Abstract
The preparation of a phosphorylated α-dicarbonyl compound designed to specifically react with arginine residues of enzymes accepting phosphorylated compounds as effectors is reported, and shown to inhibit rabbit muscle aldolase in a time-dependent and irreversible manner. This irreversible inhibition occured in a buffer devoid of borate ions, suggesting that the presence of the phosphate moiety contributes in the stabilization of the adduct formed with arginine residues. Under the same conditions, the metalloenzyme iron superoxide dismutase, in which an arginine is known to be critical for the catalytic function, is not significantly inhibited.
Introduction
Enzyme chemical modifications with specific monofunctional reagents is a method widely used for identifying amino acid residues, which play an important role for catalytic activity, and constitutes a powerful complementary approach to site-directed mutagenesis in elucidating structure-function relationships [Citation1]. Reagents such as phenylglyoxal [Citation2] (PG) and related compounds (p-hydroxyphenylglyoxal [Citation3], 4-hydroxy-3-nitrophenylglyoxal [Citation4]), 2,3-butanedione [Citation5] (BD) or 1,2-cyclohexanedione [Citation6] (CHD) () are known to be highly selective for modification of arginyl residues in proteins, although phenylglyoxal has been reported to react under some conditions with peptide and protein α-amino groups presumably by deamination reactions [Citation1]. Other non-specific reagents have also been used for the modification of arginine, such as glyoxal and ninhydrin that modify both arginyl and lysil residues [Citation7,Citation8], or hydrazine in aqueous conditions but that induces peptide bond cleavage [Citation9]. A number of reports show that the experimental conditions used, such as pH or buffer, have a significant effect on the stability of the complexes formed between arginyl residues and α-dicarbonyl compounds. For example, the reaction of 2,3-butanedione with arginyl residues has been shown to be affected by the presence of borate ions that would stabilize the adduct () [Citation10]. This complex is readily converted back to free arginine by the removal of borate by dialysis, resulting in the recovery of enzyme activity. In the presence of 1,2-cyclohexanedione in neutral conditions, arginine residues form N7,N8-(1,2-dihydroxycyclohex-1,2-ylene)-L-arginine compounds (DHCH-arginine), which were found to be stabilized by the presence of borate, but unstable in other buffers such as Tris, and converted back to free arginine by hydroxylamine [Citation11]. On the other hand, under more alkaline conditions, arginine residues react irreversibly with 1,2-cyclohexanedione to yield N5-(4-oxo-1,3-diazaspiro[4,4]non-2-yliodene)-L-ornithine compounds [Citation6]. Several of these arginine-specific reagents were used in the seventies to prove the importance of arginines for the catalytic activity of aldolases, a class of enzymes that catalyse the reversible aldol condensation of glyceraldehyde-3-phosphate and dihydroxyacetone phosphate to form fructose-1,6-bisphosphtae. For example, rabbit muscle aldolase has been shown to be modified by phenylglyoxal with a unique modifications of one arginine residue per subunit [Citation12], and by 1,2-cyclohexanedione that specifically modified Arg-55 with loss of enzyme activity [Citation13]. During the course of our studies on therapeutic agents for the treatment of parasitic diseases, we became interested in developing specific inhibitors of aldolases proven to be interesting therapeutic targets. Several compounds, including diketo derivatives, have already demonstrated inhibitory activity towards aldolases Citation13-17. In the present work, a phosphorylated α-dicarbonyl compound (phosphoric acid mono-(2,3-dioxo-butyl)ester, 6), has been synthesized and investigated for its specificity to irreversibly inhibit rabbit muscle aldolase. This compound was designed, (1) to modify arginine residues as α-diketo derivative and, (2) to target this glycolytic enzyme known to accept primarily phosphorylated compounds as effectors (substrate or inhibitors).
Figure 1 Commonly used arginine-specific reagents: phenylglyoxal (PG), 2,3-butanedione (BD) and 1,2-cyclohexanedione (CD).
