Abstract
The local injection of therapeutic drugs, including cells, oncolytic viruses and nucleic acids, into different organs is an administrative route used to achieve high drug exposure at the site of action. However, after local injection, material backflow and side effect reactions can occur. Hence, this study was carried out to investigate the effect of gelatin on backflow reduction in local injection. Gelatin particles (GPs) and hydrolyzed gelatin (HG) were injected into tissue models, including versatile training tissue (VTT), versatile training tissue tumor-in type (VTT-T), and broiler chicken muscles (BCM), using needle gauges between 23 G and 33 G. The backflow material fluid was collected with filter paper, and the backflow fluid rate was determined. The backflow rate was significantly reduced with 35 μm GPs (p value < .0001) at different concentrations up to 5% and with 75 μm GPs (p value < .01) up to 2% in the tissue models. The reduction in backflow with HG of different molecular weights showed that lower-molecular-weight HG required a higher-concentration dose (5% to 30%) and that higher-molecular-weight HG required a lower-concentration dose (7% to 8%). The backflow rate was significantly reduced with the gelatin-based formulation, in regard to the injection volumes, which varied from 10 μL to 100 μL with VTT or VTT-T and from 10 μL to 200 μL with BCM. The 35 μm GPs were injectable with needles of small gauges, which included 33 G, and the 75 μm GPs and HG were injectable with 27 G needles. The backflow rate was dependent on an optimal viscosity of the gelatin solutions. An optimal concentration of GPs or HG can prevent material backflow in local injection, and further studies with active drugs are necessary to investigate the applicability in tumor and organ injections.
Introduction
In the healthcare field, there is a growing need to improve the required drug efficacy and potency in drug administration. There are several drug delivery methods, including swallowing, inhalation, absorption, and injection. Drug administration by injection, such as parenteral administration, including intratumoral injection, and intramuscular injection, might be followed by what is commonly referred to as fluid leakage, reflux, or backflow (leak-back) at the local site after needle removal from the injection site. However, the results of some studies suggested that the amount of resulting backflow is not of clinical significance, but in these studies, backflow was found to have resulted from subcutaneous insulin administration (Hanas et al., Citation2000; Birkebaek et al., Citation2008). Thus, backflow detected at the skin surface has less or no adverse effect compared to that at other injection sites, e.g., the kidney, heart and eyes, such as in cases of cell transplantation, including the transplantation of induced pluripotent stem cells or bioactive substances (e.g., antibiotics, antibodies, and anti-inflammatory drugs) for the treatment of some medical conditions (e.g., chronic kidney disease, heart failure and age-related macular degeneration), wherein the backflow of the injectate would not only result in the loss of the optimal dose of the bioactive substance but also in side effects and complications by spreading to the surrounding area of the injection site. Moreover, there has been a rapid increase in the use of injection procedures and a significant increase in intravitreal and tumor injections (Gupta et al., Citation2010; Day et al., Citation2011; Tamura et al., Citation2015; Chaturvedi et al., Citation2019). A specific risk of various treatments requiring injection, such as oncolytic virotherapy or intravitreal therapy, is material backflow, and in principle, local reactions could occur (Præstmark et al., Citation2016). In fact, a degree of backflow in all cases in an experimental trial on cell delivery to the subretinal space has been reported (Wilson et al., Citation2017). Additionally, backflow of drugs is an apprehension of a major point of contention for the use of intratumoral chemotherapy (Hohenforst-Schmidt et al., Citation2013). For instance, ethanol ablation, which consists of the direct injection of pure ethanol into tissue to induce cell death by protein denaturation and cytoplasmic dehydration, was initially used for inoperable cancer (Shiina et al., Citation1991; Ryu et al., Citation1997). Backflow during ethanol ablation for the treatment of hepatocellular carcinomas has been reported, and some side effects include vascular and bile duct injuries, coagulation necrosis around the target region of interest, incomplete tumor coverage, and perforation of the vein of Marshall with serious complications, including atrial tachyarrhythmias (fast heartbeat following cardiac surgery) (Seki et al., Citation1989; Shiina et al., Citation1991; Koda et al., Citation1992; Kamakura et al., Citation2021). Injection studies have applied a saline-based delivery vehicle; however, biomaterial-assisted deliveries have been developed as cell carriers for optimal delivery, and injectable biomaterials purposely used to reduce backflow following injections have been reported in previous studies. Ethanol purposely incorporated in ethyl cellulose to optimize ablative therapy resulted in gel