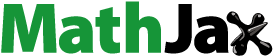
ABSTRACT
The objectives of this study are to clarify the relative importance of sink and related factors of dry matter and water use pre-and post-heading in four millet species under severe water deficit. Panicum miliaceum, Panicum sumatrense, Setaria glauca., and S. italica were used. Water deficit (20% soil water content) was imposed from 25 d after sowing until harvest, with 40% soil water as a control. At the heading, half of the leaf area or all leaves on each plant were removed in both the control and the water deficit treatment (Source manipulation). Water deficit treatment markedly decreased the leaf water potential of all species from −2.02 MPa (S. italica) to −1.72MPa (P. miliaceum). The grain yields of water deficit treatment in S. italica, P. miliaceum, S. glauca and P. sumatrense decreased to 62, 48, 31, and 24% of the control, respectively. Four millet species were divided to dehydration-tolerant millet (S. italica and P. miliaceum) and dehydration-susceptible millet (S. glauca and P. sumatrense) by calculating drought susceptibility index. The interspecific difference in dehydration tolerance was accounted for by the number of grains per panicle and the harvest index. The source operation showed that the sink was more important than the source. In conclusion, under severe soil drying, it was considered that S. italica and P. miliaceum showed strong dehydration tolerance because they maintained the number of grains per panicle and did not reduce dry matter production and the partitioning of dry matter to the panicle after heading.
Abbreviations: CLA0: all leaves were removed at heading in the control; CLA0.5: half of the leaf area per plant was removed at heading in the control; DAS: days after sowing; DLA0: all leaves were removed at heading in the dehydration treatment; DLA0.5: half of the leaf area was removed at heading in the dry treatment; DSI: drought susceptibility index; NSC: nonstructural carbohydrate; OA: osmotic adjustment; WUE: water use efficiency.
Graphical abstract
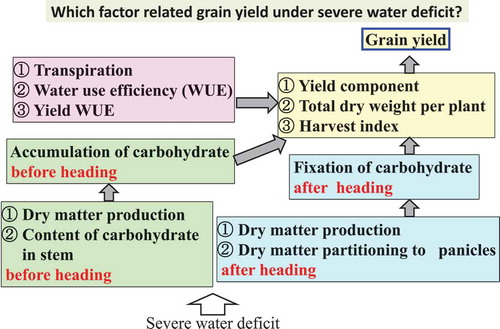
Millets are known for their climate-resilient features including adaptation to a wide range of ecological conditions, less irrigational requirements, better growth and productivity in low nutrient input conditions, less reliance on synthetic fertilizers, and minimum vulnerability to environmental stresses (Bandyopadhyay et al., Citation2017; Berg et al., Citation2013; Kole et al., Citation2015). Foxtail millet (Setaria italica) is an annual crop that originated from S. viridis. S. italica is one of the most important food and foliage crops in semi-arid areas (Wen et al., Citation2012) because of thick cell walls, a dense root structure, small leaf area (Liu et al., Citation2019). The mean grain yield of S. italica is approximately 0.150 kg m−2 and is mainly cultured as a healthy food on the hilly and mountainous areas in Japan. Yellow foxtail millet (S. glauca) is an annual weed that grows along the roadside and elsewhere; however, it is an important food crop that is often cultivated with little millet (P. sumatrense) in South India (Kimata et al., Citation2000). During severe drought, farmers in India believe that S. glauca provides a reasonable harvest, whereas P. sumatrense might fail completely (Kimata et al., Citation2000). Common millet (P. miliaceum) is one of the early-maturing millets and its drought tolerance is well known. The grain yield of P. miliaceum is approximately 0.120 kg m−2 and is mainly cultivated as a healthy food on the hilly and mountainous areas in Japan. P. miliaceum is also suited to dryland production in the semi-arid area due to its low water and nutrient requirement, short growing season and highly efficient C4 photosynthesis (Baltensperger, Citation1996; Kole et al., Citation2015; Lyon et al., Citation2008; Rajput et al., Citation2016).
Recently, mechanisms of drought tolerance in foxtail millet and common millet was reported and S. italica had higher antioxidant potential than P. miliaceum (Nematpour et al., Citation2019). However, there was not significant difference the decrease of the grain yield between S. italica and P. miliaceum (47% and 45% of the control). Furthermore, there were not significant interspecific differences of antioxidant enzyme activities and also carotenoids. Alleviation of leaf senescence played an important role in enhancing the yield of S. italica in the rain-fed area (Dai et al., Citation2012). However, grain yield did not coincide with the photosynthetic rate, chlorophyll content but also superoxide radicals and hydrogen peroxide content. Similarly, fragmental drought resistance mechanisms have been reported (Dai et al., Citation2011; Karyudi & Fletcher, Citation2002; Zhang et al., Citation2012). Many drought tolerance genes were also reported (Feng et al., Citation2015; Lata & Prasad, Citation2012; Wang et al., Citation2018), however, they are PEG-simulated drought stress which is essentially different from natural soil drought events (Tang et al., Citation2017). Bernier et al. (Citation2009) also pointed out a lack of understanding of the traits and mechanisms of drought tolerance (Bernier et al., Citation2009). Passioura (Citation2006) also pointed out that several investigations into water deficit by plant physiologists, biochemists, and molecular biologists were more concerned with survival than production. From these results, it was considered necessary to clarify the factors that determine the yield in order to clarify the dehydration tolerance mechanism of millets. Rice (Oryza sativa L.) plants transformed with the phosphoenolpyruvate carboxylase enzyme from foxtail millet showed an increase in the phosphoenolpyruvate carboxylase activity, net photosynthesis, and grain yield under drought conditions (Ding et al., Citation2013). As in that study, elucidating the mechanism of dehydration tolerance in millet may be also useful for other crop applications.
