Abstract
Catecholase and cresolase activities of mushroom tyrosinase (MT) were studied in presence of some n-alkyl carboxylic acid derivatives. Catecholase activity of MT achieved its optimal activity in presence of 1.0, 1.25, 2.0, 2.2 and 3.2 mM of pyruvic acid, acrylic acid, propanoic acid, 2-oxo-butanoic acid, and 2-oxo-octanoic acid, respectively. Contrarily, the cresolase activity of MT was inhibited by all type of the above acids. Propanoic acid caused an uncompetitive mode of inhibition (Ki=0.14 mM), however, the pyruvic, acrylic, 2-oxo-butanoic and 2-oxo-octanoic acids showed a competitive manner of inhibition with the inhibition constants (Ki) of 0.36, 0.6, 3.6 and 4.5 mM, respectively. So, it seems that, there is a physical difference in the docking of mono- and o-diphenols to the tyrosinase active site. This difference could be an essential determinant for the course of the catalytic cycle. Monophenols are proposed to bind only the oxyform of the tyrosinase. It is likely that the binding of acids occurs through their carboxylate group with one copper ion of the binuclear site. Thus, they could completely block the cresolase reaction, by preventing monophenol binding to the enzyme. From an allosteric point of view, n-alkyl acids may be involved in activation of MT catecholase reactions.
Abbreviations: | ||
= | Mushroom Tyrosinase (MT) | |
= | Inhibition constants (Ki) | |
= | Agaricus bisporus (A. bisporus) | |
= | 4-[(4-methylphenyl) azo]-phenol (MePAPh) | |
= | 4-[(4-methylbenzo) azo]-1,2-benzenediol (MeBACat) | |
= | Phosphate buffer solution (PBS) | |
= | 3,4-Dihydroxyphenylalanine (DOPA) |
Introduction
Tyrosinase (EC 1.14.18.1) catalyzes the oxidation of mono-, di-, and polyhydric phenols to o-quinones [Citation1]. Quinones can polymerize non-enzymatically to melanin as the most important natural biopolymer responsible of pigmentation and the color and patterns of mammalian skin [Citation2, Citation3].
The production of abnormal melanin pigmentation (melasma, freckles, ephelide, senile lentigines, etc.) is a serious esthetic problem in human beings [Citation4]. In addition, tyrosinase is responsible for the undesired enzymatic browning of fruits and vegetables [Citation5] that take place during senescence or damage at the time of post harvest handling, which makes the identification of novel tyrosinase inhibitors extremely important.
Various aromatic carboxylic acids are known potent inhibitors of tyrosinase [Citation6–8]. Benzoic, isovanillic, cinnamic, p-coumaric, salicylic and 3-hydroxybenzoic acids inhibit the oxidation of catechol and the hydroxylation of tyramine catalyzed by cherimoya epicarp polyphenol oxidase [Citation9]. Caffeic and ferulic acids activate the oxidation of catechol; protocatechuic and syringic acids activate the hydroxylation of tyramine [Citation9]. Aromatic carboxylic acid inhibition on o-diphenol oxidase from sweet cherry fruits was reduced by esterification or by replacing the benzene ring with an aliphatic or heterocyclic group, but not by replacing it with a highly unsaturated open chain [Citation10]. Study of phenylalkyl acids and alcohols, polyphenols, nitrophenols, esters, aldehydes, and ketones on catecholase activity showed carboxylic acids are stronger inhibitors than corresponding alcohols and aldehydes [Citation7].
Aliphatic carboxylic acids do not affect cherimoya (A. cherimolia Mill) epicarp polyphenol oxidase activity [Citation9]. Propionic, butyric and valeric acids and their salts in combination with or without unsaturated fatty acids strongly suppress the formation of tyrosinase, which catalyzes the formation of melanin and thus is a cause of dermatologic troubles. Experiments show that these acids suppress tyrosinase formation but do not inhibit tyrosinase [Citation11]. C18-22 fatty acids with more than two unsaturated bonds their salts, or their esters with mono- or di-hydric alcohols, show moisture-retaining, tyrosinase-inhibiting, hair growth stimulating effects and stabilized the cosmetics [Citation12]. In the other study, aliphatic dicarboxylic acids ranging between C8 and C13 have been introduced as inhibitors of polyphenoloxidase [Citation13]. Survey of literatures show that aromatic and aliphatic acids evokes different inhibition, activation and unchanged effects on the activity of tyrosinase from different species.