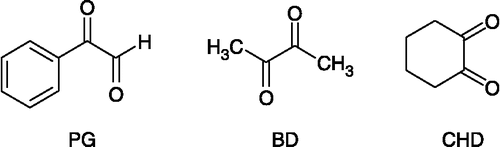
Figure 2 Postulated reaction of arginine residue with 2,3-butanedione in the presence of borate ions [Citation9] (A) and with compound 6 (B).
![Figure 2 Postulated reaction of arginine residue with 2,3-butanedione in the presence of borate ions [Citation9] (A) and with compound 6 (B).](/cms/asset/1f80042b-a9d6-493a-b676-89c98c21c891/ienz_a_238260_f0002_b.gif)
Materials and methods
General
Escherichia coli iron superoxide dismutase (EC 1.15.1.1, approx. 2500 units/mg) and rabbit muscle aldolase (EC 4.1.2.13, approx. 10 units/mg) were purchased from Sigma, and all other chemicals were obtained from Aldrich or Sigma, and used without further purification. NMR spectra were obtained with a Bruker AC 250 at 250 MHz (1 H), 60 MHz (13C) or 80 MHz (31P). Kinetics were recorded on an UV-mc2 spectrophotometer (SAFAS, Monaco).
Synthesis of phosphorylated α-diketo compound
2,2-Dimethoxy propionic acid methyl ester (2)
To a solution of pyruvic acid (5 g, 57 mmol) in methanol (200 mL) was added trimethyl orthoformate (60 mL) and concentrated sulfuric acid (1.5 mL), and the mixture was magnetically stirred for 5 days at room temperature. Resin Dowex 1X8 (HCO3− form) was then added, and the solution was filtered. The solvent was then evaporated to dryness, and the crude product was distilled under reduced pressure to afford a colorless oil (5.2 g, 62%). Bp: 70°C (2 mm Hg). 1H-NMR (CDCl3): δ 1.41 (s, 3H, CH3C), 3.17 (s, 6H, (CH3O)2), 3.70 (s, 3H, CH3OCO).
Sodium 2,2-dimethoxy propionate (3)
To a solution of 2 (2 g, 13.5 mmol) in methanol (20 mL) was added a 2 M aqueous solution of sodium hydroxide, and the mixture was stirred for 30 min at room temperature. The pH was then adjusted to pH 8.0 by addition of a 2 M aqueous solution of hydrochloric acid, and the solution was evaporated to dryness. Ethanol (50 mL) was added, and the solution was filtered. The filtrate was then evaporated to dryness to yield a white solid (1.81 g) that was dried under reduced pressure in the presence of phosphorus pentoxide (yield: 86%). 1H-NMR (D2O): δ1.30 (s, 3H, CH3C), 3.09 (s, 6H, CH3O)2C).
1-Diazo-3,3-dimethoxy-2-butanone (4)
To a solution of 3 (1.6 g, 10 mmol) in ethyl ether (50 mL) were added, at –10°C, pyridine (0.1 mL) and oxalyl chloride (1 mL) and the mixture was allow to stir without external cooling for 1 h. This solution was then added dropwise to a diazomethane solution [CARE-TOXIC] in ethyl ether at − 70°C (freshly prepared from 47 mmol of Diazald®). The resulting solution was stirred until room temperature was reached, and the excess of diazomethane was removed under argon flux and trapped in a mixture of ether and acetic acid (1:1, v:v). The solvent was removed, and the residue was then diluted into ethyl ether (10 mL), filtered, and concentrated under reduced pressure. The product was purified by column chromatography using a mixture of dichloromethane and methanol (99:1, v:v) as eluent to yield 1.1 g of pure 4 as a yellow oil (yield: 69%). IR (NaCl): ν (cm− 1) 1616 (C = O), 2112 (C = N2). 1H-NMR (CDCl3): δ 1.42 (s, 3H, CH3C), 3.25 (s, 6H, (CH3O)2), 5.83 (s, 1H, CHN2).