The increase in grain yield must be achieved in a sustainable way, overcoming the yield losses caused by environmental stresses but without increasing demand for resources (arable land, irrigation water, and fertilizers) (Albacete et al., Citation2014). Improving crop production in environments that experience severe drought is important for supplying food to the increasing global population under changing environmental conditions. Breeding drought-tolerant crops is one way to increase grain yield. Sustaining crop productivity under unfavorable conditions necessarily requires crop plants to maintain both assimilate production in source tissues and assimilate transport to and use within sinks and harvestable tissues (Albacete et al., Citation2014). In cereals, grains are the most active sinks for C and N assimilates after flowering, and most carbohydrates are provided by current photosynthesis during grain filling (Wardlaw, Citation1990). The use and supply of carbohydrates are considered as adjustment between sources and sinks. Artificial reduction of sources or sinks has been applied to provide more evidence whether grain yield in cereals is source- or sink-limited (Bingham et al., Citation2007; Blum, Mayer & Golan, Citation1988; Tanaka & Fujita, Citation1971). Based on the idea that sink capacity can be defined as the maximum size of the harvesting sink organ, the term ‘yield sink capacity’ was adopted to distinguish it from grain sinks and panicle sinks. Since ‘yield sink capacity’ multiplied by filling efficiency leads to an estimate for actual yield, the yield sink capacity has been recognized as a primary objective in crop breeding for increasing yield (Kato & Takeda, Citation1996).
Carbohydrate remobilization and osmotic adjustment are associated with crop production under water-limited conditions (Blum, Citation2005). Carbon supply in cereal kernels at maturity depends both on the photosynthates produced during grain filling and on the remobilization of assimilates from vegetative tissues (Schnyder, Citation1993). The storage of water-soluble carbohydrates in the stem of small-grain cereals and their subsequent remobilization to grain can directly influence the harvest index, especially after anthesis (Reynolds & Tuberosa, Citation2008). High osmoregulation variety showed high grain yield and there were significant correlations between grain yield, grain number and harvest index and osmoregulation at mild stress (the leaf water potential was around −0.80 MPa) in S. italica. (Karyudi & Fletcher, Citation2003). However, the contribution of remobilization and accumulation of carbohydrate and osmotic adjustment to grain yield under severe water deficit is unclear in millets.
In a drought-prone environment, the grain yield is accounted for by water uptake, water use efficiency (WUE), and harvest index (Reynolds & Tuberosa, Citation2008). WUE is defined as the ratio of C assimilation or biomass accumulation to transpiration, and it determines plant growth in water-limited environments (Vadez et al., Citation2014). WUE is determined by many factors, including photosynthetic carbon assimilated per unit of water transpired (Condon et al., Citation2002; Farquhar et al., Citation1989) and partitioning of photo assimilates (Carmo-Silva et al., Citation2009; Chaves, Citation1991). WUE is considered to be one of the key features for successful wheat (Triticum aestivum L.) growth (Condon et al., Citation2004). Recently, certain WUE-related traits have been identified in Arabidopsis thaliana (L.) Heynh. (Meng & Yao, Citation2015; Ruggiero et al., Citation2017). WUE of S. italica and P. miliaceum did not change with 50% depletion of shoot dry weight by soil water deficit in small pot experiment at heading (Zegada-Lizarazu & Iijima, Citation2005). WUE of S. italica did not change during 15days water deficit in small pot experiment with 22% depletion of seedling biomass (Xu et al., Citation2006). However, the relationship between yield and WUE is unknown. Water deficit leads directly or indirectly to low photosynthetic rates and, in turn, a decrease in overall C supplies (Chaves, Citation1991; Hsiao, Citation1973). As well as C, effectively water use is also important to keep high grain yield under severe water deficit. The objectives of this study are to clarify the relative importance of sink and related factors of dry matter and water use pre-and post-heading in four millet species under severe water deficit.
Materials and methods
Plant materials and growth conditions
In this study, we used four millet species, common millet (Panicum miliaceum cv. Higashi), foxtail millet (S. italica cv. 84-6-14-2), little millet (P. sumatrense cv. 97-4-12-2-1), and yellow foxtail millet (S. glauca cv. 97-4-12-2-2). The five seeds of each species were sown in a Wagner pot (0.02 m−2 surface area; 17.5 cm in inner diameter; 20 cm in height) containing 3.00 kg soil with 40% water content (C; the control) in a greenhouse at Tokai University, Kumamoto, Japan, on June 12 in 2009. A chemical fertilizer (4 g) with an N:P:K content of 8:8:8% and dolomitic lime (2.4 g) at 100g m−2 was added to all pots before sowing. The water deficit treatment was initiated at 25 d after sowing (DAS) by providing 20% soil water content. The pots were watered at three times per week and weighed before and after watering for calculating transpiration.
Source manipulation
At the onset of heading (S. italica: 73 and 82 DAS for the control and treatment, respectively; S. glauca: 75 and 95 DAS, respectively; P. miliaceum: 67 DAS for the control same as treatment; P. sumatrense: 87 and 110 DAS, respectively), one-third of the pots under the control and the dehydration treatment were subjected to the removal of a half of the leaf area of each plant (referred to as CLA0.5 for the control and DLA0.5 for the dehydration treatment) until harvest.