formation that served to contain the mixture near the injection site in a hamster cheek pouch model (Morhard et al., Citation2017). Additionally, a self-sealing coated 30-gauge injection of hyaluronic acid showed a reduction in the backflow of the intravitreal injected drug in a New Zealand rabbit model (Eom et al., Citation2021); however, the reduction in backflow after injection using other biomaterials is poorly understood. Chitosan, alginate, hyaluronic acid, cellulose, polyethylene glycol, collagen and gelatin are ubiquitous biomaterials. However, gelatin, a denatured form of collagen, is often used for medical purposes, including regenerative therapy and drug delivery, because of its cell adhesiveness, bioabsorbability, biocompatibility, biodegradability, safety, easy clearance from the body, and low cost (Nii, Citation2021). Medical gelatin, including nonparticle gelatin and gelatin particles (especially microparticles), has been probed for both in vitro cell culture and in vivo therapeutic studies. For instance, previous studies showed an improvement in the survival rate of rat or human cardiac stacked cell sheets exposed to iPS (induced pluripotent stem cells) incorporated in gelatin microparticles (Matsuo et al., Citation2015; Li et al., Citation2018), and the injection of gelatin microparticles containing PRP (platelet-rich plasma) or basic fibroblast growth factor or both promoted capillary formation as well as microvascular networks or differentiated stem cells toward muscle lineages (Kakudo et al., Citation2017; Mitsui et al., Citation2021). Gelatin has also been demonstrated as a drug carrier in sustained-release drug delivery systems (Young et al., Citation2005; Nahar et al., Citation2008; Kimura and Tabata, Citation2010; Foox and Zilberman, Citation2015). The pharmacological effect of the drug is also improved when it is encapsulated in GPs (Shokry et al., Citation2018). Moreover, modified gelatin can be used gelatin can be used as an ideal carrier for controlled release drugs (Foox and Zilberman, Citation2015). However, there is a paucity of data on the potential of gelatin to reduce backflow in local injections, including intramuscular or intratumoral injections, of active drugs. Moreover, while optimal methods for drug delivery, such as local administration, remain to be determined, a lack of a clinical response, for example, in clinical trials of intratumoral immunotherapy, may be a reflection of delivery failure rather than drug ineffectiveness (Sheth et al., Citation2020; Muñoz et al., Citation2021). This study aimed to evaluate the effect of gelatin solution and microparticles on the backflow of fluid following local administration in tissue models.
Methods
Gelatin solution and particle preparation
The gelatin samples used were of either porcine or bovine origin. The use of biomaterials originating from cows is risky due to the prion disease bovine spongiform encephalopathy (BSE) (Will et al., Citation1996). All bovine materials used for gelatin production are not from countries at risk of BSE or specified risk materials, and a process for prion inactivation is included in the gelatin manufacturing process. Furthermore, the OIE (International Organization for Animal Health founded as OIE has approved the safety of gelatin because of the acidic and/or alkaline treatment in the manufacturing process to obtain gelatin from the skin and bone of cows (Grobben et al., Citation2004). The gelatin samples were pigskin and bovine bone gelatin produced by acid or alkaline extraction methods. Specifically, the samples were beMatrix™ gelatin series or MedGel II™ from Nitta Gelatin, Inc. (Osaka, Japan), for which the detailed characteristics were reported in previous studies (Ikada and Tabata, Citation1998; Kadji et al., Citation2022). The beMatrix™ gelatin was dissolved in PBS to prepare 1 to 40% (w/v) hydrolyzed gelatin (HG), and the pH was adjusted to 7.4 ± 0.1 with NaOH. Moreover, the beMatrix™ gelatin was thermally dehydrated and crosslinked at 150 °C for 24 hours under vacuum. The gelatin particles (GPs) were dispersed in isopropanol to a concentration of approximately 2.5 mg/mL. The particle size distribution was measured using a laser diffraction/scattering particle size analyzer (Microtrac T3200II, Microtrac Bell, Inc.), and the cumulative 50% diameter (D 50) was calculated as the average particle diameter. GPs were suspended in PBS to prepare 1% to 5% (w/v) GP solutions.
Evaluation of particle dispersion stability and viscosity
Five percent (w/v) GPs were made. Immediately after stirring at 300 rpm for 1 minute, 3 mL of the solution was placed into a plastic cell, and the absorbance (wavelength: 600 nm) immediately after the injection was measured to obtain ‘absorbance 1’. ‘Absorbance 2’ was obtained by measuring the absorbance (wavelength: 600 nm) after standing for 1 minute immediately after the above measurement. The retention rate (%) was obtained with the formula 100× (absorbance 2)/(absorbance 1). The viscosity of the solution was measured with an MCR 302 rheometer manufactured by Anton Paar, Japan (cone plate R25, 1°, shear rate 200 s − 1) at 25 °C. After confirming that the value was stable, the value one minute after the start of the measurement was adopted.
