allergic diseases (Hough and Deshane Citation2019).
3.4.2. Nanotubes
Su and Igyártó demonstrated that epidermal Langerhans cells (LCs) contained many keratinocyte specific molecules (keratins, adhesion molecules, mRNA and protein) and were more prone to migration. These keratinocyte-specific signatures were transferred from keratinocytes to LCs through an exosome independent mechanism that likely involved tunneling nanotubules and was not unidirectional (Su and Igyártó Citation2019). Nanotubular structures have been shown to facilitate the selective transfer of membrane vesicles and organelles, thereby serving as a key mechanism of intercellular transfer of organelles (Rustom et al. Citation2004). Additionally, nanotubules enable myeloid cells to communicate with both targeted neighboring or distant cell, as well as other cell types, therefore, creating a complex variety of cellular exchanges, including pathogen spread (Dupont et al. Citation2018).
3.4.3. Blebs
Mentioned previously, Bauer et al. demonstrated a keratinocyte mechanism where neoepitopes (covalently modified proteins generated upon bromobimanes exposure) are released via keratinocyte blebbing. This form of cellular communication is likely the main source of haptenated protein for the APCs which engulf and process the material contained within the blebs (Bauer et al. Citation2011).
Although potentially interesting for understanding the mechanistically complete picture of induction of sensitization, it is unclear how quantifying the various means of “cell-to-cell” communication may be informative in estimating sensitization potency.
3.5. Antigen presenting cells activation, migration, and maturation
The activation, migration and overall maturation of DCs are the hallmarks of the KE3 of the skin sensitization AOP. The bridging event between the innate and adaptive immunity results from a cascade of several upstream cellular processes. Apart from inflammatory response, LCs undergo numerous additional changes. These include internalization of MHC Class II molecules into endosomal compartments (for loading of the haptenated peptides on to the MHC II molecules (Girolomoni et al. Citation1990; Becker et al. Citation1992)), followed by increased tyrosine phosphorylation (Kühn et al. Citation1998), increase in the expression of surface markers such as CD86 and CD54, stabilization of the HLA-DR molecules on the surface, and downregulation of cell adhesion molecules (Aiba et al. Citation1997) resulting in their migration to the local lymph nodes. While the MHC restricted antigen presentation is accepted as the main antigen presentation pathway for skin sensitization, there is emerging evidence that CD1 presentation, involving skin lipids, may also have a role to play (Betts et al. Citation2017).
Validated assays using human DC-like cell lines or monocytic cell lines that can be differentiated into DC are hCLAT (Ashikaga et al. Citation2006), USENS™(Piroird et al. Citation2015), IL 8 Luc assay (Takahashi et al. Citation2011) and GARD™skin (Johansson et al. Citation2011, Citation2013), all included in the OECD TG 442E (OECD Citation2023c). While read outs from all these assays have been aligned to KE3, some of these assays are also representative of the events leading up to KE3. New knowledge related to DC maturation and a mechanistic basis to integrate inputs from the various signaling cascades, cellular processes as well as different dendritic and other immune cell populations are discussed below.
3.5.1. Multiple signaling pathways
There is substantial evidence pointing to oxidative stress and MAP Kinase signaling as key intracellular triggers leading to activation of DCs (Mizuashi et al. Citation2005; Nakahara et al. Citation2006; Trompezinski et al. Citation2008). Further genomic evidence, as seen in the GARD™skin hazard prediction signature, shows that numerous genes with known functions underpin the biology of cellular and molecular events that occur within DCs on sensitizer exposure (e.g. small molecule biochemistry, cell death, lipid metabolism, hematological system development, cell cycle, molecular transport, cellular growth and proliferation, carbohydrate metabolism, canonical pathways (e.g. Nrf2 mediated oxidative response), xenobiotic metabolism signaling, protein ubiquitination pathway, lipopolysaccharide (LPS)/IL 1 mediated inhibition of retinoid X receptor (RXR) function, aryl hydrocarbon receptor signaling and protein kinase A signaling pathways) (Johansson et al. Citation2011). Genomic signatures indicate that metabolic processes, cell cycling and oxidative stress responses are engaged differently depending on the reactivity mechanisms or potency of the sensitizer, as well as showing regulation of specific short, endogenous noncoding RNA molecules, miRNAs (Albrekt et al. Citation2014; Zeller et al. Citation2017). Number and type of regulated miRNAs appears to rely on individual structural and/or reactivity properties of chemical sensitizers (Lindberg et al. Citation2019). Furthermore, new insights have emerged from these investigations about proteins involved in cholesterol biosynthesis, homeostasis and autophagy (Lindberg et al. Citation2020; de Ávila et al. Citation2022, Citation2023b). Although not all mentioned pathways are biomarkers for DC activation, as some precede the KE3, consideration of these changes together with the commonly quantified DC activation markers in an integrated manner can add more understanding to the mechanistic basis of varying levels of potency amongst skin sensitizers.
3.5.2. Antigen presenting cell (APC) subtypes
The human skin is resident to several subtypes of APCs of which the epidermal LCs preferentially prime and expand the CD8+ or cytotoxic T lymphocytes (CTLs) (Kissenpfennig et al. Citation2005; Klechevsky et al. Citation2008; Furio et al. Citation2010). Dermal DCs are also capable of presenting antigens to T cells, albeit not as efficiently as LCs in inducing the CTLs into potent effectors (Kaplan et al. Citation2008; Klechevsky et al. Citation2008). There is also evidence of recruitment of blood-borne Langerin + DC precursor cells to the dermis, dependent on chemokine ligands such as C-C chemokine receptor 2 (CCR2) and Selectins expressed in vascular endothelial cells (Ginhoux et al. Citation2007; Merad et al. Citation2008). Thus, not only epidermal LCs, but DCs of dermal and vascular origin, are also capable of antigen presentation and priming of T cells. It is, however, unclear which factors determine the recruitment and involvement of additional DCs.
3.5.3. Changes in cell adhesion and migration
A key aspect of LCs activation is their migration to the draining lymph nodes, for which alteration of inter-cellular adhesion within the epidermis is important (Griffiths and Nickoloff Citation1989; Barker et al. Citation1991; Groves et al. Citation1995). E-cadherin mediates the adhesion between keratinocytes and LCs (Blauvelt et al. Citation1995; Mayumi et al. Citation2013) and yet, there is conflicting evidence whether it is indispensable in LC activation and migration to the draining lymph nodes (Jakob and Udey Citation1998; Brand et al. Citation2020). Whether other cell adhesion molecules such as integrins, selectins or immunoglobulins, some of which are expressed in the epidermis (D’Arcy and Kiel Citation2021), play a role in regulating migration remains unclear and may be worthy of investigation.
APC activation, maturation and migration rightly remain a vibrant area of research, however, it is difficult to envisage utility of, thus far overlooked, indicators of these events in the context of sensitizer potency.
3.6. Cellular immune response
As described in the skin sensitization AOP, the development of ACD is a biphasic event consisting of an induction phase characterized by priming of antigen specific T cells and an elicitation phase characterized by clinical symptoms such as erythema, itching and burning (). T cell proliferation, clonal expansion of primed antigen specific T cells, is described in the KE4 of the skin sensitization AOP. This KE is the hallmark of the delayed type hypersensitivity, a point at which the individual is sensitized to a chemical, although involvement of the B cells in skin sensitization has also been investigated.
3.6.1. T cell proliferation
Currently, the only validated assay measuring T cell proliferation resulting from the induction of skin sensitization is the local lymph node assay (LLNA, (OECD Citation2010a)). However, animal data can no longer be generated for the purpose of cosmetic regulation in the European Union (EU Citation2009). While there may, indeed, be opportunities to explore development of informative assays with this output (Kimber et al. Citation2012; van Vliet et al. Citation2018), currently there are no NAMs addressing the activation and proliferation of a T cell response which are sufficiently progressed for implementation in OECD TG or for use in a NGRA approach (van Vliet et al. Citation2018). It remains difficult to envisage robust, sensitive, and reproducible assays addressing this KE in vitro in the near future.
3.6.2. B cell involvement
Historically, there have been attempts to investigate the level of involvement of B cells in pathophysiology of ACD. This is still an area generating considerable interest. Both LCs and dermal DCs have the ability to polarize naïve CD4+ T cells either to secrete Th2 cytokines or differentiate into T cells that can induce naive B cells to secrete large amounts of immunoglobulins (Igs) (Klechevsky et al. Citation2008). If B cells are presented with specific IgE to a hapten-protein complex together with the specific antigen, they have been shown to internalize the IgE-antigen complex via a CD23 mediated mechanism and cross-present the antigen to DCs in vitro (Engeroff et al. Citation2018). The involvement of B cells could potentially modulate the efficiency with which DCs present the antigens to T cells leading to immunological priming and generation of memory T cells. A relatively recent review indicates that, despite technical difficulties, more investigation, particularly in humans, is required into B cell activation and proliferation as well as hapten specific antibody production (Singleton et al. Citation2016). This represents a unique link to respiratory sensitization, where B cell response is dominant (Sullivan et al. Citation2017). The sparse knowledge of the nature of hapten protein conjugate is perhaps what hampers efforts in understanding the B cell and hapten specific antibody response in skin sensitization. The relative strength of the B cell response may be related to the type of antigen generated during KE 1. For example, certain skin sensitizers are capable of crosslinking proteins or otherwise generating “larger” antigens (e.g. p-phenylenediamine (PPD), glutaraldehyde, formaldehyde). Such complex antigens are likely to be less transient, not readily reversed, more persistent and thus, perhaps more likely to be recognized by an anti-hapten antibody. This warrants further investigation as antibodies could influence the thresholds in induction of skin sensitization.
3.7. Adverse outcome
ACD, characterized by clinical symptoms ranging from mild (such as erythema, itching and burning) to severe (blistering, severe edema and cracking of the skin), is a common, widespread ailment which can become chronic and disabling, causing a public health issue (Uter et al. Citation2020). In recent studies it has been estimated that over 20% of the general population have acquired contact allergy to at least one environmental allergen (Alinaghi et al. Citation2019; Scheinman et al. Citation2021). ACD is diagnosed by specialist dermatological investigations involving patch testing to identify the allergen to which an individual is allergic and enable allergen avoidance. In addition, the clinical patch test information can be used to detect emerging issues and identify predisposing factors for development of ACD (Schwensen et al. Citation2015). A number of opportunities may exist to make better use of the clinical evidence and experience within the safety assessment of contact allergens (Gilmour et al. Citation2019). Recently, clinical experience and established trends in contact allergy have been used within the SARA model DA to enable a prediction of the risk of induction of skin sensitization to a given exposure arising from a consumer product (Reynolds et al. Citation2022). Further utility of the clinical data and evidence could be found in understanding the sources of interindividual and/or population variability in susceptibility to sensitization. Understanding differences in individual phase II metabolizing enzymes may shed a light on differences in susceptibility to sensitization by chemicals ultimately removed by those enzymes (Sánchez-Gómez et al. Citation2016). For example, NAT1 genotypes containing a rapid acetylator NAT1*10 allele were potentially associated with reduced susceptibility to PPD sensitization (Blömeke et al. Citation2009). Similarly, differences in epidermal PUFA make-up may help understand individual susceptibility to sensitization. These questions can potentially be answered by studying key molecular components on a population level.
4. Discussion and concluding remarks
When trying to understand the sensitizing potential of chemicals and make risk assessment decisions, particularly for those molecules for which the current tools provide limited or conflicting information, there is considerable value in further mechanistic characterization of the molecular events in skin sensitization. Quantitative information about certain molecular events or models utilizing multiple quantitative outputs, discussed above, could be instrumental in this endeavor.