At the same time, one-third of the pots under the control and the dehydration treatments were subjected to the removal of all leaves (referred to as CLA0 for the control and DLA0 for the dehydration treatment); the other pots under the control and the dehydration treatments continued normal growth until harvest. The leaf blades were removed when they were fully expanded.
Dry matter production and grain yield
Six plants were sampled for each treatment 1 d before imposing a treatment, at heading, and at harvest. Leaf blade, leaf sheath, and stem samples were collected and cured at 110°C for 30 min, followed by drying at 65°C for 72 h and weighing. The root samples were washed free from soil and dried as the shoots to be used for the measurement of dry weight. The drought susceptibility index (DSI) was calculated as follows (Fisher & Maurer, Citation1978):
where GYD is the grain yield of each millet species in the dehydration treatment, GYC is the grain yield of each millet species in the control, and GYCM and GYDM are the mean grain yields of all millet species in the control and the dehydration treatments, respectively.
The increases in total dry weight (ΔTDW1 and ΔTDW2 for the start of the water deficit to heading and till harvest from heading, respectively; in g) and panicle weight till harvest from heading (ΔP, in g) were calculated via the following equations:
where W1 is the dry weight of the whole plant on the day when the water deficit treatment began, W2 and P1 are the dry weights of the whole plant and panicle on the day heading started, and W3 and P2 are the dry weights of the whole plant and panicle at harvesting, respectively. The dry matter partitioning ratios of the stem and panicle (DMPRs and DMPRp, respectively) were calculated via the following equations:
where S2 is the dry weight of the stem and leaf sheath on the day when heading started, S3 is the dry weight of the stem and leaf sheath at harvesting, and W2 and W3 are as defined for Equation (2–4).
The grain yield and yield components (i.e. the number of panicles per plant, number of grains per panicle, and 1000-grain weight, were determined in the six harvested plants for each treatment. The harvest index was calculated as the ratio of the seed dry weight to the total dry weight. The transpiration per plant was calculated as the decrease in the weight of a pot from heading to harvest. The WUE was calculated as the total dry weight divided by transpiration from heading to harvest. The yield WUE was calculated as the grain yield divided by transpiration from heading to harvest.
Water potential and osmotic potential of leaves
The water potential of the second fully expanded leaf was measured in four leaves per treatment in a pressure chamber (model 600, PMS Inc., Corvallis, US-OR, USA) at midday. Sample leaves were then put into a sealed vinyl bag containing a small amount of distilled water at 20 d after the water deficit treatment was imposed. The leaves were incubated at 10°C for 24 h, after which they became turgid and were wrapped with an aluminum foil and then submerged in liquid nitrogen. The leaves were kept in a freezer at −80°C until they were used for the measurement of osmotic potential with a thermocouple psychrometer (Tru Psi model SC10X, Decagon Devices Inc., Pullman, US-WA, USA). The osmotic adjustment (OA) of leaves was calculated as the difference in the osmotic potential between the control and water deficit treatments (Pantuwan et al., Citation2002).
Measurement of nonstructural carbohydrates
The stem, including leaf sheath, was sampled from all millet species in each treatment at heading and dried, and then being ground in a mill. For each sample, 500 mg powder was used for sugar and starch analysis (Parida et al., Citation2002; Tsuchida et al., Citation2011). The sample powders were extracted three times with 80% ethanol by boiling the samples in Erlenmeyer flasks capped with rubber stoppers and protruding glass tubes in a 50°C water bath for 10 min each. Subsequently, the extracted solution was centrifuged at 1000g for 5 min, and the supernatants of the three extractions were combined. The supernatant was evaporated to remove ethanol, followed by the addition of distilled water. To the supernatant solution, 4.7 ml of 0.3 N Ba(OH)2 and 5 ml of 5% ZnSO4 · 7H2O was added before filtration. Finally, 100 mL of the sample solution was prepared for sugar analysis. The residues remaining in the flasks were used for starch analysis. The residues, moved to a test tube, were boiled at 50°C to remove ethanol, followed by the addition of distilled water before boiling at approximately 100°C for 15 min. Next, 6.5 mL of 3.5 N HClO4 was added and stirred for 20 min. The tube was centrifuged at 3000 rpm for 5 min and the supernatant was moved to a 100-mL volumetric flask. The residues were mixed with 5 mL of distilled water and 6.5 mL of 3.5 N HClO4 then boiled at 100°C for 15 min. The supernatants were combined and the final volume was brought up to 100 mL. For both extractions, the sugar concentrations in the extracts were determined against a glucose standard via the phenol-sulfuric acid method described by Dubois et al. (Citation1956). The sugar and starch contents were combined as the nonstructural carbohydrate (NSC) content. Each treatment was replicated six times.
Statistical analysis
The pots were arranged in a completely randomized design, with three replicates. The data were analyzed via a two-way ANOVA and the treatments means were compared via the Tukey-Kramer multiple range test or t-test. A regression analysis was also performed to explore the relationships among grain yield and yield component, total dry weight, and harvest index.
Results
Grain yield and yield component
The grain yields of S. italica and P. miliaceum decreased to 62 and 48% of the control under the water deficit treatment and those of S. glauca and P. sumatrense decreased markedly to 31 and 24% of the control, respectively (). The DSI of S. italica and P. miliaceum were significantly lower than those of S. glauca and P. sumatrense (), indicating that S. italica and P. miliaceum were more dehydration-tolerant than S. glauca and P. sumatrense. Panicle number and 1000-grain weight in all millet species did not change under the water deficit treatment. However, under the water deficit treatment, the grain number per panicle in S. italica and P. miliaceum millet decreased to 75 and 70% of the control; in S. glauca and P. sumatrense, it decreased markedly to 33 and 38%. The total dry weight of S. italica, P. miliaceum, S. glauca and P. sumatrense under the water deficit treatment decreased to 68, 45, 53 and 45% of the control. Although the harvest index of S. italica and P. miliaceum did not change under the water deficit treatment, that of S. glauca and P. sumatrense decreased significantly. Significant correlations existed among the grain number per panicle, total dry weight, harvest index and grain yield in all millet species. ().