Mushroom tyrosinase (A. bisporus) is popular among researchers as it is commercially available and inexpensive and also there are easy tools to investigate the feature of this enzyme. In pursuit of our works about the structure, function and relationship of MT [Citation14–18] to understand the mechanism of enzyme activation and inhibition. Two new bi-pyridine synthetic compounds and three synthetic n-alkyl dithiocarbamates, with different tails, were recently introduced as potent uncompetitive MT inhibitors [Citation19–Citation20]. Thiophenol (0.09-0.4μM) inhibits both activities of MT and brings about no activation even at very low concentrations. But when the pH of the reaction medium was dropped to 5.3, thiophenol (<0.05μM) also caused activation of the cresolase activity of MT in the presence of p-coumaric acid [Citation21]. This work was devoted to an investigation of some saturated and unsaturated aliphatic carboxylic acids on the cresolase and catecholase reactions of mushroom tyrosinase.
Materials and methods
Materials
Mushroom tyrosinase (MT; EC 1.14.18.1), specific activity 3400 units/mg, was purchased from Sigma. 4-[(4-methylphenyl) azo]-phenol (MePAPh) and 4-[(4-methylbenzo) azo]-1,2-benzenediol (MeBACat), were synthesized as previously described [Citation22]. Pyruvic, acrylic and propanoic acids were obtained from Merck Co. 2-Oxo-butanoic and 2-oxo-octanoic acids were purchased from Sigma. The chemical formula of all acids used in this research is given in . The buffer used throughout in this research was 10 mM phosphate buffer solution (PBS), pH = 6.8, and its salts were obtained from Merck. All experiments were carried out at 293 °K and solutions were prepared in doubly distilled water.
Methods
Kinetic assays of catecholase and cresolase activities
The kinetic assays of catecholase and cresolase activities were carried out using Cary spectrophotometer, 100 Bio model, with jacketed cell holders. Freshly prepared enzyme and substrate solutions were used in this work. All enzymatic reactions were run in phosphate buffer (10 mM) at pH=6.8 in a conventional quartz cell thermostated to maintain the temperature at 20 ± 0.1 °C. The selected conditions of solvent, buffer, pH, temperature, and enzyme concentration were applied for assaying the oxidase activity of MT according to our previous studies [Citation20,Citation23]. Catecholase activity was followed by depletion of MeBACat for 2 min at its λmax = 364 nm and enzyme concentration of 11.8 mM, 40 unit/mL. The reactions were carried out using fixed concentration of substrate (50 μM) in different concentrations of the acids. Cresolase reactions were measured by depletion of MePAPh for 10 min at its λmax = 352 nm and enzyme concentration 70.8 μM, 240 unit/mL. The reactions were followed using six different fixed concentrations of substrate (5–50 μM) in different concentrations of the acids as inhibitors (0, 0.1, 0.2, 0.4 and 0.75 mM for pyruvic acid; 0, 0.2, 0.4, 0.8 and 1.2 mM for acrylic acid; 0, 0.5, 1, 1.25 and 1.5 mM for propanoic acid; 0, 0.4, 1, 1.5 and 2.5 mM for 2-oxo-butanoic acid and 0, 0.5, 1, 1.5 and 2 mM for 2-oxo-octanoic acid). All assays were repeated at least three times. Substrate addition followed after incubation of the enzyme with different concentrations of inhibitors. Definitions of units were defined by the vender. Accordingly, one unit cresolase activity is equal to the 0.001 change in the optical density of L-tyrosine per min at 280 nm in 3 mL of the reaction mixture at 25 °C and pH = 6.5. Similarly, one unit of catecholase activity is equal to the 0.001 change in the optical density of ascorbic acid per min at 265 nm in 3 mL of the reaction mixture (25 °C and pH = 6.5), when catechol or L-DOPA is used as substrate.
Results and discussion
In this study incubation of the enzyme with pyruvic, acrylic, 2-oxo-butanoic, 2-oxo-octanoic and propanoic acids in catecholase and cresolase reactions resulted to MT activation and inhibition, respectively.