The most promising area for thorough exploration remains chemical reactivity of sensitizers. Natsch and Emter (Citation2017) made a strong case for better utilization of knowledge of chemical reactivity of sensitizers and made very useful suggestions on what future assays determining reactivity may look like. This study served as a catalyst for the current review of the AOP and strengthened the resolve to keep the mechanistic knowledge up to date and useful for non-animal risk assessment of skin sensitization potential and potency. The authors noted that the emphasis in the development of new assays for skin sensitization was almost exclusively placed on the biological assays and that this bias is not mechanistically fully justified.
Cellular reaction following exposure to an electrophile is a dynamic process during which an electrophilic chemical interacts with several components of the cell defense systems, which work in concert to prevent protein damage and, in this case, induction of sensitization. It is, therefore, likely that induction of sensitization occurs only once these defense systems are overwhelmed. While the defense mechanisms of cells are unlikely to change, they will differ for different chemicals, depending on their electrophilicity and reaction mechanism to protein and other cellular nucleophiles. Following on from this, quantitatively studying ways of how cell defense mechanisms are orchestrated together to prevent sensitization is the most promising path to explore and expand our understanding. Quantitative understanding of relevant components of cell defense may ultimately allow us to consider sensitizer potency across all mechanistic domains.
While the MIE in sensitization is now better understood, not all reactivity relevant events which have the potential to influence sensitization threshold are currently always considered in risk assessment. Questions remain about the quantitative impact of oxidative stress and LP on induction of sensitization. It is further unclear if and how the RCS impact the kinetics of antigen generation and whether PUFA make-up of individual’s keratinocytes may be influencing their susceptibility to sensitization. Furthermore, as skin lipids are proportionally the highest constituents of human skin (Knox and O’Boyle Citation2021) and evidence is emerging of their involvement in an alternative antigen presentation pathway (Betts et al. Citation2017), it is prudent to investigate further how skin lipids may contribute to induction of sensitization. Another phenomenon which has not received much attention is the stability or reversibility of covalent protein adducts and what impact this may have on antigen formation. Both LP (and, therefore, levels of RCS) and cellular capacity to reverse covalent modification are likely to be stable in models and could potentially be determined, at least for the best known mechanisms. However, they may differ qualitatively and quantitatively between individuals and populations.
The repertoire of reactivity-driven events secondary to antigen formation is often regarded as part of inflammatory response and is, traditionally, considered in KE2. These events occur as a direct consequence of electrophile reactivity, either prior to or concomitantly with MIE in sensitization. It is, therefore, vital to understand their quantitative relevance to antigen formation. The established concept of direct relationship between chemical reactivity and sensitizing potency could be deepened by expanding the quantitation of reactivity from a solely “antigen generating” event to a more holistic “interaction with epidermal cells.” This encompasses detoxification, redox balance disturbance, initiation of LP, and reversibility of covalent adducts. It is possible that several of these elements could influence the potency of a sensitizer: (a) electrophilic reactivity of the chemical (including the complexity and chemoselectivity), (b) reversibility of covalent adducts formed with skin proteins, (c) type and capacity of detoxification, (d) level of redox imbalance and immediate oxidative stress induced and (e) amount of LP (and, therefore, RCS). A very potent sensitizer should be highly reactive with multiple nucleophiles and/or have complex reactivity mechanism (making adducts in several steps and/or crosslinking proteins), generate less readily reversible adducts, be subject to an exhaustible mode of detoxification and generate rapid and extensive redox balance disturbance, resulting in immediate effect on proteins and lipids, in turn generating large amount of RCS. Conversely, sensitizer at the weak end of potency spectrum should be less reactive with fewer nucleophile types, have simpler reactivity mechanism without ability to cross link, make readily reversible adducts, be subject to effective and less exhaustible (or multiple) modes of detoxification and generate less redox balance disturbance and lipid peroxidation and, therefore, less RCS.
It is plausible that simple, in chemico assays could be developed (or adapted) for detoxification mechanisms currently best understood. The sensitivity and specificity of analytical techniques is continually improving and it is likely that phenomena such as LP and reversibility mechanisms/rates could be quantitatively studied in this context using omics approaches (such as HR MAS NMR metabolomics (e.g. Moussallieh et al. Citation2020) or adapting existing proteomic methods (e.g. Lu et al. Citation2022). Utilization of the quantitative data of KEs and additional elements of AOPs may ultimately be achieved through quantitative AOP models (qAOP) (Zgheib et al. Citation2019; Spinu et al. Citation2020), which may help us avoid the linear interpolation of the quantitative outputs of the KEs in the AOP and adopt a more mechanistically relevant interpolation. Emphasis should be firmly on quantifying highly informative parts of the AOP in such models, rather than attempting to fully quantify the entire set of KEs. While the current approaches for skin sensitization risk assessment of chemicals serve us well, the ability to derive more mechanistic information on chemicals that have been identified as sensitizers may ultimately allow more informed decisions on a chemical-specific basis.
Finally, besides skin sensitization, electrophilic chemicals are implicated in other pathologies. Numerous examples exist of toxicity of electrophiles (or their metabolites), ranging from respiratory sensitization (Cochrane et al. Citation2015; Ferreira et al. Citation2018), systemic toxicity of certain antibiotics, anticonvulsants and antiretrovirals (e.g. Evans et al. Citation2004; Zhou et al. Citation2005; Hernandez-Jaimes et al. Citation2022), specific organ toxicities (liver and kidney) caused by non-steroidal anti-inflammatory drugs (NSAIDs) (e.g. acetaminophen (Bessems and Vermeulen Citation2001)) to mixed modes of toxicity. Some of the phenomena discussed above, including ways to utilize the assays with quantitative output should ultimately be applicable to variety of exposure scenarios to electrophilic chemicals or electrophilic metabolites.
Abbreviations | ||
AOP | = | Adverse Outcome Pathway |
OECD | = | The Organisation for Economic Co-operation and Development |
DA | = | defined approach |
SARA model | = | Skin Allergy Risk Assessment model |
MIE | = | molecular initiating event |
KE | = | key event |
ACD | = | allergic contact dermatitis |
NAM | = | new approach methodology |
TG | = | test guideline |
DPRA | = | Direct Peptide Reactivity Assay |
ADRA | = | Amino acid Derivative Reactivity Assay |
kDPRA | = | kinetic Direct Peptide Reactivity Assay |
h-CLAT | = | Human Cell Line Activation Test |
GARD | = | genomic allergen rapid detection |
UN GHS | = | United Nations Globally Harmonized System |
NICEATM | = | National Toxicology Program Interagency Center for the Evaluation of Alternative Toxicological Methods |
ITS | = | integrated testing strategy |
NGRA | = | Next Generation Risk Assessment |
DNCB | = | 2,4-dinitro-1-chlorobenzene |
MA | = | Michael acceptor |
SB | = | Schiff Base |
K5: K14: K1: K10 | = | keratin 5: keratin 14: keratin 1: keratin 10 |
Cys | = | cysteine |
TRITC | = | tetramethylrhodamine isothiocyanate |
MIF | = | migration inhibitory factor |
NFκB | = | nuclear factor-kappa B |
4-HNE | = | 4-hydroxy-2-nonenal |
RCS | = | reactive carbonyl species |
IKK | = | IκB kinase |
SN2 | = | bimolecular nucleophilic substitution |
SNAr | = | nucleophilic substitution on an aromatic center |
HR-MAS NMR | = | high-resolution magic angle spinning nuclear magnetic resonance |
HSA | = | human serum albumin |
RHE | = | reconstituted human epidermis |
His | = | histidine |
Met | = | methionine |
MI | = | 2-methylisothiazolin-3-one |
MCI | = | 5-chloro-2-methylsothiazolin-3-one |
GSH | = | glutathione |
GST | = | glutathione-s-transferase |
ROS | = | reactive oxygen species |
LP | = | lipid peroxidation |
PPRA | = | Peroxide-Peroxidase Reactivity Assay |
CYP | = | cytochrome p450 |
AO/ALDH | = | aldehyde oxidases/dehydrogenase |
NQR | = | NAD(P)H quinone reductase |
EH | = | epoxide hydrolase |
UGTs | = | UDP-glucuronosyl transferases |
SULTs | = | sulfotransferases |
NATs | = | N-acetyl transferases |
MDBGN | = | 2-bromo-2-(bromomethyl)glutaronitrile (methyldibromoglutaronitrile) |
PUFA | = | polyunsaturated fatty acid |
Nrf2 | = | nuclear factor erythroid 2-related factor 2 |
ER | = | endoplasmic reticulum |
UPR | = | unfolded protein response |
DNFB | = | 2,4-dinitro-1-fluorobenzene |
LA | = | lineloic acid |
AA | = | arachidonic acid |
MDA | = | malondialdehyde |
Lys | = | lysine |
TBA | = | thiobarbituric acid |
Trx | = | thioredoxin |
TrxR | = | thioredoxin reductase |
APC | = | antigen presenting cell |
Keap1 | = | Kelch-like ECH-associated protein |
EpRE/ARE | = | electrophile/antioxidant response element |
IL | = | interleukin |
ICE | = | interleukin converting enzyme |
TNF-α | = | tumor necrosis factor alpha |
DAMPs | = | damage-associated molecular patterns |
LMWHA | = | low molecular weight hyaluronic acid |
TLR | = | toll-like receptor |
DC | = | dendritic cell |
CD | = | cluster of differentiation |
ATP | = | adenosine triphosphate |
MHC | = | major histocompatibility complex |
HSF1 | = | heat shock inducible factor 1 |
HIF1α | = | hypoxia-inducible transcription factor 1 alpha |
Hsp90 | = | heat shock protein 90 |
LC | = | Langerhans cell |
HLA-DR | = | human leukocyte antigen – DR isotype |
LPS | = | lipopolysaccharide |
RXR | = | Retinoid X Receptor |
LLNA | = | Local Lymph Node Assay |
Ig | = | immunoglobulin |
PPD | = | p-phenylenediamine. |
Acknowledgments
The authors wish to thank Gavin Maxwell of Safety and Environmental Assurance Centre, Unilever for helpful suggestions and encouragement during the early stages of the manuscript preparation. Our thanks also go to Georgia Reynolds, Maria Baltazar and Carl Westmoreland (all of Safety and Environmental Assurance Centre, Unilever) for their thorough critical review of the manuscript and helpful suggestions ahead of submission. We are very grateful to Eoin Winston and Frank Munnelly of NEXU Creative for their detailed and masterful effort on the images for and . We would also like to express our gratitude for their thoughtful and detailed comments and suggestions to the reviewers of the manuscript who were selected by the Editor and whose identity is unknown to the authors.
Declaration of interest
The submitted manuscript was inspired by the publication by Natsch and Emter in 2017 (Natsch and Emter Citation2017). MA conceived the original idea, which was further developed in many discussions with RR, NG, SS and RA with a view to review the most up to date mechanistic insights into induction of sensitization in humans. Our motivation is to emphasize the most promising areas of research suitable for development of novel assays with capacity to determine sensitizing potency and aid risk assessment decisions. The authors are employees of Safety and Environmental Assurance Centre, Unilever and no external funds were used in the preparation of this manuscript. The authors do not imply competing financial interests or personal relationships that could have appeared to influence the work reported in this manuscript.