Table 1. Influence of the water deficit on grain yield and yield components in four millet species.
Figure 1. Relationships between grain yield and yield components in four millet species.
** and * indicate significant correlations at 1 and 5%, respectively. NS, no significant correlation.
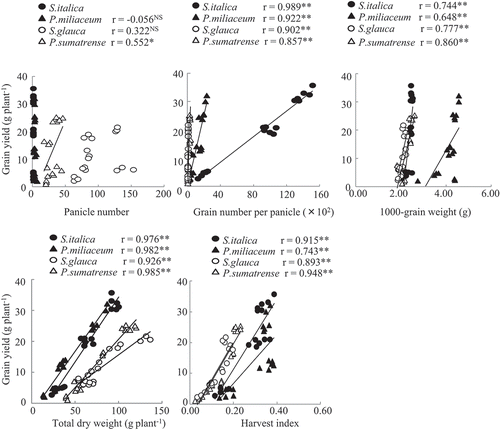
When a half of the leaf area per plant was removed at heading, the grain yield did not change between the control and the dehydration treatment in all millet species (). When all lea3ves were removed at heading, the grain yield of CLA0 in S. italica and P. miliaceum was markedly decreased to 16% of the control (). The grain yield of DLA0 in S. italica and P. miliaceum was also markedly decreased to 20 and 17%, respectively. The grain yield of CLA0 in S. glauca and P. sumatrense was decreased to 56 and 66% of the control, respectively. The grain yield of DLA0 in S. glauca and P. sumatrense also decreased to 45 and 29%, respectively. From these results, it was shown that in two millet species with low dehydration tolerance, the grain yield was reduced by leaf removing under the dehydration treatment.
Leaf water potential
The leaf water potential decreased to between −1.72 MPa (P. miliaceum) and −2.02 MPa (S. italica) under the water deficit treatment in all four millet species (). The osmotic potential at full turgor of S. glauca decreased under the water deficit treatment but did not change in other millets. The OA of the leaf was 0.06 MPa in S. italica, 0.20 MPa in P. miliaceum, 0.45 MPa in S. glauca, and 0.28 MPa in P. sumatrense. The OA was observed only in S. glauca and it was not consistent with the interspecific differences in the dehydration tolerance of millets.
Table 2. Effects of the dehydration treatment on leaf water potential (ΨL), leaf osmotic potential (πL) at full turgor, and leaf osmotic adjustment (OA) at 20 d after the initiation of the drought treatment.
Transpiration, WUE, and yield WUE
Total transpiration and the transpiration till heading decreased under the water deficit treatment in all millet species, except for S. glauca till heading, and no interspecific difference was observed that reflected the differences in dehydration tolerance (). The transpiration from heading to harvest decreased under the water deficit treatment in all four millet species, and those interspecific difference was tended to consistent with the differences in dehydration tolerance. The WUE from the initiation of water deficit treatment to heading in P. miliaceum, P. sumatrense and S. italica during the treatment to heading increased to 120 and 117% of the control under the water deficit treatment but WUE decreased to 67% in S. glauca (). The WUE from heading to harvest in S. italica and P. sumatrense increased to 127% of the control under the water deficit treatment but WUE did not change in P. miliaceum and S. glauca. Total WUE in all millet species from the treatment to harvest did not changed by the water stress. Any WUE did not consistent with the difference in dehydration tolerance. The yield WUE from heading to harvest increased to 121 and 111% of the control under the water deficit treatment in S. italica and P. miliaceum but decreased to 78 and 80% in S. glauca and P. sumatrense, respectively (). This was consistent with the interspecies difference in dehydration tolerance.
Table 3. Influence of the dehydration treatment on transpiration, water use efficiency (WUE) and yield WUE in four millet species.
Accumulation and partitioning of dry matter
The water deficit treatment reduced the increase in total dry weight (ΔTDW1) by 37 to 77% compared with the control in all millet species, except for P. sumatrense during the water deficit treatment started to heading (). The water deficit treatment also decreased the increase in total dry weight (ΔTDW2) of S. italica, P. miliaceum, S. glauca and P. sumatrense to 65, 47, 39 and 38% of the control, respectively, between heading to harvest. The increases in whole-plant dry weight pre-heading were not consistent with interspecific differences in the dehydration tolerance of millets. However, ΔTDW2 were consistent with interspecific differences in the dehydration tolerance of millets. The water deficit treatment increased the NSC content of stems containing leaf sheaths in S. italica and P. miliaceum to 152 and 127%, respectively (). Whereas the NSC content in S. glauca and P. sumatrense did not change between the control and dehydration treatment.
Table 4. Influence of the dehydration treatment on the NSC content of the stem, including leaf sheath, the increase in total dry weight (ΔTDW), the increase in the panicle dry weight (ΔP), the dry matter partitioning ratio (DMPR) in four millet species.