Injection experiments
A 1 mL syringe and 23 G (inner diameter: 350 μm), 25 G (inner diameter: 250 μm), 27 G (inner diameter: 220 μm) needles manufactured by Terumo Corporation, a 30 G (inner diameter: 200 μm) needle manufactured by Nipro Corporation, or a 33 G (inner diameter: 160 μm) needle manufactured by Nippon Genetics Co., Ltd., were used to inject between 10 μL and 100 μL/200 μL (triplicate) solutions composed of either PBS solution, GPs, and HG into tissues incubated at 37 °C, which included excised broiler chicken muscle (BCMs, 200 μL) aged between 50–55 days and obtained from a local supermarket. Chicken muscle is used to practice the hands-on method of intramuscular injection on various livestock (Walker and Thelen, Citation2016). Other tissue models were versatile training tissue (VTT, 100 μL) and VTT tumor-in type (VTT-T, 100 μL), which are simulated organs for medical training whose touch, strength and elasticity are similar to those of human tissue and were manufactured by KOTOBUKI, Medical, Inc., Japan. Then, the injection needle was pulled out, and the puncture site was immediately covered with filter paper (Hanas et al., Citation2000; Juul et al., Citation2012; Præstmark et al., Citation2016) cut into 1 cm squares to absorb the backflow liquid within 10 seconds to measure the backflow liquid weight. The backflow rate (%) was calculated based on 100 mg of injected solution ().
Figure 1. A–B. Injection procedure with VTT-T mock tissue (A) and BCM tissue (B) and dye liquid (PBS colored with bromophenol blue). The backflow was collected with filter paper immediately after needle removal. 1–0 Overall view of the BCM model.1–1 Injection in the BCM model.1–2 Backflow following needle removal in the BCM model.1–3 collection of backflow with filter paper in the BCM model. 1B. 1–0 Overall view of the VTT and VTT-T models. 1–1 Injection in the VTT and VTT-T models. 1-2 Backflow following needle removal in the VTT and VTT-T models. B1-3 Collection of backflow with filter paper in the VTT and VTT-T models.
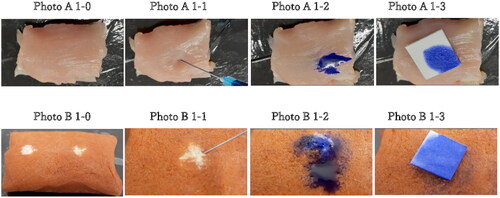
Statistical analysis
The graphical representations and statistical analysis were performed using Prism software 8.0 (GraphPad, San Diego, CA, USA). All data are presented as the mean values with standard deviations. Unpaired t tests with Welch’s correction were used for comparisons between groups, and if they passed the normality test, data were subjected to one-way ANOVA with Dunnett’s multiple comparison test to compare differences in means among more than two groups. A significance level of 5% was used throughout the study.
Results
The relevant characteristics of the gelatin particles (GPs) and hydrolyzed gelatin (HG) were determined and are summarized in . The injection of GP35 and GP75 microparticles either in the VTT, or VTT-T or BCM tissue models resulted in a significant reduction in backflow in a concentration-dependent manner and exhibited 5% for the lowest backflow with 35 μm GP (p value < .0001) or 75 µm GP (p value < .01) in each tissue model (). Additionally, the injection with 1% or 2% of 75 μm GP microparticles resulted in significant backflow reduction in the VTT model (p value < .01), VTT-T model (p value < .01), or BCM (p value < .001) (). The injection of HG solution at dose-dependent concentrations indicated that there was an optimal concentration of 20–30% for a significant backflow reduction in the VTT (p value < .01) and 30% in the VTT-T (p value < .05) and 20–30% in the BCM (p value < .001) tissue models (). We also performed studies to determine the relationship between molecular weight and backflow. The lower-molecular-weight HG appeared to require a higher concentration for optimal backflow reduction compared to the higher-molecular-weight HG, and optimal concentrations of 30%, 20%, and 8% were found for the lower-, medium- and higher-molecular-weight HG, respectively (). To evaluate the effect of needle size on backflow, the injection of gelatin microparticles or HG at various concentrations was carried out with various needle gauges. The injection of GP35 microparticles significantly reduced the backflow volume with all needle gauges, including the 25 G (VTT, p value < .0001; VTT-T, p value < .0001; BCM, p value < …), 30 G (VTT, p value < .0001; VTT-T, p value < .0001; BCM, p value < …), and 33 G (VTT, p value < .05; VTT-T, p value < .01; BCM, p value < …) (). The GP75 microparticles and HG could not pass through smaller needle sizes, including the 30 G and 33 G needles; therefore, larger needle sizes were used for the evaluation of backflow reduction in BCM, which also resulted in significant backflow reduction with the 23 G (GP75, p value < .05; HG, P value < .01), 25 G (GP75, p value < .01; HG, p value < .001) and 27 G (GP75, p value < .05; HG, p value < .0001) needles (). The effect of injection volume on backflow occurrence was also evaluated in all three tissue models. The results showed negligible backflow with low injection volumes, including 10 μL and 20 μL, regardless of the tissue model. However, a significant reduction was found for the injection volume effect in VTT (50 μL, p value < .01; 100 μL, p value < .001), VTT-T (50 μL, p value < .0001; 100 μL, (GP) p value < .05; 100 μL, (HG) P value not statistically significant) and BCM (50 μL, p value < .001; 100 μL, p value < .001; 200 μL, p value < .001) (. An analysis of a series of HG solutions (HG 1, HG 2, and HG 3) with different molecular weights and different viscosities with respect to the backflow volume showed an optimal viscosity for backflow reduction with lower molecular weights ().