References
- Ade N, Leon F, Pallardy M, Peiffer JL, Kerdine-Romer S, Tissier MH, Bonnet PA, Fabre I, Ourlin JC. 2009. HMOX1 and NQO1 genes are upregulated in response to contact sensitizers in dendritic cells and THP-1 cell line: role of the Keap1/Nrf2 pathway. Toxicol Sci. 107(2):451–460. doi: 10.1093/toxsci/kfn243.
- Aiba S, Terunuma A, Manome H, Tagami H. 1997. Dendritic cells differently respond to haptens and irritants by their production of cytokines and expression of co-stimulatory molecules. Eur J Immunol. 27(11):3031–3038. doi: 10.1002/eji.1830271141.
- Ainscough JS, Frank Gerberick G, Dearman RJ, Kimber I. 2013. Danger, intracellular signaling, and the orchestration of dendritic cell function in skin sensitization. J Immunotoxicol. 10(3):223–234. doi: 10.3109/1547691X.2012.711782.
- Albrekt A-S, Johansson H, Börje A, Borrebaeck C, Lindstedt M. 2014. Skin sensitizers differentially regulate signaling pathways in MUTZ-3 cells in relation to their individual potency. BMC Pharmacol Toxicol. 15(1):5. doi: 10.1186/2050-6511-15-5.
- Aleksic M, Thain E, Roger D, Saib O, Davies M, Li J, Aptula A, Zazzeroni R. 2009. Reactivity profiling: covalent modification of single nucleophile peptides for skin sensitization risk assessment. Toxicol Sci. 108(2):401–411. doi: 10.1093/toxsci/kfp030.
- Alépée N, Tourneix F, Singh A, Ade N, Grégoire S. 2023. Off to a good start? Review of the predictivity of reactivity methods modelling the molecular initiating event of skin sensitization. ALTEX. 40(4):606–618. doi: 10.14573/altex.2212201.
- Alinaghi F, Bennike NH, Egeberg A, Thyssen JP, Johansen JD. 2019. Prevalence of contact allergy in the general population: a systematic review and meta-analysis. Contact Dermatitis. 80(2):77–85. doi: 10.1111/cod.13119.
- Alvarez-Sánchez R, Basketter D, Pease C, Lepoittevin JP. 2004a. Covalent binding of the 13C-labeled skin sensitizers 5-chloro-2-methylisothiazol-3-one (MCI) and 2-methylisothiazol-3-one (MI) to a model peptide and glutathione. Bioorg Med Chem Lett. 14(2):365–368. doi: 10.1016/j.bmcl.2003.11.002.
- Alvarez-Sánchez R, Divkovic M, Basketter D, Pease C, Panico M, Dell A, Morris H, Lepoittevin J-P. 2004b. Effect of glutathione on the covalent binding of the (13)C-labeled skin sensitizer 5-chloro-2-methylisothiazol-3-one to human serum albumin: identification of adducts by nuclear magnetic resonance, matrix-assisted laser desorption/ionization mass spectrometry, and nanoelectrospray tandem mass spectrometry. Chem Res Toxicol. 17(9):1280–1288. doi: 10.1021/tx049935+.
- Anders MW. 2008. Chemical toxicology of reactive intermediates formed by the glutathione-dependent bioactivation of halogen-containing compounds. Chem Res Toxicol. 21(1):145–159. doi: 10.1021/tx700202w.
- Ankley GT, Bennett RS, Erickson RJ, Hoff DJ, Hornung MW, Johnson RD, Mount DR, Nichols JW, Russom CL, Schmieder PK, et al. 2010. Adverse outcome pathways: a conceptual framework to support ecotoxicology research and risk assessment. Environ Toxicol Chem. 29(3):730–741. doi: 10.1002/etc.34.
- Ashikaga T, Yoshida Y, Hirota M, Yoneyama K, Itagaki H, Sakaguchi H, Miyazawa M, Ito Y, Suzuki H, Toyoda H. 2006. Development of an in vitro skin sensitization test using human cell lines: the human cell line activation test (h-CLAT). I. Optimization of the h-CLAT protocol. Toxicol In Vitro. 20(5):767–773. doi: 10.1016/j.tiv.2005.10.012.
- Ayala A, Muñoz MF, Argüelles S. 2014. Lipid peroxidation: production, metabolism, and signaling mechanisms of malondialdehyde and 4-hydroxy-2-nonenal. Oxid Med Cell Longev. 2014:360438. doi: 10.1155/2014/360438.
- Bailey A, Nicholas B, Darley R, Parkinson E, Teo Y, Aleksic M, Maxwell G, Elliott T, Ardern-Jones M, Skipp P. 2021. Characterization of the Class I MHC peptidome resulting from DNCB exposure of HaCaT cells. Toxicol Sci. 180(1):136–147. doi: 10.1093/toxsci/kfaa184.
- Baillie TA, Kassahun K. 1994. Reversibility in glutathione-conjugate formation. In: Anders MW, Dekant W, editors. Advances in pharmacology. Cambridge (MA): Academic Press; p. 163–181.
- Bang C, Thum T. 2012. Exosomes: new players in cell–cell communication. Int J Biochem Cell Biol. 44(11):2060–2064. doi: 10.1016/j.biocel.2012.08.007.
- Barker JN, Mitra RS, Griffiths CE, Dixit VM, Nickoloff BJ. 1991. Keratinocytes as initiators of inflammation. Lancet. 337(8735):211–214. doi: 10.1016/0140-6736(91)92168-2.
- Basketter DA, Alépée N, Ashikaga T, Barroso J, Gilmour N, Goebel C, Hibatallah J, Hoffmann S, Kern P, Martinozzi-Teissier S, et al. 2014. Categorization of chemicals according to their relative human skin sensitizing potency. Dermatitis. 25(1):11–21. doi: 10.1097/DER.0000000000000003.
- Bauer B, Andersson SI, Stenfeldt AL, Simonsson C, Bergstroom J, Ericson MB, Jonsson CA, Broo KS. 2011. Modification and expulsion of keratins by human epidermal keratinocytes upon hapten exposure in vitro. Chem Res Toxicol. 24(5):737–743. doi: 10.1021/tx200030y.
- Becker D, Mohamadzadeh M, Reske K, Knop J. 1992. Increased level of intracellular MHC class II molecules in murine Langerhans cells following in vivo and in vitro administration of contact allergens. J Invest Dermatol. 99(5):545–549. doi: 10.1111/1523-1747.ep12667308.
- Bessems JG, Vermeulen NP. 2001. Paracetamol (acetaminophen)-induced toxicity: molecular and biochemical mechanisms, analogues and protective approaches. Crit Rev Toxicol. 31(1):55–138. doi: 10.1080/20014091111677.
- Betts RJ, Perkovic A, Mahapatra S, Del Bufalo A, Camara K, Howell AR, Martinozzi Teissier S, De Libero G, Mori L. 2017. Contact sensitizers trigger human CD1-autoreactive T-cell responses. Eur J Immunol. 47(7):1171–1180. doi: 10.1002/eji.201746939.
- Billman JH, Diesing AC. 1957. Reduction of Schiff bases with sodium borohydride. J Org Chem. 22(9):1068–1070. doi: 10.1021/jo01360a019.
- Blauvelt A, Katz SI, Udey MC. 1995. Human langerhans cells express E-cadherin. J Invest Dermatol. 104(2):293–296. doi: 10.1111/1523-1747.ep12612830.
- Blömeke B, Brans R, Coenraads PJ, Dickel H, Bruckner T, Hein DW, Heesen M, Merk HF, Kawakubo Y. 2009. Para-phenylenediamine and allergic sensitization: risk modification by N-acetyltransferase 1 and 2 genotypes. Br J Dermatol. 161(5):1130–1135. doi: 10.1111/j.1365-2133.2009.09352.x.
- Bowes JH, Cater CW. 1968. The interaction of aldehydes with collagen. Biochim Biophys Acta. 168(2):341–352. doi: 10.1016/0005-2795(68)90156-6.
- Brand A, Diener N, Zahner SP, Tripp C, Backer RA, Karram K, Jiang A, Mellman I, Stoitzner P, Clausen BE. 2020. E-Cadherin is dispensable to maintain langerhans cells in the epidermis. J Invest Dermatol. 140(1):132–142.e133. doi: 10.1016/j.jid.2019.06.132.
- Bruchhausen S, Zahn S, Valk E, Knop J, Becker D. 2003. Thiol antioxidants block the activation of antigen-presenting cells by contact sensitizers. J Invest Dermatol. 121(5):1039–1044. doi: 10.1046/j.1523-1747.2003.12510.x.
- Bryniarski K, Ptak W, Jayakumar A, Püllmann K, Caplan MJ, Chairoungdua A, Lu J, Adams BD, Sikora E, Nazimek K, et al. 2013. Antigen-specific, antibody-coated, exosome-like nanovesicles deliver suppressor T-cell microRNA-150 to effector T cells to inhibit contact sensitivity. J Allergy Clin Immunol. 132(1):170–181. doi: 10.1016/j.jaci.2013.04.048.
- Bryniarski K, Ptak W, Martin E, Nazimek K, Szczepanik M, Sanak M, Askenase PW. 2015. Free extracellular miRNA functionally targets cells by transfecting exosomes from their companion cells. PLoS One. 10(4):e0122991. doi: 10.1371/journal.pone.0122991.
- Buratti FM, Darney K, Vichi S, Turco L, Di Consiglio E, Lautz LS, Béchaux C, Dorne J-L, Testai E. 2021. Human variability in glutathione-S-transferase activities, tissue distribution and major polymorphic variants: meta-analysis and implication for chemical risk assessment. Toxicol Lett. 337:78–90. doi: 10.1016/j.toxlet.2020.11.007.
- Cai XW, Zhu R, Ran L, Li YQ, Huang K, Peng J, He W, Zhou CL, Wang RP. 2017. A novel non‑contact communication between human keratinocytes and T cells: exosomes derived from keratinocytes support superantigen‑induced proliferation of resting T cells. Mol Med Rep. 16(5):7032–7038. doi: 10.3892/mmr.2017.7492.
- Castro JP, Jung T, Grune T, Siems W. 2017. 4-Hydroxynonenal (HNE) modified proteins in metabolic diseases. Free Radic Biol Med. 111:309–315. doi: 10.1016/j.freeradbiomed.2016.10.497.
- Chipinda I, Ajibola RO, Morakinyo MK, Ruwona TB, Simoyi RH, Siegel PD. 2010. Rapid and simple kinetics screening assay for electrophilic dermal sensitizers using nitrobenzenethiol. Chem Res Toxicol. 23(5):918–925. doi: 10.1021/tx100003w.
- Chipinda I, Mbiya W, Adigun RA, Morakinyo MK, Law BF, Simoyi RH, Siegel PD. 2014. Pyridoxylamine reactivity kinetics as an amine based nucleophile for screening electrophilic dermal sensitizers. Toxicology. 315:102–109. doi: 10.1016/j.tox.2013.11.009.
- Cochrane SA, Arts JH, Ehnes C, Hindle S, Hollnagel HM, Poole A, Suto H, Kimber I. 2015. Thresholds in chemical respiratory sensitisation. Toxicology. 333:179–194. doi: 10.1016/j.tox.2015.04.010.
- Cooper AJL, Hanigan MH. 2018. 10.17 - Metabolism of glutathione S-conjugates: multiple pathways. In: McQueen CA, editor. Comprehensive toxicology. 3rd ed. Oxford: Elsevier; p. 363–406.
- Cooper G. 2000. Lysosomes. The cell: a molecular approach. 2nd ed. Sunderland, MA: Sinauer Associates.