The water deficit decreased the increase in the dry weight of panicles (ΔP) of S. italica, P. miliaceum, S. glauca and P. sumatrense to 65, 47, 31 and 31% of the control, respectively (). The ΔP were consistent with interspecific differences in the dehydration tolerance of millets. The water deficit treatment decreased dry matter partitioning to the stem in S. italica and P. miliaceum but not in S. glauca and P. sumatrense (). Dry matter partitioning to the panicle was not affected by the water deficit treatment in S. italica and P. miliaceum but showed a decrease in S. glauca and P. sumatrense. Both of these factors were consistent with interspecific differences in the dehydration tolerance of millets.
Discussion
Grain yield and relative importance of sinks
The grain yield under water stress might depend on both yield potential (grain yield without water stress) and susceptibility to water stress (Fisher & Maurer, Citation1978). The latter was defined as the DSI by Fisher and Maurer (Citation1978) and can be used for making comparisons between drought levels and experiments. The DSI has been widely used to classify sensitive and resistant genotypes and appears to be a suitable selection index for distinguishing resistant cultivars because it is inversely correlated with grain yield under stress (Fernandez, Citation1992; Matsuura et al., Citation2012; Sio-Se Mardeh et al., Citation2006). The yield potential might partly contribute to the interspecific differences in dehydration tolerance among the four millet species in our experiment because of a significant correlation between the grain yields of the control and those of the dehydration treatment. The DSI showed that S. italica and P. miliaceum were more dehydration-tolerant than S. glauca and P. sumatrense.
Grain yield is calculated as ‘yield sink capacity’ x ‘filling efficiency’ (Kato & Takeda, Citation1996). Yield sink capacity consists of the number of spikes per plant, the number of spike flowers per spike, and the full grain weight (Kato & Takeda, Citation1996). The dehydration tolerance in this experiment was mainly caused by the number of grains per panicle, as was the case for rice discovered by Kato and Takeda (Citation1996). In addition, the ‘filling efficiency’ was considered to make some contribution to the interspecific differences in dehydration tolerance because there was a significant correlation between grain yield and harvest index. ( and ).
When a half of the leaf area was removed from each plant, the grain yield did not change in either the control or the dehydration treatment in all millet species (). This result showed that the grain yield was mainly sink-limited. Tanaka and Fujita (Citation1971) also reported that restricting the source by removing several leaves enhanced the productivity of the remaining leaves and concluded that the sink size controls the grain yield in maize (Zea mays L.). Results of removed all leaves suggests that contribution of photosynthesis after heading to the grain yield did not change in S. italica and P. miliaceum (). On the other hand, contribution of photosynthesis after heading to the grain yield increased by the dehydration stress from 44% to 55 and 34% to 71% in S. glauca and P. sumatrense, respectively. Supply of carbohydrate after heading was more important in dehydration-susceptible millets.
Leaf water potential under severe water deficit
The water deficit treatment decreased the leaf water potential in all millet species under severe stress in this experiment (). From these results, it was considered that the four millet species were subjected to severe water stress similarly. It has been reported that the grain number decreases because of ovary abortion in maize, or pollen sterility in wheat, barley (Hordeum vulgare L.), and rice at low leaf water potential under drought stress (Boyer & Westgate, Citation2004). In this experiment as well, it was considered that the grain number per panicle was reduced by the severe water stress observed in the decrease in leaf water potential. Osmotic adjustment helps to maintain photosynthesis by keeping the pressure potential of cells constant when the leaf water potential falls. It has been reported that the high-osmoregulation group produced 1.58 times more grain yield and harvest index than the low-osmoregulation group in S. italica at −0.8 MPa of leaf water potential. (Karyudi & Fletcher, Citation2003). OA of high-osmoregulation group and low-osmoregulation group were 1.22 and 0.87 MPa, respectively. However, OA of millet species were between 0.06 and 0.45 MPa at −1.72 and −2.02 MPa of leaf water potential in this experiment. These results suggest that the strength of water deficit was different between experiments. Furthermore, no relationship between the dehydration tolerance of millet species and OA was found in this experiment.
Factors in relation to water use affecting dehydration tolerance
Generally, there is a positive correlation between water absorption and dry matter production. In this experiment, the amount of transpiration from heading to harvest and the dehydration tolerance tended to be consistent (). The amount of transpiration from heading to harvest of two species of Setaria was higher than that of two species of Panicum, and it was thought that the fine roots were densely developed as reported previously (Liu et al., Citation2019; Matsuura et al., Citation2012). The amount of transpiration after heading was clearly higher than that before heading, and it decreased greatly by water deficit treatment. This result was similar for pearl millet (Vadez et al., Citation2013).
During the ripening period, it is important for plants to use water efficiently during severe water shortages. When the plants were grown in a small pot 25 days after sowing, were subjected with water deficiency for about 1 month, WUE of the well-watered S.italica and P.miliaceum were 2.73 g L−1 and 2.88 g L−1, respectively, and WUE in the dry treatment were 2.59 g L−1 and 2.95 g L−1, which did not change significantly by the water deficit treatment (Zegada-Lizarazu & Iijima, Citation2005). When seedlings were grown in a small pot and subjected 15 days of water deficit treatment, WUE of well-watered and dehydration treatment in S.italica were 8.67g L−1 and 8.11 g L−1, respectively, those were not significant difference (Xu et al., Citation2006). In our experiment, WUE did not change by the dehydration treatment in all millet species (). This suggest that WUE was not a factor related of dehydration tolerance. The interspecies differences in yield WUE and dehydration tolerance were consistent in our experiment.