Figure 2. A–B. Effect of GP35 (A) and GP75 (B) microparticle concentration dependence on backflow reduction in the VTT model, VTT-T model and BCM. **: p value ≤ 0.01, ***: P value ≤ .001, ****: p value ≤ .0001. The experiment was performed with 27 G needles.
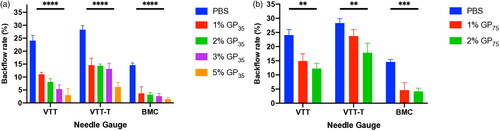
Figure 3. Effect of HG on backflow reduction in the VTT model, VTT-T and BCM model. *: p value ≤ .05. **: p value ≤ .01, ***: p value ≤ .001. The experiment was performed with 27 G needles.
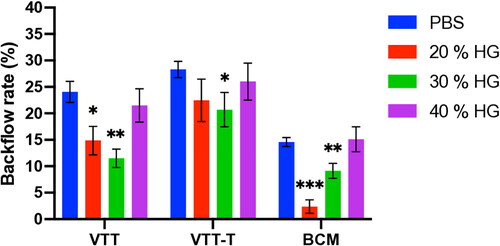
Figure 4. A–C. Effect of HG low (a), medium (B), and high (C) molecular weight on backflow reduction in the BCM model. **: p value ≤ .01, ***: p value ≤ .001. The experiment was performed with 27 G needles.
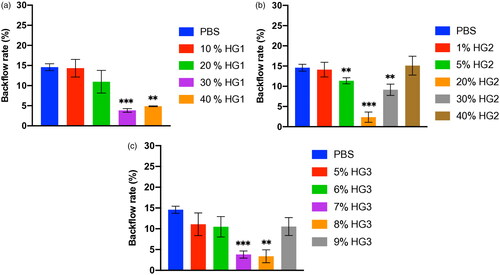
Figure 5. A–B. Effect of the needle gauge on backflow reduction in GP35 concentration-dependent injection in VTT (a), VTT-T (B) tissues. *: p value ≤ .05, **: p value ≤ .01, ****: p value ≤ .0001. C. Effect of the needle gauge on backflow reduction in GP75 and HG in BCM tissue. **: p value ≤ .01, ***: p value ≤ .001. The experiment was performed with 27 G needles.
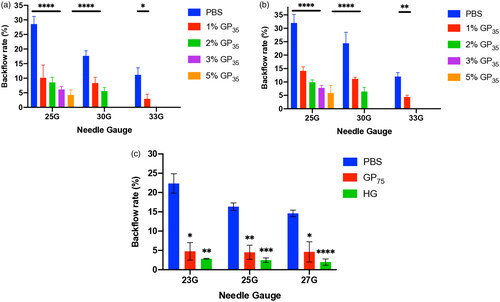
Figure 6. A-C. Effect of the injection volume on backflow reduction in GP35 and HG injection in VTT (a), VTT-T (B) and BCM (C) tissues. *: p value ≤ .05, **: p value ≤ .01, ***: p value ≤ .001, ****: P value ≤ .0001.
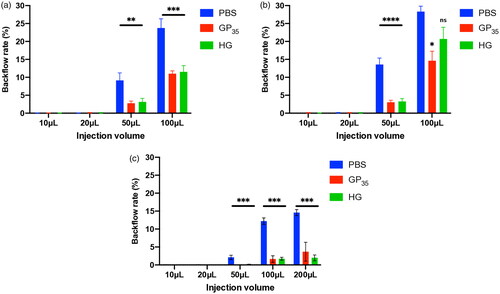
Figure 7. Relationship between gelatin viscosity and backflow reduction. Gelatin solutions HG1, HG2, and HG3 of different molecular weights were injected 3 mm deep into the BCM tissue with 27 G needles.
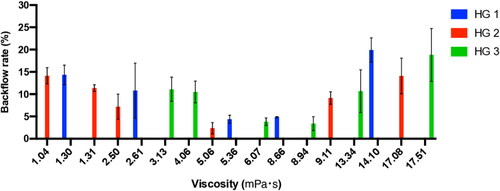
Table 1. Sample characteristics.