- Cottrez F, Boitel E, Auriault C, Aeby P, Groux H. 2015. Genes specifically modulated in sensitized skins allow the detection of sensitizers in a reconstructed human skin model. Development of the SENS-IS assay. Toxicol In Vitro. 29(4):787–802. doi: 10.1016/j.tiv.2015.02.012.
- Cottrez F, Boitel E, Ourlin J-C, Peiffer J-L, Fabre I, Henaoui I-S, Mari B, Vallauri A, Paquet A, Barbry P, et al. 2016. SENS-IS, a 3D reconstituted epidermis based model for quantifying chemical sensitization potency: reproducibility and predictivity results from an inter-laboratory study. Toxicol In Vitro. 32:248–260. doi: 10.1016/j.tiv.2016.01.007.
- Cyran AM, Zhitkovich A. 2022. HIF1, HSF1, and NRF2: oxidant-responsive trio raising cellular defenses and engaging immune system. Chem Res Toxicol. 35(10):1690–1700. doi: 10.1021/acs.chemrestox.2c00131.
- D’Arcy C, Kiel C. 2021. Cell adhesion molecules in normal skin and melanoma. Biomolecules. 11(8):1213. doi: 10.3390/biom11081213.
- de Ávila RI, Aleksic M, Zhu B, Li J, Pendlington R, Campos Valadares M. 2023a. Non-animal approaches for photoallergenicity safety assessment: needs and perspectives for the toxicology for the 21st century. Regul Toxicol Pharmacol 145:105499. doi: 10.1016/j.yrtph.2023.105499.
- de Ávila RI, Carreira Santos S, Siino V, Levander F, Lindstedt M, Zeller KS. 2022. Adjuvants in fungicide formulations can be skin sensitizers and cause different types of cell stress responses. Toxicol Rep. 9:2030–2041. doi: 10.1016/j.toxrep.2022.11.004.
- de Ávila RI, Carreira Santos S, Siino V, Levander F, Lindstedt M, Zeller KS. 2023b. A proteomics dataset capturing myeloid cell responses upon cellular exposure to fungicides, adjuvants and fungicide formulations. Data Brief. 46:108878. doi: 10.1016/j.dib.2022.108878.
- Debeuckelaere C, Berl V, Elbayed K, Moussallieh FM, Namer IJ, Lepoittevin JP. 2015. Matrix effect of human reconstructed epidermis on the chemoselectivity of a skin sensitizing alpha-methylene-gamma-butyrolactone: consequences for the development of in chemico alternative methods. Chem Res Toxicol. 28(11):2192–2198. doi: 10.1021/acs.chemrestox.5b00363.
- Debeuckelaere C, Moussallieh F-M, Elbayed K, Namer I-J, Berl V, Giménez-Arnau E, Lepoittevin J-P. 2016. In situ chemical behaviour of methylisothiazolinone (MI) and methylchloroisothiazolinone (MCI) in reconstructed human epidermis: a new approach to the cross-reactivity issue. Contact Dermatitis. 74(3):159–167. doi: 10.1111/cod.12524.
- Domingues RM, Domingues P, Melo T, Pérez-Sala D, Reis A, Spickett CM. 2013. Lipoxidation adducts with peptides and proteins: deleterious modifications or signaling mechanisms? J Proteomics. 92:110–131. doi: 10.1016/j.jprot.2013.06.004.
- Dupont M, Souriant S, Lugo-Villarino G, Maridonneau-Parini I, Vérollet C. 2018. Tunneling nanotubes: intimate communication between myeloid cells. Front Immunol. 9:43. doi: 10.3389/fimmu.2018.00043.
- Eggink M, Wijtmans M, Ekkebus R, Lingeman H, de Esch IJ, Kool J, Niessen WM, Irth H. 2008. Development of a selective ESI-MS derivatization reagent: synthesis and optimization for the analysis of aldehydes in biological mixtures. Anal Chem. 80(23):9042–9051. doi: 10.1021/ac801429w.
- Elbayed K, Berl V, Debeuckelaere C, Moussallieh FM, Piotto M, Namer IJ, Lepoittevin JP. 2013. HR-MAS NMR spectroscopy of reconstructed human epidermis: potential for the in situ investigation of the chemical interactions between skin allergens and nucleophilic amino acids. Chem Res Toxicol. 26(1):136–145. doi: 10.1021/tx300428u.
- Emter R, Ellis G, Natsch A. 2010. Performance of a novel keratinocyte-based reporter cell line to screen skin sensitizers in vitro. Toxicol Appl Pharmacol. 245(3):281–290. doi: 10.1016/j.taap.2010.03.009.
- Engeroff P, Fellmann M, Yerly D, Bachmann MF, Vogel M. 2018. A novel recycling mechanism of native IgE-antigen complexes in human B cells facilitates transfer of antigen to dendritic cells for antigen presentation. J Allergy Clin Immunol. 142(2):557–568.e556. doi: 10.1016/j.jaci.2017.09.024.
- EU. 2009. Regulation (EC) No 1223/2009 of the European Parliament and of the Council of 30 November 2009 on cosmetic products. Off J Eur Union. 52(L342):59–209.
- Evans DC, Watt AP, Nicoll-Griffith DA, Baillie TA. 2004. Drug-protein adducts: an industry perspective on minimizing the potential for drug bioactivation in drug discovery and development. Chem Res Toxicol. 17(1):3–16. doi: 10.1021/tx034170b.
- Ezendam J, Braakhuis HM, Vandebriel RJ. 2016. State of the art in non-animal approaches for skin sensitization testing: from individual test methods towards testing strategies. Arch Toxicol. 90(12):2861–2883. doi: 10.1007/s00204-016-1842-4.
- Ferreira I, Silva A, Martins JD, Neves BM, Cruz MT. 2018. Nature and kinetics of redox imbalance triggered by respiratory and skin chemical sensitizers on the human monocytic cell line THP-1. Redox Biol. 16:75–86. doi: 10.1016/j.redox.2018.02.002.
- Fujita M, Yamamoto Y, Tahara H, Kasahara T, Jimbo Y, Hioki T. 2014. Development of a prediction method for skin sensitization using novel cysteine and lysine derivatives. J Pharmacol Toxicol Methods. 70(1):94–105. doi: 10.1016/j.vascn.2014.06.001.
- Fujita M, Yamamoto Y, Watanabe S, Sugawara T, Wakabayashi K, Tahara Y, Horie N, Fujimoto K, Kusakari K, Kurokawa Y, et al. 2019. Cause of and countermeasures for oxidation of the cysteine-derived reagent used in the amino acid derivative reactivity assay. J Appl Toxicol. 39(2):191–208. doi: 10.1002/jat.3707.
- Furio L, Briotet I, Journeaux A, Billard H, Péguet-Navarro J. 2010. Human langerhans cells are more efficient than CD14(-)CD1c(+) dermal dendritic cells at priming naive CD4(+) T cells. J Invest Dermatol. 130(5):1345–1354. doi: 10.1038/jid.2009.424.
- Galbiati V, Bianchi S, Martínez V, Mitjans M, Corsini E. 2014. NCTC 2544 and IL-18 production: a tool for the in vitro identification of photoallergens. Toxicol in Vitro. 28(1):13–17. doi: 10.1016/j.tiv.2013.06.008.
- García-Piñeres AJ, Castro V, Mora G, Schmidt TJ, Strunck E, Pahl HL, Merfort I. 2001. Cysteine 38 in p65/NF-kappaB plays a crucial role in DNA binding inhibition by sesquiterpene lactones. J Biol Chem. 276(43):39713–39720. doi: 10.1074/jbc.M101985200.
- Gerberick F, Troutman JA, Foertsch LM, Vassallo JD, Quijano M, Dobson RLM, Goebel C, Lepoittevin JP. 2009. Ivestigation of peptide reactivity of pro-hapten skin sensitizers using a peroxidase-peroxide oxidation system. Toxicol Sci. 112(1):164–174. doi: 10.1093/toxsci/kfp192.
- Gerberick GF, Ryan CA, Kern PS, Schlatter H, Dearman RJ, Kimber I, Patlewicz GY, Basketter DA. 2005. Compilation of historical local lymph node data for evaluation of skin sensitization alternative methods. Dermatitis. 16(4):157–202.
- Gerberick GF, Vassallo JD, Bailey RE, Chaney JG, Morrall SW, Lepoittevin JP. 2004. Development of a peptide reactivity assay for screening contact allergens. Toxicol Sci. 81(2):332–343. doi: 10.1093/toxsci/kfh213.
- Gerberick GF, Vassallo JD, Foertsch LM, Price BB, Chaney JG, Lepoittevin JP. 2007. Quantification of chemical peptide reactivity for screening contact allergens: a classification tree model approach. Toxicol Sci. 97(2):417–427. doi: 10.1093/toxsci/kfm064.
- Gilmour N, Alépée N, Hoffmann S, Kern PS, Van Vliet E, Bury D, Miyazawa M, Nishida H, Cosmetics E. 2023. Applying a next generation risk assessment framework for skin sensitisation to inconsistent new approach methodology information. ALTEX. 40( 3):439–451. doi: 10.14573/altex.2211161.
- Gilmour N, Kern PS, Alépée N, Boislève F, Bury D, Clouet E, Hirota M, Hoffmann S, Kühnl J, Lalko JF, et al. 2020. Development of a next generation risk assessment framework for the evaluation of skin sensitisation of cosmetic ingredients. Regul Toxicol Pharmacol. 116:104721. doi: 10.1016/j.yrtph.2020.104721.
- Gilmour N, Kimber I, Williams J, Maxwell G. 2019. Skin sensitization: uncertainties, challenges, and opportunities for improved risk assessment. Contact Dermatitis. 80(3):195–200. doi: 10.1111/cod.13167.
- Gilmour N, Reynolds J, Przybylak K, Aleksic M, Aptula N, Baltazar MT, Cubberley R, Rajagopal R, Reynolds G, Spriggs S, et al. 2022. Next generation risk assessment for skin allergy: decision making using new approach methodologies. Regul Toxicol Pharmacol. 131:105159. doi: 10.1016/j.yrtph.2022.105159.
- Ginhoux F, Collin MP, Bogunovic M, Abel M, Leboeuf M, Helft J, Ochando J, Kissenpfennig A, Malissen B, Grisotto M, et al. 2007. Blood-derived dermal langerin + dendritic cells survey the skin in the steady state. J Exp Med. 204(13):3133–3146. doi: 10.1084/jem.20071733.
- Girolomoni G, Cruz PD, Jr., Bergstresser PR. 1990. Internalization and acidification of surface HLA-DR molecules by epidermal Langerhans cells: a paradigm for antigen processing. J Invest Dermatol. 94(6):753–760. doi: 10.1111/1523-1747.ep12874611.
- Gradin R, Forreryd A, Mattson U, Jerre A, Johansson H. 2021. Quantitative assessment of sensitizing potency using a dose–response adaptation of GARDskin. Sci Rep. 11(1):18904. doi: 10.1038/s41598-021-98247-7.
- Griffiths CE, Nickoloff BJ. 1989. Keratinocyte intercellular adhesion molecule-1 (ICAM-1) expression precedes dermal T lymphocytic infiltration in allergic contact dermatitis (Rhus dermatitis). Am J Pathol. 135(6):1045–1053.
- Groves RW, Allen MH, Ross EL, Barker JN, MacDonald DM. 1995. Tumour necrosis factor alpha is pro-inflammatory in normal human skin and modulates cutaneous adhesion molecule expression. Br J Dermatol. 132(3):345–352. doi: 10.1111/j.1365-2133.1995.tb08666.x.