The interspecies differences in yield WUE and dehydration tolerance were consistent in our experiment. Similar to our results, it was reported that the yield WUE of dehydration-tolerant variety was higher than the dehydration susceptible variety and there was no varietal difference of WUE in wheat (Thapa et al., Citation2018). Also, the high yield WUE values of maize were also associated with their high grain yields, resulting from the high number of grains per plant (Cai et al., Citation2017; Nagore et al., Citation2017).
Factors in relation to dry matter affecting dehydration tolerance
In this experiment, the interspecific difference of NSC, ΔTDW2, ΔP, DMPRS and DMPRP were consisted with the dehydration tolerance of four millet species. (). The only effective mechanism of drought tolerance in crops is utilization of accumulated photosynthates in the stem for grain filling under drought stress (Blum, Citation1998). Water stress that reduces plant water status and photosynthesis during grain filing induces the conversion of stem storage into soluble sugars and the mobilization of sugars into grains (Blum, Citation2005). Our results indicated that the NSC content of the dehydration-tolerant millet species increased under the dehydration treatment, whereas that of the dehydration-susceptible millet species did not change. This result agrees with the result of Fu et al. (Citation2011), who reported that the NSC reserves in the stem before anthesis are closely associated with sink strength during grain filling in rice. The storage of water-soluble carbohydrates in the stem of small-grain cereals and their subsequent remobilization to grain can directly influence the harvest index, especially under stress after anthesis (Reynolds & Tuberosa, Citation2008).
In this experiment, the two dehydration-tolerant millet species did not change in ΔP and total dry weight at ΔP of the dehydration treatment in dehydration-tolerant millet species decreased to 47 and 65% of the control whereas those of dehydration-susceptible millet species markedly decreased to 31% of the control (). These reduction of ΔP coincided with the decrease of ΔTDW2 in this experiment (). Xu et al. (Citation1997) also reported that the high-yielding rice cultivar showed a high harvest index, an increase in dry matter after heading, and dry matter partitioning to panicles. A significant correlation between the loss in stem and leaf sheath weight and grain yield have been reported during grain filling in barley (Richards et al., Citation2002). This was similar results of DMPRS and DMPRP in our experiment ().
In conclusion, under severe soil drying, it was considered that S. italica and P. miliaceum showed strong dehydration tolerance because they maintained the number of grains per panicle and did not reduce dry matter production and the partitioning of dry matter to the panicle after heading.
Acknowledgments
We also thank Mr. T. Yuki and Mr. K. Mizuma, students of the Department of Agriculture, Tokai University, for their assistance in carrying out the experiments.
Disclosure statement
No potential conflict of interest was reported by the authors.
Additional information
Funding
References
- Albacete, A., Martinez-Andujar, C., & Perez-Alfocea, F. (2014). Hormonal and metabolic regulation of source–Sink relations under salinity and drought: From plant survival to crop yield stability. Biotechnology Advances, 32, 12–30.
- Baltensperger, D. D. (1996). Foxtail and proso millet. In J. Janick (Ed.), Progress in new crops (pp. 182–190). Alexandria, VA: ASHS Press.
- Bandyopadhyay, T., Muthamilarasan, M., & Prasad, M. (2017). Millets for next generation climate-smart agriculture. Frontiers in Plant Science, 8, 1266.
- Berg, A., Noblet-Ducoudre, N., Sultan, B., Lengaigne, M., & Guimberteau, M. (2013). Projections of climate change impacts on potential C4 crop productivity over tropical regions. Agricultural and Forest Meteorology, 170, 89–102.
- Bernier, J., Serraj, R., Kumar, A., Venuprasad, R., Impa, S., Veeresh Gowda, R. P., … Atlin, G. (2009). The large-effect drought-resistance QTL qtl12.1 increase water uptake in upland rice. Field Crops Research, 110, 139–146.
- Bingham, J. I., Blake, J., Foulikes, M. J., & Spink, J. (2007). Is barley yield in the UK sink limited? I. Post-anthesis radiation interception, radiation-use efficiency and source–Sink balance. Field Crops Research, 101, 198–211.
- Blum, A. (1998). Improving wheat grain filling under stress by stem reserve mobilization. Euphytica, 100, 77–83.
- Blum, A. (2005). Drought resistance, water-use efficiency, and yield potential—Are they compatible, dissonant, or mutually exclusive? Australian Journal of Agricultural Research, 56, 1159–1168.
- Blum, A., Mayer, J., & Golan, C. (1988). The effect of grain number per ear (sink size) on source activity and its water-relations in wheat. Journal of Experimental Botany, 39, 106–114.
- Boyer, J. S., & Westgate, M. E. (2004). Grain yields with limited water. Journal of Experimental Botany, 55, 2385–2394.
- Cai, Q., Zhang, Y., Sun, Z., Zheng, J., Bai, W., Zhang, Y., & Zhang, L. (2017). Morphological plasticity of root growth under mild water stress increases water use efficiency without reducing yield in maize. Biogeosciences, 14, 3851–3858.
- Carmo-Silva, A. E., Francisco, A., Powers, S. J., Keys, A. J., Ascensão, L., Parry, M. A. J., & Arrabaça, M. C. (2009). Grasses of different C4 subtypes reveal leaf traits related to drought tolerance in their natural habitats: Changes in structure, water potential, and amino acid content. American Journal of Botany, 96, 1222–1235.
- Chaves, M. M. (1991). Effect of water stress on carbon assimilation. Journal of Experimental Botany, 42, 1–16.
- Condon, A. G., Richards, R. A., Rebetzke, G. J., & Farquhar, G. D. (2002). Improving intrinsic water-use efficiency and crop yield. Crop Science, 42, 122–131.