Discussion
This study was carried out to evaluate the effect of gelatin on backflow reduction following injection in organ models. Using different tissue models, the results demonstrated that gelatin molecules, including gelatin particles (GPs) and hydrolyzed gelatin (HG), exhibit the potential to reduce injection backflow. Gelatin is used for various applications in the medical field. For instance, there are risks of bone cement leakage in vertebroplasty (Cotten et al., Citation1996; Schmidt et al., Citation2005; Liu et al., Citation2013; Premat et al., Citation2017; Li et al., Citation2020), and gelatin is used to prevent this leakage (Xu et al., Citation2020). Some reports showed that a mixture of gelatin microparticles with other therapeutic agents demonstrated a cement leakage reduction in vertebral augmentation (Bhatia et al., Citation2006; Meng et al., Citation2013). In other studies, an intratumoral injection of a rapid drug-releasing type of gelatin in an animal model demonstrated a long-term retention of high drug concentrations with a reduction in backflow, which was not a direct result of gelatin action but rather a result of the dense tumor extracellular matrix (ECM) and angiogenic blood vessels (Park et al., Citation2022). In this study, the reduction in injection backflow was influenced by some factors, including the GP and HG concentrations and needle size. The lower-molecular-weight gelatin appeared to require a higher optimal concentration for a significant backflow reduction rate compared to the higher-molecular-weight gelatin, implying that a faster blockage of the hole occurred at the injection site. The mechanism of action remains to be elucidated. Moreover, a likely explanation may be related to the gelatin gel phase and/or viscosity. Unexpectedly, an analysis of the effect of viscosity on the backflow reduction with respect to the dose-dependent concentration showed that low viscosity was an optimal factor for backflow reduction. Additionally, the gelatin most likely remained at the administration site compared to the liquid solvent, increasing the viscosity and blocking the hole at the injection site. A possible mechanism that explains the reduction in backflow with gelatin is likely the increased viscosity of the injectate with gelatin and/or gelatin-based gel formation after exposure to the aqueous tissue environment, as suggested in previous studies (Morhard et al., Citation2017; Citation2020). We also speculated that high-viscosity gelatin is difficult to penetrate due to back-pressure to the injection force in the injection area, causing backflow from the injection site (Allmendinger et al., Citation2015). We also found that the GPs and HG significantly prevented backflow regardless of the needle size, showing a potential relevance of these molecules in preventing the loss of the active drugs through backflow following local injection. However, the injectability of HG was limited with smaller needle sizes, including 30 G and 33 G, and this limitation was caused by needle clogging and was most likely a result of the GP size and the high concentration of GPs in the case of GP75 or gelatin viscosity in the case of HG, hence indicating that these factors must be considered for the local injection of formulations containing GPs or HG with smaller needle sizes. Moreover, small needle sizes, such as 26 G or 30 G, are most often used to prevent backflow from the injection site, and our results showed reduced backflow with both the control PBS solution and the gelatin microparticles with the smallest 33 G needle used. However, the difference between them appeared significant, with GPs demonstrating the highest backflow reduction rate. To minimize backflow, a slow insertion rate is preferred (Casanova et al., Citation2014;) to lessen tissue dimpling as a result of maximizing the compressive stress between the tissue and the needle interface. Hence, an insertion rate of approximately 1 mm/s was observed during the experiments. Other factors that affect backflow are the injection volume, increased interstitial pressure and tumor size (Heise et al., Citation2014; Præstmark et al., Citation2016; Marabelle et al., Citation2018). In this study, either an injection volume of 200 μL in VTT and VTT-T or 300 μL in BCM resulted in leakage at different sites; therefore, the maximum injection volumes of 100 μL and 200 μL were adopted for VTT, VTT-T and BCM organs, respectively. The injection volume is an important factor in backflow occurrence (Heise et al., Citation2014; Præstmark et al., Citation2016). Therefore, the effect on injection volume was evaluated, and the backflow volume was positively related to the injection volumes, which was corroborated in previous studies (Heise et al., Citation2014; Mathaes et al., Citation2016; Præstmark et al., Citation2016). The backflow rates of GP and HG were reduced compared to the control for injection volumes larger than 20 μL in any of the tissue models. An increase within the tissue model of pressure by larger material deposition and the volume of the model tissue limited the evaluation of injections with larger volumes. Although this study involved a proof-of-concept evaluation of backflow after the injection of GPs and HGs with a large range of needle sizes, including those as small as 33 G, and demonstrated the plausibility of reducing injectate backflow, it would also be necessary to investigate the relevance of these findings in backflow reduction in CED (convection-enhanced delivery) for brain tumor injection therapy, where backflow remains a challenge (Casanova et al., Citation2014). Nevertheless, this study is not without limitations, thus necessitating further investigation. First, the tissues used as models to mimic intramuscular and intratumoral injections are primarily used for surgical simulation and training, namely, VTT, which is reported to provide feeling and flexibility very similar to those of real tissue; however, the VTT and VTT-T models are plant-based synthetic tissues and therefore are different in terms of composition, as well as tissue density and internal pressure, compared to animal tissue. Additionally, the results obtained from using the BCM to mimic intramuscular injection to evaluate backflow prevention using different needle sizes may differ if evaluated with another muscular organ model similar to a human muscular organ model, such as a mouse muscular organ model. However, other technical factors, including the needle insertion angle and wait time before needle removal, which are reported to influence backflow, have been observed (; S.A.B.f.t.T.I.T. Workshop, Citation2010; Præstmark et al., Citation2016). Therefore, it will be of interest and necessary to explore the ability of GPs and HGs to prevent the backflow of active drugs applied with local injection routes, including intramuscular and intratumoral injection, in preclinical studies. Notably, the gelatin products used in this study are of pharmaceutical grade, and their use in the clinical study phase is also acceptable.