- Han B, Stevens JF, Maier CS. 2007. Design, synthesis, and application of a hydrazide-functionalized isotope-coded affinity tag for the quantification of oxylipid-protein conjugates. Anal Chem. 79(9):3342–3354. doi: 10.1021/ac062262a.
- Helou DG, Martin SF, Pallardy M, Chollet-Martin S, Kerdine-Römer S. 2019. Nrf2 involvement in chemical-induced skin innate immunity. Front Immunol. 10:1004. doi: 10.3389/fimmu.2019.01004.
- Hernandez-Jaimes OA, Cazares-Olvera DV, Line J, Moreno-Eutimio MA, Gómez-Castro CZ, Naisbitt DJ, Castrejón-Flores JL. 2022. Advances in our understanding of the interaction of drugs with t-cells: implications for the discovery of biomarkers in severe cutaneous drug reactions. Chem Res Toxicol. 35(7):1162–1183. doi: 10.1021/acs.chemrestox.1c00434.
- Hooyberghs J, Schoeters E, Lambrechts N, Nelissen I, Witters H, Schoeters G, Van Den Heuvel R. 2008. A cell-based in vitro alternative to identify skin sensitizers by gene expression. Toxicol Appl Pharmacol. 231(1):103–111. doi: 10.1016/j.taap.2008.03.014.
- Hough KP, Deshane JS. 2019. Exosomes in allergic airway diseases. Curr Allergy Asthma Rep. 19(5):26. doi: 10.1007/s11882-019-0857-3.
- Hough KP, Trevor JL, Strenkowski JG, Wang Y, Chacko BK, Tousif S, Chanda D, Steele C, Antony VB, Dokland T, et al. 2018. Exosomal transfer of mitochondria from airway myeloid-derived regulatory cells to T cells. Redox Biol. 18:54–64. doi: 10.1016/j.redox.2018.06.009.
- Hulbert AJ, Kelly MA, Abbott SK. 2014. Polyunsaturated fats, membrane lipids and animal longevity. J Comp Physiol B. 184(2):149–166. doi: 10.1007/s00360-013-0786-8.
- Jacquoilleot S, Sheffield D, Olayanju A, Sison-Young R, Kitteringham NR, Naisbitt DJ, Aleksic M. 2015. Glutathione metabolism in the HaCaT cell line as a model for the detoxification of the model sensitisers 2,4-dinitrohalobenzenes in human skin. Toxicol Lett. 237(1):11–20. doi: 10.1016/j.toxlet.2015.05.016.
- Jakob T, Udey MC. 1998. Regulation of E-cadherin-mediated adhesion in Langerhans cell-like dendritic cells by inflammatory mediators that mobilize Langerhans cells in vivo. J Immunol. 160(8):4067–4073. doi: 10.4049/jimmunol.160.8.4067.
- Jaworska J, Dancik Y, Kern P, Gerberick F, Natsch A. 2013. Bayesian integrated testing strategy to assess skin sensitization potency: from theory to practice. J Appl Toxicol. 33(11):1353–1364. doi: 10.1002/jat.2869.
- Jella KK, Nasti TH, Li Z, Malla SR, Buchwald ZS, Khan MK. 2018. Exosomes, their biogenesis and role in inter-cellular communication, tumor microenvironment and cancer immunotherapy. Vaccines (Basel). 6(4):69. doi: 10.3390/vaccines6040069.
- Ji C, Kozak KR, Marnett LJ. 2001. IkappaB kinase, a molecular target for inhibition by 4-hydroxy-2-nonenal. J Biol Chem. 276(21):18223–18228. doi: 10.1074/jbc.M101266200.
- Jiang D, Liang J, Noble PW. 2011. Hyaluronan as an immune regulator in human diseases. Physiol Rev. 91(1):221–264. doi: 10.1152/physrev.00052.2009.
- Johansson H, Albrekt AS, Borrebaeck CA, Lindstedt M. 2013. The GARD assay for assessment of chemical skin sensitizers. Toxicol In Vitro. 27(3):1163–1169. doi: 10.1016/j.tiv.2012.05.019.
- Johansson H, Gradin R, Johansson A, Adriaens E, Edwards A, Zuckerstätter V, Jerre A, Burleson F, Gehrke H, Roggen EL. 2019. Validation of the GARD™skin assay for assessment of chemical skin sensitizers: ring trial results of predictive performance and reproducibility. Toxicol Sci. 170(2):374–381. doi: 10.1093/toxsci/kfz108.
- Johansson H, Lindstedt M, Albrekt A-S, Borrebaeck CAK. 2011. A genomic biomarker signature can predict skin sensitizers using a cell-based in vitro alternative to animal tests. BMC Genomics. 12(1):399. doi: 10.1186/1471-2164-12-399.
- JRC EC. 2021. ESAC opinion on the scientific validity of the GARDskin and GARDpotency test methods. Luxembourg: Publications Office.
- Kaplan DH, Kissenpfennig A, Clausen BE. 2008. Insights into Langerhans cell function from Langerhans cell ablation models. Eur J Immunol. 38(9):2369–2376. doi: 10.1002/eji.200838397.
- Karlsson I, Samuelsson K, Ponting DJ, Törnqvist M, Ilag LL, Nilsson U. 2016. Peptide reactivity of isothiocyanates – implications for skin allergy. Sci Rep. 6(1):21203. doi: 10.1038/srep21203.
- Karlsson I, Samuelsson K, Simonsson C, Stenfeldt A-L, Nilsson U, Ilag LL, Jonsson C, Karlberg A-T. 2018. The fate of a hapten - from the skin to modification of macrophage migration inhibitory factor (MIF) in lymph nodes. Sci Rep. 8(1):2895. doi: 10.1038/s41598-018-21327-8.
- Kavasi RM, Berdiaki A, Spyridaki I, Papoutsidakis A, Corsini E, Tsatsakis A, Tzanakakis GN, Nikitovic D. 2019. Contact allergen (PPD and DNCB)-induced keratinocyte sensitization is partly mediated through a low molecular weight hyaluronan (LMWHA)/TLR4/NF-κB signaling axis. Toxicol Appl Pharmacol. 377:114632. doi: 10.1016/j.taap.2019.114632.
- Kawai Y, Takeda S, Terao J. 2007. Lipidomic analysis for lipid peroxidation-derived aldehydes using gas chromatography-mass spectrometry. Chem Res Toxicol. 20(1):99–107. doi: 10.1021/tx060199e.
- Kawakami T, Isama K, Ikarashi Y, Jinno H. 2020. Evaluation of the sensitization potential of volatile and semi-volatile organic compounds using the direct peptide reactivity assay. J Toxicol Sci. 45(11):725–735. doi: 10.2131/jts.45.725.
- Kazem S, Linssen EC, Gibbs S. 2019. Skin metabolism phase I and phase II enzymes in native and reconstructed human skin: a short review. Drug Discov Today. 24(9):1899–1910. doi: 10.1016/j.drudis.2019.06.002.
- Kim S-M, Studnitzer B, Esser-Kahn A. 2022. Heat shock protein 90’s mechanistic role in contact hypersensitivity. J Immunol. 208(12):2622–2631. doi: 10.4049/jimmunol.2101023.
- Kimber I, Basketter DA, Gerberick GF, Dearman RJ. 2002. Allergic contact dermatitis. Int Immunopharmacol. 2(2–3):201–211. doi: 10.1016/s1567-5769(01)00173-4.
- Kimber I, Basketter DA, Gerberick GF, Ryan CA, Dearman RJ. 2011. Chemical allergy: translating biology into hazard characterization. Toxicol Sci. 120(Supplement 1):S238–S268. doi: 10.1093/toxsci/kfq346.
- Kimber I, Maxwell G, Gilmour N, Dearman RJ, Friedmann PS, Martin SF. 2012. Allergic contact dermatitis: a commentary on the relationship between T lymphocytes and skin sensitising potency. Toxicology. 291(1–3):18–24. doi: 10.1016/j.tox.2011.11.007.
- Kissenpfennig A, Henri S, Dubois B, Laplace-Builhé C, Perrin P, Romani N, Tripp CH, Douillard P, Leserman L, Kaiserlian D, et al. 2005. Dynamics and function of Langerhans cells in vivo: dermal dendritic cells colonize lymph node areas distinct from slower migrating Langerhans cells. Immunity. 22(5):643–654. doi: 10.1016/j.immuni.2005.04.004.
- Klechevsky E, Morita R, Liu M, Cao Y, Coquery S, Thompson-Snipes L, Briere F, Chaussabel D, Zurawski G, Palucka AK, et al. 2008. Functional specializations of human epidermal Langerhans cells and CD14+ dermal dendritic cells. Immunity. 29(3):497–510. doi: 10.1016/j.immuni.2008.07.013.
- Kleinstreuer NC, Hoffmann S, Alépée N, Allen D, Ashikaga T, Casey W, Clouet E, Cluzel M, Desprez B, Gellatly N, et al. 2018. Non-animal methods to predict skin sensitization (II): an assessment of defined approaches (*). Crit Rev Toxicol. 48(5):359–374. doi: 10.1080/10408444.2018.1429386.
- Knox S, O’Boyle NM. 2021. Skin lipids in health and disease: a review. Chem Phys Lipids. 236:105055. doi: 10.1016/j.chemphyslip.2021.105055.
- Kotzerke K, Mempel M, Aung T, Wulf GG, Urlaub H, Wenzel D, Schön MP, Braun A. 2013. Immunostimulatory activity of murine keratinocyte-derived exosomes. Exp Dermatol. 22(10):650–655. doi: 10.1111/exd.12230.
- Krishnan S, Miller RM, Tian B, Mullins RD, Jacobson MP, Taunton J. 2014. Design of reversible, cysteine-targeted Michael acceptors guided by kinetic and computational analysis. J Am Chem Soc. 136(36):12624–12630.
- Kühn U, Brand P, Willemsen J, Jonuleit H, Enk AH, van Brandwijk-Petershans R, Saloga J, Knop J, Becker D. 1998. Induction of tyrosine phosphorylation in human MHC class II-positive antigen-presenting cells by stimulation with contact sensitizers. J Immunol (Baltimore, Md: 1950). 160(2):667–673. doi: 10.4049/jimmunol.160.2.667.
- Kwok BH, Koh B, Ndubuisi MI, Elofsson M, Crews CM. 2001. The anti-inflammatory natural product parthenolide from the medicinal herb Feverfew directly binds to and inhibits IkappaB kinase. Chem Biol. 8(8):759–766. doi: 10.1016/s1074-5521(01)00049-7.
- Lambrechts N, Vanheel H, Nelissen I, Witters H, Van Den Heuvel R, Van Tendeloo V, Schoeters G, Hooyberghs J. 2010. Assessment of chemical skin-sensitizing potency by an in vitro assay based on human dendritic cells. Toxicol Sci. 116(1):122–129. doi: 10.1093/toxsci/kfq108.
- Landsteiner K, Jacobs J. 1935. Studies on the sensitization of animals with simple chemical compounds. J Exp Med. 61(5):643–656. doi: 10.1084/jem.61.5.643.
- Lee C-U, Grossmann TN. 2012. Reversible covalent inhibition of a protein target. Angew. Chem. Int. Ed. 51(35):8699–8700.
- Lindberg T, de Ávila RI, Zeller KS, Levander F, Eriksson D, Chawade A, Lindstedt M. 2020. An integrated transcriptomic- and proteomic-based approach to evaluate the human skin sensitization potential of glyphosate and its commercial agrochemical formulations. J Proteomics. 217:103647. doi: 10.1016/j.jprot.2020.103647.