- Condon, A. G., Richards, R. A., Rebetzke, G. J., & Farquhar, G. D. (2004). Breeding for high water-use efficiency. Journal of Experimental Botany, 55, 2447–2460.
- Dai, H. P., Jia, G. L., Lu, C., Wei, A. Z., Feng, B. L., & Zhang, S. W. (2011). Studies of synergism between root system and leaves senescence in broomcorn millet (Panicum miliaceum L.). Journal of Food Agriculture and Environment, 9, 177–180.
- Dai, H. P., Shan, C. J., Wei, A. Z., Yang, T., Sa, W. Q., & Feng, B. L. (2012). Leaf senescence and photosynthesis in foxtail millet [Setaria italica (L.) P. Beauv] varieties exposed to drought conditions. Australian Journal of Crop Science, 6, 232–237.
- Ding, Z. S., Huang, S. H., Zhou, B. Y., Sun, X. F., & Zhao, M. (2013). Over-expression of phosphoenolpyruvate carboxylase cDNA from C4 millet (Setaria italica) increases rice photosynthesis and yield under upland condition but not in wetland fields. Plant Biotechnology Reports, 7, 155–163.
- Dubois, M., Gilles, K. A., Hamilton, J. K., Rebers, P. A., & Smith, F. (1956). Colorimetric method for determination of sugars and related substances. Analytical Chemistry, 28, 350–356.
- Farquhar, G. D., Hubick, K. T., Condon, A. G., & Richards, R. A. (1989). Carbon isotope fractionation and plant water-use efficiency. In P. W. Rundel, J. R. Ehleringer, & K. A. Nagy (Eds.), Stable isotopes in ecological research (pp. 21–40). New York, NY: Springer.
- Feng, Z. J., He, G. H., Zheng, W. J., Lu, P. P., Chen, M., Gong, Y. M., & Xu, Z. S. (2015). Foxtail millet NF-Y families: Genome-wide survey and evolution analyses identified two functional genes important in abiotic stresses. Frontiers in Plant Science, 6, 1142.
- Fernandez, G. C. J. (1992, August 13–18). Effective selection criteria for assessing stress tolerance. In C. G. Kuo, editor, Proceedings of the international symposium on adaptation of food crops to temperature and water stress (pp. 257–270), Taiwan. Shanhua, Tainan: Asian Vegetable Research and Development Center Publication.
- Fisher, R. A., & Maurer, R. (1978). Drought resistance in spring wheat cultivars. C. Grain yield responses. Australian Journal of Agricultural Research, 29, 897–912.
- Fu, J., Huang, Z., Wang, Z., Yang, J., & Zhang, J. (2011). Pre-anthesis non-structural carbohydrate reserve in the stem enhances the sink strength of inferior spikelets during grain filling of rice. Field Crops Research, 123, 170–182.
- Hsiao, T. C. (1973). Plant responses to stress. Annual Review of Plant Physiology, 24, 519–570.
- Karyudi, D., & Fletcher, R. J. (2002). Osmoregulative capacity in birdseed millet under conditions of water stress. I. variation in Setaria italica and Panicum miliaceum. Euphytica, 125, 337–348.
- Karyudi, R. J. F., & Fletcher, R. J. (2003). Osmoregulation in birdseed millet under conditions of water stress Ⅱ. variation in F3 lines of Setaria italica and its relationship to plant morphology and yield. Euphytica, 132, 191–197.
- Kato, T., & Takeda, K. (1996). Associations among characters related to yield sink capacity in space-planted rice. Crop Science, 36, 1135–1139.
- Kimata, M., Ashok, E. G., & Seetharam, A. (2000). Domestication, cultivation and utilization of two small millets, Brachiria ramosa and Setaria glauca (Poaceae), in South India. Economic Botany, 54, 217–227.
- Kole, C., Muthamilarasan, M., Henry, R., Edwards, D., Sharma, R., Abberton, M., & Prasad, M. (2015). Application of genomics-assisted breeding for generation of climate resilient crops: Progress and prospects. Frontiers in Plant Science, 6, 563.
- Lata, C., & Prasad, M. (2012). Foxtail millet, a model crop for genetic, genomic studies in bioenergy grasses. Critical Reviews in Biotechnology, 33, 328–343.
- Liu, T. Y., Ye, N., Song, T., Cao, Y., Gao, B., Zhang, D., & Zhang, J. (2019). Rhizosheath formation and involvement in foxtail millet (Setaria italica) root growth under drought stress. Journal of Integrative Plant Biology, 61, 449–462.
- Lyon, D. J., Burgener, P. A., DeBoer, K. L., Harveson, R. M., Hein, G. L., Hergert, G. W., & Vigil, M. F. (2008). Producing and marketing proso millet in the great plains. Publication # EC137. Lincoln, NE: University of Nebraska Cooperative Extension Service.
- Matsuura, A., Tsuji, W., An, P., Inanaga, S., & Murata, K. (2012). Effect of pre- and post-heading water deficit on growth and grain yield of four millets. Plant Production Science, 15, 323–331.
- Meng, L. S., & Yao, S. Q. (2015). Transcription co-activator Arabidopsis ANGUSTIFOLIA3 (AN3) regulates water-use efficiency and drought tolerance by modulating stomatal density and improving root architecture by the trans repression of YODA (YDA). Plant Biotechnology Journal, 13, 893–902.
- Nagore, M. L., Maggiora, A. D., Andrade, F. H., & Echarte, L. (2017). Water use efficiency for grain yield in an old and two more recent maize hybrids. Field Crops Research, 214, 185–193.