Conclusion
In summary, this study showed that GPs and HG can reduce the backflow of drugs by inducing the timely closure of the hole at the injection site following local injection, and an optimal gelatin viscosity plays a key role in backflow reduction. Our findings imply that GPs or HGs can prevent the backflow of drugs through the needle passage site, and further investigation regarding applicability for backflow reduction in tumor and organ injections would be relevant.
Authors’ contributions
Conceptualization, YH, KK, and FMNK; Methodology, KK, FMNK, YM and YH; Investigation, KK and FMNK; Writing-original draft, FMNK; Review & editing, FMNK and YH; Project administration, YH and YM. All authors have read and agreed to the published version of the manuscript.
Institutional review board statement
Not applicable
Informed consent statement
Not applicable
Acknowledgments
We are grateful to both the members of the research team in the biomedical department for their technical support and Kazuya Hayashi in the R&D center for his comments on the study development at Nitta Gelatin, Inc., in Yao City, Osaka.
Disclosure statement
The authors are employees of Nitta Gelatin Inc.
Data availability statement
The data presented in this study are available upon request to the corresponding authors.
Additional information
Funding
References
- Allmendinger A, Mueller R, Schwarb E, et al. (2015). Measuring tissue back-pressure–in vivo injection forces during subcutaneous injection. Pharm Res 32:1–9. doi: 10.1007/s11095-014-1611-0.
- Bhatia C, Barzilay Y, Krishna M, et al. (2006). Cement leakage in percutaneous vertebroplasty: effect of preinjection gelfoam embolization. Spine 31:915–9. doi: 10.1097/01.brs.0000209307.03930.38.
- Birkebaek NH, Solvig J, Hansen B, et al. (2008). A 4-mm needle reduces the risk of intramuscular injections without increasing backflow to skin surface in lean diabetic children and adults. Diabetes Care 31:e65–e65. doi: 10.2337/dc08-0977.
- Casanova F, Carney PR, Sarntinoranont M. (2014). Effect of needle insertion speed on tissue injury, stress, and backflow distribution for convection-enhanced delivery in the rat brain. PLOS One 9:e94919. doi: 10.1371/journal.pone.0094919.
- Casanova F, Carney PR, Sarntinoranont M. (2014). In vivo evaluation of needle force and friction stress during insertion at varying insertion speed into the brain. J Neurosci Methods 237:79–89. doi: 10.1016/j.jneumeth.2014.08.012.
- Chaturvedi R, Wannamaker KW, Riviere PJ, et al. (2019). Real-world trends in intravitreal injection Practices among American Retina Specialists. Ophthalmol Retina 3:656–62. doi: 10.1016/j.oret.2019.03.023.
- Cotten A, Deramond H, Cortet B, et al. (1996). Preoperative percutaneous injection of methyl methacrylate and N-butyl cyanoacrylate in vertebral hemangiomas. AJNR Am J Neuroradiol 17:137–42.
- Day S, Acquah K, Lee PP, et al. (2011). Medicare costs for neovascular age-related macular degeneration, 1994-2007. Am J Ophthalmol 152:1014–20. doi: 10.1016/j.ajo.2011.05.008.
- Eom Y, Kim S, Huh J, et al. (2021). Self-sealing hyaluronic acid-coated 30-gauge intravitreal injection needles for preventing vitreous and drug reflux through needle passage. Sci Rep 11:16996. doi: 10.1038/s41598-021-96561-8.
- Foox M, Zilberman M. (2015). Drug delivery from gelatin-based systems. Expert Opin Drug Deliv 12:1547–63. doi: 10.1517/17425247.2015.1037272.
- Frid A, Hirsch L, Gaspar R, S.A.B.f.t.T.I.T. Workshop, et al. (2010). New injection recommendations for patients with diabetes. Diabetes Metab 36 Suppl 2:S3–S18. doi: 10.1016/S1262-3636(10)70002-1.
- Grobben AH, Steele PJ, Somerville RA, Taylor DM. (2004). Inactivation of the bovine-spongiform-encephalopathy (BSE) agent by the acid and alkaline processes used in the manufacture of bone gelatine. Biotechnol Appl Biochem 39:329–38. doi: 10.1042/BA20030149.
- Gupta OP, Shienbaum G, Patel AH, et al. (2010). A treat and extend regimen using ranibizumab for neovascular age-related macular degeneration clinical and economic impact. Ophthalmology 117:2134–40. doi: 10.1016/j.ophtha.2010.02.032.