- Lindberg T, Forreryd A, Bergendorff O, Lindstedt M, Zeller KS. 2019. In vitro assessment of mechanistic events induced by structurally related chemical rubber sensitizers. Toxicol In Vitro. 60:144–153. doi: 10.1016/j.tiv.2019.05.006.
- Lu K, Hsiao Y-C, Liu C-W, Schoeny R, Gentry R, Starr TB. 2022. A review of stable isotope labeling and mass spectrometry methods to distinguish exogenous from endogenous DNA adducts and improve dose–response assessments. Chem Res Toxicol. 35(1):7–29. doi: 10.1021/acs.chemrestox.1c00212.
- Łuczaj W, Gęgotek A, Skrzydlewska E. 2017. Antioxidants and HNE in redox homeostasis. Free Radic Biol Med. 111:87–101. doi: 10.1016/j.freeradbiomed.2016.11.033.
- Luís A, Martins JD, Silva A, Ferreira I, Cruz MT, Neves BM. 2014. Oxidative stress-dependent activation of the eIF2α–ATF4 unfolded protein response branch by skin sensitizer 1-fluoro-2,4-dinitrobenzene modulates dendritic-like cell maturation and inflammatory status in a biphasic manner. Free Radic Biol Med. 77:217–229. doi: 10.1016/j.freeradbiomed.2014.09.008.
- Lyss G, Knorre A, Schmidt TJ, Pahl HL, Merfort I. 1998. The anti-inflammatory sesquiterpene lactone helenalin inhibits the transcription factor NF-kappaB by directly targeting p65. J Biol Chem. 273(50):33508–33516. doi: 10.1074/jbc.273.50.33508.
- Manevski N, Swart P, Balavenkatraman KK, Bertschi B, Camenisch G, Kretz O, Schiller H, Walles M, Ling B, Wettstein R, et al. 2015. Phase II metabolism in human skin: skin explants show full coverage for glucuronidation, sulfation, N-acetylation, catechol methylation, and glutathione conjugation. Drug Metab Dispos. 43(1):126–139. doi: 10.1124/dmd.114.060350.
- Marnett LJ, Riggins JN, West JD. 2003. Endogenous generation of reactive oxidants and electrophiles and their reactions with DNA and protein. J Clin Invest. 111(5):583–593. doi: 10.1172/JCI200318022.
- Martin SF. 2017. The role of the innate immune system in allergic contact dermatitis. Allergol Select. 1(1):39–43. doi: 10.5414/ALX01274E.
- Martin SF, Esser PR, Weber FC, Jakob T, Freudenberg MA, Schmidt M, Goebeler M. 2011. Mechanisms of chemical-induced innate immunity in allergic contact dermatitis. Allergy. 66(9):1152–1163. doi: 10.1111/j.1398-9995.2011.02652.x.
- Matos TJ, Jaleco SP, Gonçalo M, Duarte CB, Lopes MC. 2005. Release of IL-1beta via IL-1beta-converting enzyme in a skin dendritic cell line exposed to 2,4-dinitrofluorobenzene. Mediators Inflamm. 2005(3):131–138. doi: 10.1155/MI.2005.131.
- Mattii M, Ayala F, Balato N, Filotico R, Lembo S, Schiattarella M, Patruno C, Marone G, Balato A. 2013. The balance between pro- and anti-inflammatory cytokines is crucial in human allergic contact dermatitis pathogenesis: the role of IL-1 family members. Exp Dermatol. 22(12):813–819. doi: 10.1111/exd.12272.
- Mayumi N, Watanabe E, Norose Y, Watari E, Kawana S, Geijtenbeek TBH, Takahashi H. 2013. E-cadherin interactions are required for Langerhans cell differentiation. Eur J Immunol. 43(1):270–280. doi: 10.1002/eji.201242654.
- McKim JM, Jr., Keller DJ, 3rd, Gorski JR. 2012. An in vitro method for detecting chemical sensitization using human reconstructed skin models and its applicability to cosmetic, pharmaceutical, and medical device safety testing. Cutan Ocul Toxicol. 31(4):292–305. doi: 10.3109/15569527.2012.667031.
- Mee JB, Alam Y, Groves RW. 2000. Human keratinocytes constitutively produce but do not process interleukin-18. Br J Dermatol. 143(2):330–336. doi: 10.1046/j.1365-2133.2000.03759.x.
- Mehrotra P, Mishra KP, Raman G, Banerjee G. 2005. Differential regulation of free radicals (reactive oxygen and nitrogen species) by contact allergens and irritants in human keratinocyte cell line. Toxicol Mech Methods. 15(5):343–350. doi: 10.1080/15376520500191490.
- Merad M, Ginhoux F, Collin M. 2008. Origin, homeostasis and function of Langerhans cells and other langerin-expressing dendritic cells. Nat Rev Immunol. 8(12):935–947. doi: 10.1038/nri2455.
- Metz B, Kersten GF, Baart GJ, de Jong A, Meiring H, ten Hove J, van Steenbergen MJ, Hennink WE, Crommelin DJ, Jiskoot W. 2006. Identification of formaldehyde-induced modifications in proteins: reactions with insulin. Bioconjug Chem. 17(3):815–822. doi: 10.1021/bc050340f.
- Metz B, Kersten GFA, Hoogerhout P, Brugghe HF, Timmermans HAM, de Jong A, Meiring H, ten Hove J, Hennink WE, Crommelin DJA, et al. 2004. Identification of formaldehyde-induced modifications in proteins: reactions with model peptides. J Biol Chem. 279(8):6235–6243. doi: 10.1074/jbc.M310752200.
- Michiels TJM, Schöneich C, Hamzink MRJ, Meiring HD, Kersten GFA, Jiskoot W, Metz B. 2020. Novel formaldehyde-induced modifications of lysine residue pairs in peptides and proteins: identification and relevance to vaccine development. Mol Pharm. 17(11):4375–4385. doi: 10.1021/acs.molpharmaceut.0c00851.
- Migneault I, Dartiguenave C, Bertrand MJ, Waldron KC. 2004. Glutaraldehyde: behavior in aqueous solution, reaction with proteins, and application to enzyme crosslinking. Biotechniques. 37(5):790–802. doi: 10.2144/04375RV01.
- Mizuashi M, Ohtani T, Nakagawa S, Aiba S. 2005. Redox imbalance induced by contact sensitizers triggers the maturation of dendritic cells. J Invest Dermatol. 124(3):579–586. doi: 10.1111/j.0022-202X.2005.23624.x.
- Mizumoto N, Mummert ME, Shalhevet D, Takashima A. 2003. Keratinocyte ATP release assay for testing skin-irritating potentials of structurally diverse chemicals. J Invest Dermatol. 121(5):1066–1072. doi: 10.1046/j.1523-1747.2003.12558.x.
- Mol M, Regazzoni L, Altomare A, Degani G, Carini M, Vistoli G, Aldini G. 2017. Enzymatic and non-enzymatic detoxification of 4-hydroxynonenal: methodological aspects and biological consequences. Free Radic Biol Med. 111:328–344. doi: 10.1016/j.freeradbiomed.2017.01.036.
- Moll R, Divo M, Langbein L. 2008. The human keratins: biology and pathology. Histochem Cell Biol. 129(6):705–733. doi: 10.1007/s00418-008-0435-6.
- Moussallieh FM, Moss E, Elbayed K, Lereaux G, Tourneix F, Lepoittevin JP. 2020. Modifications induced by chemical skin allergens on the metabolome of reconstructed human epidermis: a pilot high-resolution magic angle spinning nuclear magnetic resonance study. Contact Dermatitis. 82(3):137–146. doi: 10.1111/cod.13415.
- Naik SM, Cannon G, Burbach GJ, Singh SR, Swerlick RA, Wilcox JN, Ansel JC, Caughman SW. 1999. Human keratinocytes constitutively express interleukin-18 and secrete biologically active interleukin-18 after treatment with pro-inflammatory mediators and dinitrochlorobenzene. J Invest Dermatol. 113(5):766–772. doi: 10.1046/j.1523-1747.1999.00750.x.
- Nakahara T, Moroi Y, Uchi H, Furue M. 2006. Differential role of MAPK signaling in human dendritic cell maturation and Th1/Th2 engagement. J Dermatol Sci. 42(1):1–11. doi: 10.1016/j.jdermsci.2005.11.004.
- Natsch A. 2023. Integrated skin sensitization assessment based on OECD methods (III): adding human data to the assessment. ALTEX. 40(4):571–583. doi: 10.14573/altex.2302081.
- Natsch A, Emter R. 2016. Nrf2 activation as a key event triggered by skin sensitisers: the development of the stable KeratinoSens reporter gene assay. Altern Lab Anim. 44(5):443–451. doi: 10.1177/026119291604400513.
- Natsch A, Emter R. 2017. Reaction chemistry to characterize the molecular initiating event in skin sensitization: a journey to be continued. Chem Res Toxicol. 30(1):315–331. doi: 10.1021/acs.chemrestox.6b00365.
- Natsch A, Emter R, Haupt T, Ellis G. 2018. Deriving a no expected sensitization induction level for fragrance ingredients without animal testing: an integrated approach applied to specific case studies. Toxicol Sci. 165(1):170–185. doi: 10.1093/toxsci/kfy135.
- Natsch A, Gerberick GF. 2022a. Integrated skin sensitization assessment based on OECD methods (I): deriving a point of departure for risk assessment. ALTEX. 39(4):636–646. doi: 10.14573/altex.2201141s4.
- Natsch A, Gerberick GF. 2022b. Integrated skin sensitization assessment based on OECD methods (II): hazard and potency by combining kinetic peptide reactivity and the “2 out of 3” defined approach. ALTEX. 39(4):647–655. doi: 10.14573/altex.2201142s4.
- Natsch A, Gfeller H. 2008. LC-MS-based characterization of the peptide reactivity of chemicals to improve the in vitro prediction of the skin sensitization potential. Toxicol Sci. 106(2):464–478. doi: 10.1093/toxsci/kfn194.
- Natsch A, Gfeller H, Rothaupt M, Ellis G. 2007. Utility and limitations of a peptide reactivity assay to predict fragrance allergens in vitro. Toxicol In Vitro. 21(7):1220–1226. doi: 10.1016/j.tiv.2007.03.016.
- Natsch A, Haupt T, Laue H. 2011. Relating skin sensitizing potency to chemical reactivity: reactive Michael acceptors inhibit NF-κB signaling and are less sensitizing than S(N)Ar- and S(N)2- reactive chemicals. Chem Res Toxicol. 24(11):2018–2027. doi: 10.1021/tx2003678.
- Natsch A, Haupt T, Wareing B, Landsiedel R, Kolle SN. 2020. Predictivity of the kinetic direct peptide reactivity assay (kDPRA) for sensitizer potency assessment and GHS subclassification. ALTEX. 37(4):652–664.
- Ndreu L, Erber LN, Törnqvist M, Tretyakova NY, Karlsson I. 2020. Characterizing adduct formation of electrophilic skin allergens with human serum albumin and hemoglobin. Chem Res Toxicol. 33(10):2623–2636. doi: 10.1021/acs.chemrestox.0c00271.
- Ndreu L, Sasse S, Karlberg AT, Karlsson I. 2022. Haptenation of macrophage migration inhibitory factor: a potential biomarker for contact hypersensitivity. Front Toxicol. 4:856614. doi: 10.3389/ftox.2022.856614.