- Nematpour, A., Eshghizadeh, H. R., & Zahedi, M. (2019). Drought-tolerance mechanisms in foxtail millet (Setaria italica) and proso millet (Panicum miliaceum) under different nitrogen supply and sowing dates. Crop Pasture Science, 70, 442–452.
- Pantuwan, G., Fukai, S., Cooper, M., Rajatasereekul, S., & O’Toole, J. C. (2002). Yield response of rice (Oryza sativa L.) genotypes to drought under rainfed lowland. 3. Plant factors contributing to drought resistance. Field Crops Research, 73, 181–200.
- Parida, A., Das, A. B., & Dam, P. (2002). NaCI stress causes changes in photosynthetic pigments, proteins, and other metabolic components in the leaves of a true mangrove, Bruguiera parviflora, in hydroponic cultures. Journal of Plant Biology, 45, 28–36.
- Passioura, J. B. (2006). Increasing crop productivity when water is scarce - From breeding to field management. Agricultural Water Management, 80, 176–196.
- Rajput, S. G., Santra, D. K., & Schnable, J. (2016). Mapping QTLs for morphoagronomic traits in proso millet (Panicum miliaceum, L.). Molecular Breeding, 36, 1–18.
- Reynolds, M., & Tuberosa, R. (2008). Translational research impacting on crop productivity in drought-prone environments. Current Opinion in Plant Biology, 11, 171–179.
- Richards, R. A., Rebetzke, G. J., Condon, A. G., & van Herwaarden, A. F. (2002). Breeding opportunities for increasing the efficiency of water use and crop yield in temperate cereals. Crop Science, 42, 111–121.
- Ruggiero, A., Punzo, P., Landi, S., Costa, A., Oosten, M. J., & Grillo, S. (2017). Improving plant water use efficiency through molecular genetics. Horticulture, 3, 31.
- Schnyder, H. (1993). The role of carbohydrate strong and redistribution in the source–Sink relations of wheat and barley during grain filling—A review. New Phytologist, 123, 233–245.
- Sio-Se Mardeh, A., Ahmadi, A., Poustini, K., & Mohammadi, V. (2006). Evaluation of drought resistance indices under various environmental conditions. Field Crops Research, 98, 222–229.
- Tanaka, A., & Fujita, K. (1971). Studies on the nutrio-physiology of the corn plant (Part 7): Analysis of dry matter production from the source–Sink concept. Japanese Society of Soil Science and Plant Nutrition, 42, 152–156. (In Japanese.).
- Tang, S., Li, L., Wang, Y., Chen, Q., Zhang, W., Jia, G., & Diao, X. (2017). Genotype-specific physiological and transcriptomic responses to drought stress in Setaria italica (an emerging model for Panicoideae grasses). Scientific Reports, 7, 10009.
- Thapa, S., Reddy, S. K., Fuentealba, M. P., Xue, Q., Rudd, J. C., Jessup, K. E., & Liu, S. (2018). Physiological responses to water stress and yield of winter wheat cultivars differing in drought tolerance. Journal of Agronomy and Crop Science, 204, 347–358.
- Tsuchida, Y., Negoro, K., & Hishiike, M. (2011). Effect of initiation timing of drought stress on carbohydrate content and vegetative growth in Japanese apricot (Prunus mume Sieb. et Zucc.) ‘Nanko’. Journal of the Japanese Society for Horticultural Science, 80, 19–25.
- Vadez, V., Kholová, J., Yadav, R. S., & Hash, T. H. (2013). Small temporal differences in water uptake among varieties of pearl millet (Pennisetum glaucum (L.) R. Br.) are critical for grain yield under terminal drought. Plant and Soil, 371, 447–462.
- Vadez, V., Kholova, J., Medina, S., Kakkera, A., & Anderberg, H. (2014). Transpiration efficiency: New insights into an old story. Journal of Experimental Botany, 65, 6141–6153.
- Wang, P., Wang, H., Wang, Y., Ren, F., & Liu, W. (2018). Analysis of bHLH genes from foxtail millet (Setaria italica) and their potential relevance to drought stress. PLoS One, 13(11), e0207344.
- Wardlaw, I. F. (1990). The control of carbon partitioning in plants. New Phytologist, 116, 341–381.
- Wen, X. X., Zhang, D. Q., Liao, Y. C., Jia, Z. K., & Ji, S. Q. (2012). Effect of water-collecting and retaining techniques on photosynthetic rates, yield, and water use efficiency of millet grown in a semiarid region. Journal of Integrative Agriculture, 11, 1119–1128.
- Xu, B., Li, F., Shan, L., Ma, Y., Ichizen, N., & Huang, J. (2006). Gas exchange, biomass partition, and water relationships of three grass seedlings under water stress. Weed Biology Management, 6, 79–88.
- Xu, Y.-F., Ookawa, T., & Ishihara, K. (1997). Analysis of the dry matter production process and yield formation of the high-yielding rice cultivar Takanari, from 1991 to 1994. Japanese Journal of Crop Science, 66, 42–50. (In Japanese with English abstract.).
- Zegada-Lizarazu, W., & Iijima, M. (2005). Deep root water uptake ability and water use efficiency of pearl millet in comparison to other millet species. Plant Production Science, 8, 454–460.
- Zhang, P. P., Feng, B. L., Wang, P. K., Dai, H. P., Song, H., Gao, X. L., Gao, J., … Chai, Y. (2012). Leaf senescence and activities of antioxidant enzymes in different broomcorn millet (Panicum miliaceum L.) cultivars under simulated drought condition. Journal of Food, Agriculture & Environment, 10, 438–444.