- Hanas R, Lytzen L, Ludvigsson J. (2000). Thinner needles do not influence injection pain, insulin leakage or bleeding in children and adolescents with type 1 diabetes. Pediatr Diabetes 1:142–9. doi: 10.1034/j.1399-5448.2000.010305.x.
- Heise T, Nosek L, Dellweg S, et al. (2014). Impact of injection speed and volume on perceived pain during subcutaneous injections into the abdomen and thigh: a single-centre, randomized controlled trial. Diabetes Obes Metab 16:971–6. doi: 10.1111/dom.12304.
- Hohenforst-Schmidt W, Zarogoulidis P, Darwiche K, et al. (2013). Intratumoral chemotherapy for lung cancer: re-challenge current targeted therapies. Drug Des Devel Ther 7:571–83. doi: 10.2147/DDDT.S46393.
- Ikada Y, Tabata Y. (1998). Protein release from gelatin matrices. Adv Drug Deliv Rev 31:287–301. doi: 10.1016/s0169-409x(97)00125-7.
- Juul KA, Bengtsson H, Eyving B, et al. (2012). Influence of hypodermic needle dimensions on subcutaneous injection delivery–a pig study of injection deposition evaluated by CT scanning, histology, and backflow. Skin Res Technol 18:447–55. doi: 10.1111/j.1600-0846.2011.00592.x.
- Kadji FMN, Kotani K, Tsukamoto H, et al. (2022). Stability of enveloped and nonenveloped viruses in hydrolyzed gelatin liquid formulation. Virol J 19:94. doi: 10.1186/s12985-022-01819-w.
- Kakudo N, Morimoto N, Ogawa T, et al. (2017). Angiogenic effect of platelet-rich plasma combined with gelatin hydrogel granules injected into murine subcutis. J Tissue Eng Regen Med 11:1941–8. doi: 10.1002/term.2091.
- Kamakura T, Derval N, Duchateau J, et al. (2021). Vein of Marshall ethanol infusion: feasibility, pitfalls, and complications in over 700 patients. Circ Arrhythm Electrophysiol 14:e010001.
- Kimura Y, Tabata Y. (2010). Controlled release of stromal-cell-derived factor-1 from gelatin hydrogels enhances angiogenesis. J Biomater Sci Polym Ed 21:37–51. doi: 10.1163/156856209X410193.
- Koda M, Okamoto K, Miyoshi Y, Kawasaki H. (1992). Hepatic vascular and bile duct injury after ethanol injection therapy for hepatocellular carcinoma. Gastrointest Radiol 17:167–9. doi: 10.1007/BF01888537.
- Liu XW, Jin P, Wang LJ, et al. (2013). Vertebroplasty in the treatment of symptomatic vertebral haemangiomas without neurological deficit. Eur Radiol 23:2575–81. doi: 10.1007/s00330-013-2843-9.
- Li Z, Masumoto H, Jo JI, et al. (2018). Sustained release of basic fibroblast growth factor using gelatin hydrogel improved left ventricular function through the alteration of collagen subtype in a rat chronic myocardial infarction model. Gen Thorac Cardiovasc Surg 66:641–7. doi: 10.1007/s11748-018-0969-z.
- Li Z, Yu K, Chang X, et al. (2020). Cement leakage following percutaneous kyphoplasty in a patient after a posterior lumbar fusion: a case report. BMC Surg 20:74. doi: 10.1186/s12893-020-00733-8.
- Marabelle A, Andtbacka R, Harrington K, et al. (2018). Starting the fight in the tumor: expert recommendations for the development of human intratumoral immunotherapy (HIT-IT. Ann Oncol 29:2163–74.), doi: 10.1093/annonc/mdy423.
- Mathaes R, Koulov A, Joerg S, Mahler HC. (2016). Subcutaneous injection volume of biopharmaceuticals-pushing the boundaries. J Pharm Sci 105:2255–9. doi: 10.1016/j.xphs.2016.05.029.
- Matsuo T, Masumoto H, Tajima S, et al. (2015). Efficient long-term survival of cell grafts after myocardial infarction with thick viable cardiac tissue entirely from pluripotent stem cells. Sci Rep 5:16842. doi: 10.1038/srep16842.
- Meng B, Qian M, Xia SX, et al. (2013). Biomechanical characteristics of cement/gelatin mixture for prevention of cement leakage in vertebral augmentation. Eur Spine J 22:2249–55. doi: 10.1007/s00586-013-2886-2.
- Mitsui R, Matsukawa M, Nakagawa K, et al. (2021). Efficient cell transplantation combining injectable hydrogels with control release of growth factors. Regen Ther 18:372–83. doi: 10.1016/j.reth.2021.09.003.
- Morhard R, Mueller JL, Tang Q, et al. (2020). Understanding factors governing distribution volume of ethyl cellulose-ethanol to optimize ablative therapy in the liver. IEEE Trans Biomed Eng 67:2337–48. doi: 10.1109/TBME.2019.2960049.