Phosphoric acid dibenzyl ester 3,3-dimethoxy-2-oxo-butyl ester (5)
To a solution of 4 (0.9 g, 5.7 mmol) in toluene (5 mL) was added a solution of dibenzyl phosphate (2 g, 7.2 mmol) in toluene (20 mL). After 60 h under magnetic stirring at 50°C, the mixture was diluted into ethyl ether (100 mL). The solution was washed with a diluted bicarbonate aqueous solution (50 mL), then with water (50 mL). The organic layer was then dried over magnesium sulfate, and the solvent was removed under reduced pressure. The product was purified by column chromatography using a mixture of ethyl ether and hexane (80:20, v:v) as eluent to yield a colorless oil (300 mg, yield: 13%). 1H-NMR (CDCl3): δ (ppm) 1.34 (s, 3H, CH3C), 3.19 (s, 6H, (CH3O)2), 4.88 (d, 2H, 3JHP = 11 Hz, CH2OP), 5.12 (d, 4H, 2JHP = 7.9 Hz, CH2-C6H5). 13C-NMR (CDCl3): δ (ppm) 20.1 (CH3C), 49.6 (CH3O), 69.6 (d, 2JCP = 5.4 Hz, CH2-O-P), 69.7 (d, 2JCP = 5.6 Hz, P(O)CH2–C6H5), 102.5 (C(OCH3)2), 128.0, 128.6, 135.8 (Carom), 202.2 (C = O). 31P-NMR (CDCl3): δ (ppm) – 0.66.
Phosphoric acid mono-(2,3-dioxo-butyl) ester (6)
Palladium on charcoal (10% w/w, 0.1 g) was added to a stirred solution of 5 (0.15 g, 0.37 mmol) in methanol (10 mL). The final mixture was transferred to a pressure reactor and kept under molecular hydrogen (1 atm.) at room temperature for 30 min. The catalyst was then filtered off, and a solution of cyclohexylamine (74 mg, 0.75 mmol) in methanol (5 mL) was added to the reaction mixture and the solvent was evaporated to dryness. The resulting white solid was then washed with ethyl ether (20 mL) and dried for 16 h under reduced pressure over P2O5 to afford 125 mg of compound 6 as the cyclohexylammonium salt (62 − .2X+, yield: 79%). IR (KBr): ν (cm− 1) 3600–3000 (N-H), 1749 (C = O). 1H-NMR (D2O): δ (ppm) 1.38 (s, 3H, CH3C), 2.10 (m, 20H, CH2 cyclohexyl), 3.06 (m, 2H, CHN), 3.18 (s, 6H, (CH3)2), 4.70 (d, 2H, 3JHP = 10.7 Hz, CH2OP). 13C-NMR (D2O): δ (ppm) 22.2 (CH3C), 26.4, 26.9 and 32.9 (CH2 cyclohexyl), 52.2 (OCH3), 52.8 (CHN), 69.6 (CH2OP), 105.1 (C(OCH3)2), 210.7 (d, 3JCP = 7.2 Hz, C = O). 31P-NMR (D2O): δ (ppm) 4.32. Anal. Calcd. for C18H39N2O7P, 2H2O C, 46.74; H 9.37; N, 6.06. Found: C, 46.69; H, 8.72; N, 6.03%. To a solution of 62 − .2X+. (50 mg, 0.12 mmol) in water (0.5 mL) was added 1 mL Dowex 50WX8 resin (H+ form). After 5 min at room temperature, the resin was filtered off and washed with water (0.5 mL), and the solution was kept at 40°C for 5 h. Compound 6 was thus obtained in solution in water (conc. ≈ 0.12 moL.L− 1). 1H-NMR (H2O + D2O): δ (ppm) 2.30 (s, 3H, CH3CO), 3.96 (d, 2H, 3JHP = 5.3 Hz, CH2OP). 13C-NMR (H2O + D2O): δ (ppm) 26.9 (CH3C), 70.35 (d, 2JCP = 5.0 Hz, CH2OP), 97.5 (d, 3JCP = 10.5 Hz, C(2)(OH)2), 211.8 (C(3) = O), 210.7. (Note: 13C-NMR spectrum indicates that the 2-carbonyl is hydrated to form the geminal dihydroxy compound, as revealed by 31P coupling to the hydrated carbon at δ 97.5 ppm).