- Negre-Salvayre A, Coatrieux C, Ingueneau C, Salvayre R. 2008. Advanced lipid peroxidation end products in oxidative damage to proteins. Potential role in diseases and therapeutic prospects for the inhibitors. Br J Pharmacol. 153(1):6–20. doi: 10.1038/sj.bjp.0707395.
- Nikitovic D, Berdiaki A, Galbiati V, Kavasi RM, Papale A, Tsatsakis A, Tzanakakis GN, Corsini E. 2015. Hyaluronan regulates chemical allergen-induced IL-18 production in human keratinocytes. Toxicol Lett. 232(1):89–97. doi: 10.1016/j.toxlet.2014.09.026.
- Niklasson IB, Ponting DJ, Luthman K, Karlberg AT. 2014. Bioactivation of cinnamic alcohol forms several strong skin sensitizers. Chem Res Toxicol. 27(4):568–575. doi: 10.1021/tx400428f.
- Nordberg J, Arnér ESJ. 2001. Reactive oxygen species, antioxidants, and the mammalian thioredoxin system. Free Radical Biol Med. 31(11):1287–1312. doi: 10.1016/S0891-5849(01)00724-9.
- Nukada Y, Miyazawa M, Kosaka N, Ito Y, Sakaguchi H, Nishiyama N. 2008. Production of IL-8 in THP-1 cells following contact allergen stimulation via mitogen-activated protein kinase activation or tumor necrosis factor-alpha production. J Toxicol Sci. 33(2):175–185. doi: 10.2131/jts.33.175.
- OECD. 2010a. Test No. 429: skin sensitisation: Local lymph node assay, OECD guidelines for the testing of chemicals, Section 4. Paris: OECD Publishing. doi: 10.1787/9789264071100-en.
- OECD. 2010b. Test No. 442A: skin sensitization: Local lymph node assay: DA, OECD guidelines for the testing of chemicals, Section 4. Paris: OECD Publishing. doi: 10.1787/9789264090972-en.
- OECD. 2014. The adverse outcome pathway for skin sensitisation initiated by covalent binding to proteins. OECD series on testing and assessment, No. 168. Paris: OECD Publishing. doi: 10.1787/9789264221444-en.
- OECD. 2018. Test No. 442B: skin sensitization: Local lymph node assay: BrdU-ELISA or –FCM, OECD guidelines for the testing of chemicals, Section 4. Paris: OECD Publishing. doi: 10.1787/9789264090996-en.
- OECD. 2022a. Test No. 442D: in vitro skin sensitisation: ARE-Nrf2 Luciferase test method, OECD Guidelines for the Testing of Chemicals, Section 4. Paris: OECD Publishing. doi: 10.1787/9789264229822-en.
- OECD. 2022b. Test No. 406: skin sensitisation, OECD guidelines for the testing of chemicals, Section 4. Paris: OECD Publishing. doi: 10.1787/9789264070660-en.
- OECD. 2022c. Report on considerations from case studies on integrated approaches for testing and assessment (IATA). OECD Series on Testing and Assessment No 369. Paris: OECD Publishing.
- OECD. 2023a. Guideline No. 497: defined approaches on skin sensitisation, OECD guidelines for the testing of chemicals, Section 4. Paris: OECD Publishing. doi: 10.1787/b92879a4-en.
- OECD. 2023b. Test No. 442C: in chemico skin sensitisation: assays addressing the adverse outcome pathway key event on covalent binding to proteins, OECD guidelines for the testing of chemicals, Section 4. Paris: OECD Publishing. doi: 10.1787/9789264229709-en.
- OECD. 2023c. Test No. 442E: in vitro skin sensitisation: in vitro skin sensitisation assays addressing the key event on activation of dendritic cells on the adverse outcome pathway for skin sensitisation, OECD guidelines for the testing of chemicals, Section 4. Paris: OECD Publishing, Paris. doi: 10.1787/9789264264359-en
- Oesch F, Fabian E, Guth K, Landsiedel R. 2014. Xenobiotic-metabolizing enzymes in the skin of rat, mouse, pig, guinea pig, man, and in human skin models. Arch Toxicol. 88(12):2135–2190. doi: 10.1007/s00204-014-1382-8.
- Pamplona R. 2011. Advanced lipoxidation end-products. Chem Biol Interact. 192(1–2):14–20. doi: 10.1016/j.cbi.2011.01.007.
- Papac-Milicevic N, Busch CJL, Binder CJ. 2016. Chapter one - Malondialdehyde epitopes as targets of immunity and the implications for atherosclerosis. In: Alt FW, editor. Advances in immunology. Cambridge (MA): Academic Press; p. 1–59.
- Parkinson E, Aleksic M, Arthur R, Regufe Da Mota S, Cubberley R, Skipp PJ. 2020a. Proteomic analysis of haptenation by skin sensitisers: diphencyprone and ethyl acrylate. Toxicol In Vitro. 62:104697. doi: 10.1016/j.tiv.2019.104697.
- Parkinson E, Aleksic M, Cubberley R, Kaur-Atwal G, Vissers JPC, Skipp P. 2018. Determination of protein haptenation by chemical sensitisers within the complexity of the human skin proteome. Toxicol Sci. 162(2):429–438. doi: 10.1093/toxsci/kfx265.
- Parkinson E, Aleksic M, Kukic P, Bailey A, Cubberley R, Skipp P. 2020b. Proteomic analysis of the cellular response to a potent sensitiser unveils the dynamics of haptenation in living cells. Toxicology. 445:152603. doi: 10.1016/j.tox.2020.152603.
- Parkinson E, Boyd P, Aleksic M, Cubberley R, O’Connor D, Skipp P. 2014. Stable isotope labeling method for the investigation of protein haptenation by electrophilic skin sensitizers. Toxicol Sci. 142(1):239–249. doi: 10.1093/toxsci/kfu168.
- Patlewicz G, Casati S, Basketter DA, Asturiol D, Roberts DW, Lepoittevin J-P, Worth AP, Aschberger K. 2016. Can currently available non-animal methods detect pre and pro-haptens relevant for skin sensitization? Regul Toxicol Pharmacol. 82:147–155. doi: 10.1016/j.yrtph.2016.08.007.
- Patlewicz GY, Wright ZM, Basketter DA, Pease CK, Lepoittevin J-P, Arnau EG. 2002. Structure-activity relationships for selected fragrance allergens. Contact Dermatitis. 47(4):219–226. doi: 10.1034/j.1600-0536.2002.470406.x.
- Piroird C, Ovigne JM, Rousset F, Martinozzi-Teissier S, Gomes C, Cotovio J, Alépée N. 2015. The myeloid U937 skin sensitization test (U-SENS) addresses the activation of dendritic cell event in the adverse outcome pathway for skin sensitization. Toxicol In Vitro. 29(5):901–916. doi: 10.1016/j.tiv.2015.03.009.
- Pizzorno J. 2014. Glutathione! Integr Med (Encinitas). 13(1):8–12.
- Ponec M, Weerheim A, Kempenaar J, Mommaas AM, Nugteren DH. 1988. Lipid composition of cultured human keratinocytes in relation to their differentiation. J Lipid Res. 29(7):949–961. doi: 10.1016/S0022-2275(20)38476-5.
- Rambukkana A, Pistoor FH, Bos JD, Kapsenberg ML, Das PK. 1996. Effects of contact allergens on human Langerhans cells in skin organ culture: migration, modulation of cell surface molecules, and early expression of interleukin-1 beta protein. Lab Invest. 74(2):422–436.
- Ramirez T, Mehling A, Kolle SN, Wruck CJ, Teubner W, Eltze T, Aumann A, Urbisch D, van Ravenzwaay B, Landsiedel R. 2014. LuSens: a keratinocyte based ARE reporter gene assay for use in integrated testing strategies for skin sensitization hazard identification. Toxicol In Vitro. 28(8):1482–1497. doi: 10.1016/j.tiv.2014.08.002.
- Read A, Schröder M. 2021. The unfolded protein response: an overview. Biology (Basel). 10(5):384. doi: 10.3390/biology10050384.
- Reynolds G, Reynolds J, Gilmour N, Cubberley R, Spriggs S, Aptula A, Przybylak K, Windebank S, Maxwell G, Baltazar MT. 2021. A hypothetical skin sensitisation next generation risk assessment for coumarin in cosmetic products. Regul Toxicol Pharmacol. 127:105075. doi: 10.1016/j.yrtph.2021.105075.
- Reynolds J, Gilmour N, Baltazar MT, Reynolds G, Windebank S, Maxwell G. 2022. Decision making in next generation risk assessment for skin allergy: using historical clinical experience to benchmark risk. Regul Toxicol Pharmacol. 134:105219. doi: 10.1016/j.yrtph.2022.105219.
- Reynolds J, Gilmour N, Reynolds G, Reinkeb EN, Strickland J, Germolec D, Allen DG, Maxwell G, Kleinstreuer NC. 2024. Deriving a point of departure for Quantitative Risk Assessment for Skin Sensitisation: defined approach application to isothiazolinones. Manuscript in preparation.
- Reynolds J, MacKay C, Gilmour N, Miguel-Vilumbrales D, Maxwell G. 2019. Probabilistic prediction of human skin sensitiser potency for use in next generation risk assessment. Comput Toxicol. 9:36–49. doi: 10.1016/j.comtox.2018.10.004.
- Roberts DW, Aptula AO, Patlewicz G. 2007. Electrophilic chemistry related to skin sensitization. Reaction mechanistic applicability domain classification for a published data set of 106 chemicals tested in the mouse local lymph node assay. Chem Res Toxicol. 20(1):44–60. doi: 10.1021/tx060121y.
- Roberts DW, Natsch A. 2009. High throughput kinetic profiling approach for covalent binding to peptides: application to skin sensitization potency of Michael acceptor electrophiles. Chem Res Toxicol. 22(3):592–603. doi: 10.1021/tx800431x.
- Roberts DW, Schultz TW, Api AM. 2017. Skin sensitization QMM for HRIPT NOEL data: aldehyde Schiff-Base domain. Chem Res Toxicol. 30(6):1309–1316. doi: 10.1021/acs.chemrestox.7b00050.
- Rustom A, Saffrich R, Markovic I, Walther P, Gerdes H-H. 2004. Nanotubular highways for intercellular organelle transport. Science. 303(5660):1007–1010. doi: 10.1126/science.1093133.
- Ryan CA, Troutman JA, Kern PS, Quijano M, Dobson RLM, Jian Dai H, Burt TM, Gerberick GF. 2020. Refinement of the peroxidase peptide reactivity assay and prediction model for assessing skin sensitization potential. Toxicol Sci. 178(1):88–103. doi: 10.1093/toxsci/kfaa137.
- Saito K, Takenouchi O, Nukada Y, Miyazawa M, Sakaguchi H. 2017. An in vitro skin sensitization assay termed EpiSensA for broad sets of chemicals including lipophilic chemicals and pre/pro-haptens. Toxicol In Vitro. 40:11–25. doi: 10.1016/j.tiv.2016.12.005.
- Sánchez-Gómez FJ, Díez-Dacal B, García-Martín E, Agúndez JA, Pajares MA, Pérez-Sala D. 2016. Detoxifying enzymes at the cross-roads of inflammation, oxidative stress, and drug hypersensitivity: role of glutathione transferase P1-1 and aldose reductase. Front Pharmacol. 7:237. doi: 10.3389/fphar.2016.00237.