- Morhard R, Nief C, Barrero Castedo C, et al. (2017). Development of enhanced ethanol ablation as an alternative to surgery in treatment of superficial solid tumors. Sci Rep 7:8750. doi: 10.1038/s41598-017-09371-2.
- Muñoz NM, Williams M, Dixon K, et al. (2021). Influence of injection technique, drug formulation and tumor microenvironment on intratumoral immunotherapy delivery and efficacy. J Immunother Cancer 9:e001800. doi: 10.1136/jitc-2020-001800.
- Nahar M, Mishra D, Dubey V, Jain NK. (2008). Development, characterization, and toxicity evaluation of amphotericin B-loaded gelatin nanoparticles. Nanomedicine 4:252–61. doi: 10.1016/j.nano.2008.03.007.
- Nii T. (2021). Strategies using gelatin microparticles for regenerative therapy and drug screening applications. Molecules 26:6795. doi: 10.3390/molecules26226795.
- Park S, Kim J, Lee C. (2022). Injectable rapidly dissolving needle-type gelatin implant capable of delivering high concentrations of H2O2 through intratumoral injection. Biomed Pharmacother 156:113910. doi: 10.1016/j.biopha.2022.113910.
- Præstmark KA, Stallknecht B, Jensen ML, et al. (2016). Injection technique and pen needle design affect leakage from skin after subcutaneous injections. J Diabetes Sci Technol 10:914–22. doi: 10.1177/1932296815626723.
- Premat K, Clarençon F, Cormier É, et al. (2017). Long-term outcome of percutaneous alcohol embolization combined with percutaneous vertebroplasty in aggressive vertebral hemangiomas with epidural extension. Eur Radiol 27:2860–7. doi: 10.1007/s00330-016-4664-0.
- Ryu M, Shimamura Y, Kinoshita T, et al. (1997). Therapeutic results of resection, transcatheter arterial embolization and percutaneous transhepatic ethanol injection in 3225 patients with hepatocellular carcinoma: a retrospective multicenter study. Jpn J Clin Oncol 27:251–7. doi: 10.1093/jjco/27.4.251.
- Schmidt R, Cakir B, Mattes T, et al. (2005). Cement leakage during vertebroplasty: an underestimated problem? Eur Spine J 14:466–73. doi: 10.1007/s00586-004-0839-5.
- Seki T, Nonaka T, Kubota Y, et al. (1989). Ultrasonically guided percutaneous ethanol injection therapy for hepatocellular carcinoma. Am J Gastroenterol 84:1400–7.
- Sheth RA, Murthy R, Hong DS, et al. (2020). Assessment of image-guided intratumoral delivery of immunotherapeutics in patients with cancer. JAMA Netw Open 3:e207911. doi: 10.1001/jamanetworkopen.2020.7911.
- Shiina S, Tagawa K, Unuma T, et al. (1991). Percutaneous ethanol injection therapy for hepatocellular carcinoma. A histopathologic study. Cancer 68:1524–30. doi: 10.1002/1097-0142(19911001)68:7<1524::AID-CNCR2820680711>3.0.CO;2-O.
- Shokry M, Hathout RM, Mansour S. (2018). Exploring gelatin nanoparticles as novel nanocarriers for Timolol Maleate: augmented in-vivo efficacy and safe histological profile. Int J Pharm 545:229–39. doi: 10.1016/j.ijpharm.2018.04.059.
- Tamura H, Goto R, Akune Y, et al. (2015). the clinical effectiveness and cost-effectiveness of screening for age-related macular degeneration in Japan: a Markov modeling study. PLOS One 10:e0133628. doi: 10.1371/journal.pone.0133628.
- Walker C, Thelen J. Livestock Injection methods & placement, ANR Communications and Marketing ((2016). http://anrcom.msu.edu/) for MSU Extension (http://msue.anr.msu.edu/).
- Will RG, Ironside JW, Zeidler M, et al. (1996). A new variant of Creutzfeldt-Jakob disease in the UK. Lancet 347:921–5. doi: 10.1016/s0140-6736(96)91412-9.
- Wilson DJ, Neuringer M, Stoddard J, et al. (2017). Subretinal cell-based therapy: an analysis of surgical variables to increase cell survival. Retina 37:2162–6. doi: 10.1097/IAE.0000000000001462.
- Xu W, Lan Z, Huang Y. (2020). Intraoperative injection of absorbable gelatin sponge (AGS) mixed with cement followed by spinal decompression to treat elderly with vertebral hemangiomas. BMC Musculoskelet Disord 21:125. doi: 10.1186/s12891-020-3143-6.
- Young S, Wong M, Tabata Y, Mikos AG. (2005). Gelatin as a delivery vehicle for the controlled release of bioactive molecules. J Control Release 109:256–74. doi: 10.1016/j.jconrel.2005.09.023.