Aldolase. Assay methods
Aldolase activity (10 U/mg at 25°C) was measured by following NADH oxidation at 340 nm at 25°C using a coupled assay system based on triose-phosphate isomerase (TIM) and glycerol-3-phosphate dehydrogenase (GDH). Assays were initiated by the addition of substrate fructose-1,6-bisphosphate (FBP, 1 mM final concentration) to a final volume of 1 mL of solution containing aldolase in triethanolamine (TEA) buffer (100 mM TEA/HCl, pH 7.6, 50 mM NaCl and 1 mM NaN3), 0.42 mM NADH and coupling enzymes (10 μg/mL GDH, 1 μg/mL TIM), as already described [Citation18] Aldolase was dialyzed overnight at 4°C against TEA buffer before use and the protein concentration was estimated using A1% (280 nm) = 9.1.
Inhibition study
Aldolase (0.2 mg/mL in 0.2 mL TEA buffer) was incubated in the presence of various concentrations of compound 6 (1.2–4.2 mM). Periodically, 20 μL aliquots were removed and assayed for aldolase activity. Routinely, control experiments were run in the absence of inhibitor and all measurements were conducted in triplicate and the mean values were calculated.
Irreversible inhibition study of aldolase. Data analysis
Time-dependant inactivation plots such as those presented in resulted from experiments run in triplicate. For such inactivation, two pathways can be generally envisaged: (1) formation of a reversible enzyme-inhibitor complex (EI) between the enzyme (E) and the inhibitor (I), followed by irreversible inactivation of the enzyme through formation of a covalent complex (EI*) (Equation I) or (2) simultaneous complex formation and inactivation (Equation II). The two pathways of inactivation can be presented as follows:
Figure 3 Kinetics of inactivation of aldolase by compound 6. Reactions were initiated by the addition of varying concentrations of 6 to solutions containing aldolase in 0.1 M TEA buffer, pH 7.6, at 25°C. At the indicated times, 20 μL aliquots were removed and assayed for aldolase activity, as indicated in the experimental section. (A) Neperian logarithm of residual activity (RA) of rabbit muscle aldolase (2 μg/mL) at different incubation times of inhibitor 6. ([6] = 1.2 mM (▪), 2.1 mM (•), 3 mM (▴), 4.2 mM (♦)). (B) Half life of inactivation of aldolase plotted in Kitz-Wilson format vs the reciprocal of the concentration in inhibitor 6. An apparent Ki value of 3.6 mM and an apparent kinact value of 0.03 min− 1 were determined.
![Figure 3 Kinetics of inactivation of aldolase by compound 6. Reactions were initiated by the addition of varying concentrations of 6 to solutions containing aldolase in 0.1 M TEA buffer, pH 7.6, at 25°C. At the indicated times, 20 μL aliquots were removed and assayed for aldolase activity, as indicated in the experimental section. (A) Neperian logarithm of residual activity (RA) of rabbit muscle aldolase (2 μg/mL) at different incubation times of inhibitor 6. ([6] = 1.2 mM (▪), 2.1 mM (•), 3 mM (▴), 4.2 mM (♦)). (B) Half life of inactivation of aldolase plotted in Kitz-Wilson format vs the reciprocal of the concentration in inhibitor 6. An apparent Ki value of 3.6 mM and an apparent kinact value of 0.03 min− 1 were determined.](/cms/asset/87603921-b4be-4af0-a48d-fc93e5211c92/ienz_a_238260_f0003_b.gif)
The values of the inactivation rate constant (kinact) and of the dissociation constant of complex EI (Ki) defined as (k− 1+k+1) / k+1, were obtained from the kinetic Equation (Equation III) corresponding to the first pathway (Equation I) under steady-state conditions, by plotting the half-life of inactivation (t1/2) against the inverse of inhibitor concentration.