- Sand J, Haertel E, Biedermann T, Contassot E, Reichmann E, French LE, Werner S, Beer HD. 2018. Expression of inflammasome proteins and inflammasome activation occurs in human, but not in murine keratinocytes. Cell Death Dis. 9(2):24. doi: 10.1038/s41419-017-0009-4.
- Sanderson PN, Simpson W, Cubberley R, Aleksic M, Gutsell S, Russell PJ. 2016. Mechanistic understanding of molecular initiating events (MIEs) using NMR spectroscopy. Toxicol Res (Camb). 5(1):34–44. doi: 10.1039/c5tx00246j.
- Schaefer L. 2014. Complexity of danger: the diverse nature of damage-associated molecular patterns. J Biol Chem. 289(51):35237–35245. doi: 10.1074/jbc.R114.619304.
- Scheibner KA, Lutz MA, Boodoo S, Fenton MJ, Powell JD, Horton MR. 2006. Hyaluronan fragments act as an endogenous danger signal by engaging TLR2. J Immunol. 177(2):1272–1281. doi: 10.4049/jimmunol.177.2.1272.
- Scheinman PL, Vocanson M, Thyssen JP, Johansen JD, Nixon RL, Dear K, Botto NC, Morot J, Goldminz AM. 2021. Contact dermatitis. Nat Rev Dis Primers. 7(1):38. doi: 10.1038/s41572-021-00271-4.
- Schnurr M, Then F, Galambos P, Scholz C, Siegmund B, Endres S, Eigler A. 2000. Extracellular ATP and TNF-alpha synergize in the activation and maturation of human dendritic cells. J Immunol. 165(8):4704–4709. doi: 10.4049/jimmunol.165.8.4704.
- Schultz TW, Yarbrough JW, Johnson EL. 2005. Structure–activity relationships for reactivity of carbonyl-containing compounds with glutathione. SAR QSAR Environ Res. 16(4):313–322. doi: 10.1080/10659360500204152.
- Schürer N, Köhne A, Schliep V, Barlag K, Goerz G. 1993. Lipid composition and synthesis of HaCaT cells, an immortalized human keratinocyte line, in comparison with normal human adult keratinocytes. Exp Dermatol. 2(4):179–185. doi: 10.1111/j.1600-0625.1993.tb00030.x.
- Schwensen JF, White IR, Thyssen JP, Menné T, Johansen JD. 2015. Failures in risk assessment and risk management for cosmetic preservatives in Europe and the impact on public health. Contact Dermatitis. 73(3):133–141. doi: 10.1111/cod.12446.
- Simonsson C, Andersson SI, Stenfeldt A-L, Bergström J, Bauer B, Jonsson CA, Ericson MB, Broo KS. 2011. Caged fluorescent haptens reveal the generation of cryptic epitopes in allergic contact dermatitis. J Invest Dermatol. 131(7):1486–1493. doi: 10.1038/jid.2010.422.
- Singleton H, Popple A, Gellatly N, Maxwell G, Williams J, Friedmann PS, Kimber I, Dearman RJ. 2016. Anti-hapten antibodies in response to skin sensitization. Contact Dermatitis. 74(4):197–204. doi: 10.1111/cod.12486.
- Sousa BC, Pitt AR, Spickett CM. 2017. Chemistry and analysis of HNE and other prominent carbonyl-containing lipid oxidation compounds. Free Radic Biol Med. 111:294–308. doi: 10.1016/j.freeradbiomed.2017.02.003.
- Spickett CM, Wiswedel I, Siems W, Zarkovic K, Zarkovic N. 2010. Advances in methods for the determination of biologically relevant lipid peroxidation products. Free Radic Res. 44(10):1172–1202. doi: 10.3109/10715762.2010.498476.
- Spinu N, Cronin MTD, Enoch SJ, Madden JC, Worth AP. 2020. Quantitative adverse outcome pathway (qAOP) models for toxicity prediction. Arch Toxicol. 94(5):1497–1510. doi: 10.1007/s00204-020-02774-7.
- Spriggs S, Cubberley R, Loadman P, Sheffield D, Wierzbicki A. 2018. A study of inter-individual variability in the Phase II metabolism of xenobiotics in human skin. Toxicol Lett. 292:63–72. doi: 10.1016/j.toxlet.2018.04.011.
- Spriggs S, Sheffield D, Olayanju A, Kitteringham NR, Naisbitt DJ, Aleksic M. 2016. Effect of repeated daily dosing with 2,4-dinitrochlorobenzene on glutathione biosynthesis and Nrf2 activation in reconstructed human epidermis. Toxicol Sci. 154(1):5–15. doi: 10.1093/toxsci/kfw140.
- Su Q, Igyártó BZ. 2019. Keratinocytes share gene expression fingerprint with epidermal Langerhans cells via mRNA transfer. J Invest Dermatol. 139(11):2313–2323.e8. doi: 10.1016/j.jid.2019.05.006.
- Sullivan KM, Enoch SJ, Ezendam J, Sewald K, Roggen EL, Cochrane S. 2017. An adverse outcome pathway for sensitization of the respiratory tract by low-molecular-weight chemicals: building evidence to support the utility of in vitro and in silico methods in a regulatory context. Appl In Vitro Toxicol. 3(3):213–226. doi: 10.1089/aivt.2017.0010.
- Takahashi T, Kimura Y, Saito R, Nakajima Y, Ohmiya Y, Yamasaki K, Aiba S. 2011. An in vitro test to screen skin sensitizers using a stable THP-1-derived IL-8 reporter cell line, THP-G8. Toxicol Sci. 124(2):359–369. doi: 10.1093/toxsci/kfr237.
- Termeer C, Benedix F, Sleeman J, Fieber C, Voith U, Ahrens T, Miyake K, Freudenberg M, Galanos C, Simon JC. 2002. Oligosaccharides of hyaluronan activate dendritic cells via toll-like receptor 4. J Exp Med. 195(1):99–111. doi: 10.1084/jem.20001858.
- Trompezinski S, Migdal C, Tailhardat M, Le Varlet B, Courtellemont P, Haftek M, Serres M. 2008. Characterization of early events involved in human dendritic cell maturation induced by sensitizers: cross talk between MAPK signalling pathways. Toxicol Appl Pharmacol. 230(3):397–406. doi: 10.1016/j.taap.2008.03.012.
- Troutman JA, Foertsch LM, Kern PS, Dai HJ, Quijano M, Dobson RLM, Lalko JF, Lepoittevin JP, Gerberick F. 2011. The incorporation of lysine into the peroxidase peptide reactivity assay for skin sensitization assessments. Toxicol Sci. 122(2):422–436. doi: 10.1093/toxsci/kfr101.
- Tsujita-Inoue K, Atobe T, Hirota M, Ashikaga T, Kouzuki H. 2015. In silico risk assessment for skin sensitization using artificial neural network analysis. J Toxicol Sci. 40(2):193–209. doi: 10.2131/jts.40.193.
- UN. 2021. Globally harmonised system of classification and labelling of chemicals (GHS). New York, Geneva: United Nations.
- Urbisch D, Becker M, Honarvar N, Kolle SN, Mehling A, Teubner W, Wareing B, Landsiedel R. 2016. Assessment of pre- and pro-haptens using non-animal test methods for skin sensitization. Chem Res Toxicol. 29(5):901–913. doi: 10.1021/acs.chemrestox.6b00055.
- Uter W, Werfel T, Lepoittevin J-P, White IR. 2020. Contact allergy—emerging allergens and public health impact. Int J Environ Res Public Health. 17(7):2404. doi: 10.3390/ijerph17072404.
- Vallion R, Kerdine-Römer S. 2022. Regulation of the immune response to contact sensitizers by Nrf2. Contact Dermatitis. 87(1):13–19. doi: 10.1111/cod.14073.
- van der Veen JW, Hodemaekers H, Reus AA, Maas WJM, van Loveren H, Ezendam J. 2015. Human relevance of an in vitro gene signature in HaCaT for skin sensitization. Toxicol In Vitro. 29(1):81–84. doi: 10.1016/j.tiv.2014.08.010.
- van der Veen JW, Paskel RF, Smits NA, Hodemaekers H, van Loveren H, Ezendam J. 2016. The involvement of the toll-like receptor signaling and Nrf2-Keap1 pathways in the in vitro regulation of IL-8 and HMOX1 for skin sensitization. J Immunotoxicol. 13(1):1–6. doi: 10.3109/1547691X.2014.975897.
- van Vliet E, Kühnl J, Goebel C, Martinozzi-Teissier S, Alépée N, Ashikaga T, Blömeke B, Del Bufalo A, Cluzel M, Corsini E, et al. 2018. State-of-the-art and new options to assess T cell activation by skin sensitizers: cosmetics Europe workshop. ALTEX. 35(2):179–192. doi: 10.14573/altex.1709011.
- Wareing B, Urbisch D, Kolle SN, Honarvar N, Sauer UG, Mehling A, Landsiedel R. 2017. Prediction of skin sensitization potency sub-categories using peptide reactivity data. Toxicol In Vitro. 45(Pt 1):134–145. doi: 10.1016/j.tiv.2017.08.015.
- Watanabe H, Gaide O, Pétrilli V, Martinon F, Contassot E, Roques S, Kummer JA, Tschopp J, French LE. 2007. Activation of the IL-1beta-processing inflammasome is involved in contact hypersensitivity. J Invest Dermatol. 127(8):1956–1963. doi: 10.1038/sj.jid.5700819.
- Yamamoto Y, Tahara H, Usami R, Kasahara T, Jimbo Y, Hioki T, Fujita M. 2015. A novel in chemico method to detect skin sensitizers in highly diluted reaction conditions. J Appl Toxicol. 35(11):1348–1360. doi: 10.1002/jat.3139.
- Yang J, Tallman KA, Porter NA, Liebler DC. 2015. Quantitative chemoproteomics for site-specific analysis of protein alkylation by 4-hydroxy-2-nonenal in cells. Anal Chem. 87(5):2535–2541. doi: 10.1021/ac504685y.
- Zeller KS, Forreryd A, Lindberg T, Gradin R, Chawade A, Lindstedt M. 2017. The GARD platform for potency assessment of skin sensitizing chemicals. ALTEX. 34(4):539–559. doi: 10.14573/altex.1701101.
- Zepter K, Häffner A, Soohoo LF, De Luca D, Tang HP, Fisher P, Chavinson J, Elmets CA. 1997. Induction of biologically active IL-1 beta-converting enzyme and mature IL-1 beta in human keratinocytes by inflammatory and immunologic stimuli. J Immunol (Baltimore, Md: 1950). 159(12):6203–6208. doi: 10.4049/jimmunol.159.12.6203.
- Zgheib E, Gao W, Limonciel A, Aladjov H, Yang H, Tebby C, Gayraud G, Jennings P, Sachana M, Beltman JB, et al. 2019. Application of three approaches for quantitative AOP development to renal toxicity. Comput Toxicol. 11:1–13. doi: 10.1016/j.comtox.2019.02.001.
- Zhang H, Forman HJ. 2017. Signaling by 4-hydroxy-2-nonenal: exposure protocols, target selectivity and degradation. Arch Biochem Biophys. 617:145–154. doi: 10.1016/j.abb.2016.11.003.
- Zhou S, Chan E, Duan W, Huang M, Chen YZ. 2005. Drug bioactivation, covalent binding to target proteins and toxicity relevance. Drug Metab Rev. 37(1):41–213. doi: 10.1081/dmr-200028812.
- Zimniak P. 2011. Relationship of electrophilic stress to aging. Free Radic Biol Med. 51(6):1087–1105. doi: 10.1016/j.freeradbiomed.2011.05.039.