Extrapolating from this plot, ln (2) / kinact was obtained from the intercept on the ordinate and − 1 / Ki on the abscissa.
For the second pathway (Equation II) the kinetic equation can be simplified to Equation (IV). Plotting the half-life of inactivation against the inverse of inhibitor concentration results in a straight line that would pass through the origin. However, this model did not provide good fits with our experimental data.
Irreversible inhibition study of superoxide dismutase
Inactivation assays of superoxide dismutase was carried out with E. coli Fe-SOD in the presence of 6 (5 – 50 mM) at 25°C in 50 mM sodium pyrophosphate, 50 mM NaHCO3, containing 0.1 mM EDTA (pH 9.0). At different times, aliquots were diluted and enzymatic activity was quantified using the SOD-assay based on the superoxide-mediated autoxidation of pyrogallol [Citation19] (conditions: 25°C in a 50 mM Tris buffer pH 8.2 containing 1 mM EDTA, 0.2 mM pyrogallol and enzyme (1.5 units), in a final volume of 500 μL with 1% DMSO).
Results and Discussion
Synthesis of Phosphoric acid mono-(2,3-dioxo-butyl) ester (6)
Owing to the importance of dihydroxyacetone and its phosphate analog dihydroxyacetone phosphate (DHAP) in nature as an important C3 building block, a number of DHAP analogs has been developed Citation20-21 and used for different purposes, for example as enzyme inhibitors. A general procedure for the preparation of such compounds involves reaction of phosphoric diesters with the appropriate α-diazoketone derivatives [Citation22], which are generally easily obtained from acyl chlorides and diazomethane. Here, we used this strategy to prepare the phosphorylated α-diketo compound 6 (phosphoric acid mono-(2,3-dioxo-butyl)ester), which was obtained in five steps from pyruvate as starting material (Scheme ). In the first step, treatment of pyruvic acid (1) by trimethyl orthoformate in the presence of methanol and sulfuric acid gave, after five days at room temperature, the corresponding dimethyl acetal ester 2. After saponification, the resulting dimethoxypropanoate intermediate 3 was transformed by oxalyl chloride into its acyl chloride derivative, which was then converted to the corresponding diazoketone 4 by treatment with in situ generated diazomethane. Reaction of compound 4 with dibenzyl phosphate gave in moderate yields the corresponding phosphorylated compound 5. Removing of benzyl groups under catalytic hydrogenation conditions, followed by treatment on cation-exchange resin gave finally the desired compound 6.
Time-dependent irreversible inhibition of aldolase by compound 6
The catalytic activity of rabbit muscle aldolase has been reported to be affected by 1,2-cyclohexanedione with a specific modification of the solvent-exposed residue arginine-55 [Citation13]. The role of this residue, which is not located at the active site of the enzyme, has been proposed in participating, with lysine-146, in the phosphate-binding site for the C-1 phosphate of 1,6-bisphosphate [Citation13]. But in view of the available structural data on aldolases, arginine-55 is too far from the active site for a possible interaction with the substrate [Citation23]. However this residue is supposed to play a critical role as its modification leads to the inactivation of the enzyme. Thus, one possibility is that chemical modification of this residue, located near the entrance to the active site could lead to a steric repulsion of the substrate. Plots of percent residual activity versus time for different concentrations of compound 6 show a time-dependant inactivation of rabbit muscle aldolase in TEA buffer (pH 7.6). As shown in , data of inactivation fitted a pseudo-first-order kinetic process. The activity of the partially modified enzyme remained constant with time when an aliquot of the enzyme-inhibitor complex solution (10 μL) was diluted into TEA buffer (1 mL final volume), consistent with an irreversible inhibition process. In accordance with a two-step model for irreversible inhibition (i.e. formation of a reversible complex EI, followed by the slow formation of an irreversible EI* complex, Equation (I), see material and method, the dissociation constant of the reversible enzyme-inhibitor complex Ki = 3.6 mM and the rate constant for irreversible inhibition kinact = 0.03 min− 1 were determined from a Kitz-Wilson plot, in which the half lives of inactivation were plotted against the reciprocal of the inhibitor concentration ().
Aldolase was reported to be irreversibly inhibited by 2,3-butanedione, but only in the presence of borate ions that were proposed to form a stabilized cyclic borate ester (). However, the inactivation of the enzyme by butanedione was shown to be reversed by removing excess of borate ion, leading to a rapid restoration of enzymatic activity. Here, an irreversible inhibition of aldolase was observed in a buffer devoid of borate ions suggesting that the presence of a phosphate moiety in compound 6 contributes to stabilize intramolecularly the adduct. One possibility is that the phosphate group, which exists mainly as the mono-ionized form under the experimental conditions (pH 7.6), could catalyze dehydration of the carbinolamine intermediate resulting in imine formation, and subsequent irreversible enzyme inactivation, as proposed in . To further study the inhibitory properties of dicarbonyl compound 6 on arginine-dependant enzymes, we then examined inhibition of superoxide dismutases (SODs). This class of enzymes constitute the primary enzymatic defense against oxygen metabolites by catalyzing the dismutation of superoxide anion radical O2. − into hydrogen peroxide and oxygen. Chemical modification of Fe and Mn-SODs with arginyl-specific reagents has been used to show that residue Arg-189, involved in the electrostatic control of substrate diffusion and highly conserved in a large majority SODs, is critical for the catalytic function [Citation24]. Astonishingly, we found that the activity of E. Coli Fe-SOD in the presence of compound 6, even at high concentrations (5 mM) was not significantly affected after 3 hours (data not shown). From this result, it appears that the phosphate group in compound 6 play a determinant role in the discrimination between the targeted enzymes and provide specificity towards enzymes that accept phosphorylated effectors.
Conclusion
α-Dicarbonyl compounds including phenylglyoxal, butanedione and cyclohexanedione are the most commonly employed arginyl reagents, and their use have led in the past to the identification of a large number of enzymes and other proteins that contain arginine residues critical to biological function. Here, we prepared a phosphorylated α-dicarbonyl compound that inhibits aldolase in a time-dependent and irreversible manner, while Fe-SOD is not affected. A mechanism based on the intramolecular phosphate-catalyzed dehydration of the carbinolamine of the adduct between inhibitor and arginine residue is proposed. Further studies are now underway to examine whether other glycolytic arginine-dependant enzymes known to accept phosphorylated compounds are inhibited by this compound.
References
- Lundblad RL. The modification of arginine. Chemical reagents for protein modification2nd ed. CRC Press. 1991; 173–213
- Takahashi K. The reaction of phenylglyoxal with arginine residues in proteins. J Biol Chem 1968; 243: 6171–6179
- Yamasaki RB, Vega A, Feeney RE. Modification of available arginine residues in proteins by p-Hydroxyphenylglyoxal. Anal Biochem 1980; 109: 32–40
- Borders CL, Jr, Pearson LJ, McLaughlin AE, Gustafson ME, Vasiloff J, An FY, Morgan DJ. 4-Hydroxy-3-nitrophenylglyoxal. A chromophoric reagent for arginyl residues in proteins. Biochim Biophys Acta 1979; 568: 491–495
- Yankeelov JA, Jr, Mitchell CD, Crawford TH. A simple trimerization of 2,3-butanedione yielding a selective reagent for the modification of arginine in proteins. J Am Chem Soc 1968; 90: 1664–1666
- Toi K, Bynum E, Norris E, Itano HA. Studies on the chemical modification of arginine. I. The reaction of 1,2-cyclohexanedione with arginine and arginyl residues of proteins. J Biol Chem 1967; 242: 1036–1043
- Takahashi K. Further studies on the reactions of phenylglyoxal and related reagents with proteins. J Biochem (Tokyo) 1977; 81: 403–414
- Chaplin MF. The use of ninhydrin as a reagent for the reversible modification of arginine residues in proteins. Biochem J 1976; 155: 457–459
- Honegger A, Hughes GJ, Wilson KJ. Chemical modification of peptides by hydrazine. Biochem J 1981; 199: 53–59
- Riordan JF. Functional arginyl residues in carboxypeptidase A. Modification with butanedione. Biochemistry 1973; 12: 3915–3923
- Patthy L, Smith EL. Reversible modification of arginine residues. Application to sequence studies by restriction of tryptic hydrolysis to lysine residues. J Biol Chem 1975; 250: 557–564
- Lobb RR, Stokes AM, Hill HA, Riordan JF. A functional arginine residue in rabbit-muscle aldolase. Eur J Biochem 1976; 70: 517–522
- Patthy L, Varadi A, Thesz J, Kovacs K. Identification of the C-1-phosphate-binding arginine residue of rabbit-muscle aldolase. Isolation of 1,2-cyclohexanedione-labeled peptide by chemisorption chromatography. Eur J Biochem 1979; 99: 309–313
- Vlahos CJ, Ghalambor MA, Dekker EE. Evidence for an essential arginine residue in the active site of Escherichia coli 2-keto-4-hydroxyglutarate aldolase. Modification with 1,2-cyclohexanedione. J Biol Chem 1985; 260: 5480–5485
- Ogata H, Takeo K, Kuwahara A, Suzuno R, Fujimoto M, Shimizu J. An exploration of the binding site of aldolase using alkanediol monoglycolate bisphosphoric esters. Biochim Biophys Acta 1983; 742: 384–390
- Patthy L, Thesz J. Origin of the selectivity of alpha-dicarbonyl reagents for arginyl residues of anion-binding sites. Eur J Biochem 1980; 105: 387–393
- Lakhdar-Ghazal F, Blonski C, Willson M, Michels P, Perie J. Glycolysis and proteases as targets for the design of new anti-trypanosome drugs. Curr Top Med Chem 2002; 2: 439–456
- Racker E. Spectrophotometric measurement of hexokinase and phosphohexokinase activity. J Biol Chem 1947; 167: 843–854
- Marklund S, Marklund G. Involvement of the superoxide anion radical in the autoxidation of pyrogallol and a convenient assay for superoxide dismutase. Eur J Biochem 1974; 47: 469–474
- Gefflaut T, Blonski C, Perie J. Slow reversible inhibitions of rabbit muscle aldolase with substrate analogues: Synthesis, enzymatic kinetics and UV difference spectroscopy studies. Bioorg Med Chem 1996; 4: 2043–2054
- Blonski C, Gefflaut T, Perie J. Effects of chirality and substituents at carbon 3 in dihydroxyacetone-phosphate analogues on their binding to rabbit muscle aldolase. Bioorg Med Chem 1995; 3: 1247–1253
- Hajra AK, Agranoff BW. Acyl dihydroxyacetone phosphate. Characterization of a 32P-labeled lipid from guinea pig liver mitochondria. J Biol Chem 1968; 243: 1617–1622
- Blom N, Sygusch J. Product binding and role of the C-terminal region in class I D-fructose 1,6-bisphosphate aldolase. Nat Struct Biol 1997; 4: 36–39
- Polticelli F, Bottaro G, Battistoni A, Carri MT, Djinovic-Carugo K, Bolognesi M, O'Neill P, Rotilio G, Desideri A. Modulation of the catalytic rate of Cu,Zn superoxide dismutase in single and double mutants of conserved positively and negatively charged residues. Biochemistry 1995; 34: 6043–6049