The activatory effect of all five acids (shown in ) in catecholase reactions has been illustrated in . The relative activity of enzyme due to the oxidation of MeBACat increase in a concentration dependent manner to an optimum activity and after that the activity decrease gradually. Magnitudes of optimum activity and acid concentrations to reach these points were summarized in . Few studies have been devoted to investigation of the activation of tyrosinase from structural and kinetic point of view. In the case of frog epidermis pro-tyrosinase the activation should be the result of the cleavage of the polypeptide chain that determines change in tertiary or quaternary structure [Citation24]. A latent isoform of Agaricus bisporus tyrosinase has been isolated and activated by benzyl alcohol. The presence of a lag period and the lack of change of the molecular mass of the protein after activation could indicate a slow conformational change of the protein to render the final active form [Citation25]. The activation of latent tyrosinase occurred by different treatments or agents such as SDS [Citation26], fatty acids [Citation27], alcohols [Citation28] and proteases [Citation29]. Aspergillus oryzae protyrosinase is inactive at neutral and alkaline pH, and is activated by acid treatment [Citation30]. In other view to reveal such activation, we should refer to different binding sites for these acids and allosteric effects of the enzyme. Duckworth and Coleman studies with MT showed that the hydroxylation capacity of the enzyme shifts to one activated form in the presence of diphenol as soon as micromolar concentrations of the diphenol product appear [Citation6]. These allosteric models supported by Hearing and Ekel, who found that DOPA at low concentration acts as a non-competitive activator of tyrosine binding with induction of conformational change in normal tyrosinase [Citation31]. The word “allosteric” was proposed by Monod et al. in 1963, to explain the effect of non-substrate-like molecules on enzyme activity, in contrast to the effect of substrates or substrate-like molecules which cause cooperativity between the subunits of an enzyme [Citation32]. Although conformational flexibility of enzymes is considered to be the main reason for cooperation and allostery phenomena and there are also many allosteric enzymes which are cooperative or vice versa, it is still preferred that these terms are distinguished from each other [Citation33]. Nevertheless, this is a subtle topic which deserves some attention. For an enzyme like MT which can host substrates of various sizes, it seems that the interaction of the substrate residue with the pocket of the active site seriously influences the impact of the docking head of the substrate which coordinates to the copper ion in the active site. As a matter of fact, the docking style of different phenols of various sizes is not expected to be too different in the MT active site. But the way the residues of these substrates lay in the pocket of the active site would be predictably different. Thus, the overall conformational changes of MT results from the sum of the interactions of both head and body of the substrate with the active site and the pocket of the active site.
Figure 2. MT activation in the catecholase reactions with MeBACat as substrate in 10 mM PBS, 20 °C and pH 6.8, in the presence of pyruvic acid (○), acrylic acid (♦), propanoic acid (▵), 2-oxo-butanoic acid (□) and 2-oxo-octanoic acid (◊).
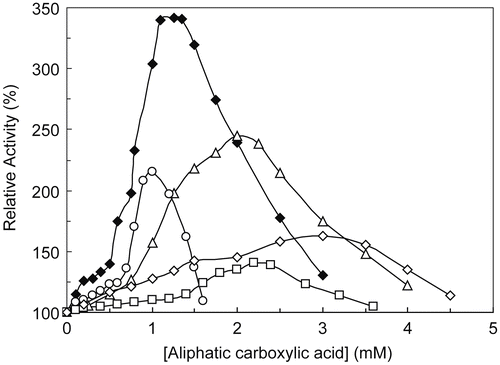
Table 1. Activation and inhibition parameters in catecholase and cresolase reactions
In a similar way, this discussion can be extended to the inhibitors of MT. Observing different responses from similar inhibitors with various sizes is not an unreasonable expectation [Citation20,Citation34]. So, if a critical condition for the “allostery” is to consider a second molecule alongside with the substrate as an effector. Therefore, from an allosteric point of view, these five aliphatic carboxylic acids may confer with the MT and activate its catecholase reaction.
depicts the double reciprocal Lineweaver-Burk plots for the cresolase activity of MT in different concentrations of propanoic acid. The plot show parallel straight lines indicate decreasing of the apparent values of Vmax with no effect on Km/Vmax values, which confirms the uncompetitive inhibition of the enzyme. The inhibition constant was calculated from values of reciprocal apparent maximum velocity (1/V′max) versus the concentration of inhibitor in the relevant secondary plot depicted in , which equals to 0.14 mM (). Double reciprocal Lineweaver-Burk plots for the cresolase activity of MT in the presence of pyruvic, acrylic, 2-oxo-butanoic and 2-oxo-octanoic acids, (), reveal inhibition with either of the acids (a, b, c and d, respectively). A series of straight lines intersecting exactly on the vertical axis, the value of maximum velocity (Vmax) is unchanged by the inhibitor but the K´m value is increased, which, confirms competitive inhibition of MT. The slope values at each concentration of inhibitor were obtained from and plotted versus concentration of inhibitor to give a secondary plot in (a, b, c and d, respectively), from which the inhibition constants (Ki) were obtained from the abscissa-intercept. Results for the Ki values have been summarized in . It is proposed that the catalytic and inhibitory sites of the enzyme seem to be close together [Citation10]. The uncompetitive type of inhibition for propanoic acid indicates that it should be bind at a site distinct from the active site and bind just with the enzyme-substrate (ES) complex and not with the free enzyme (E). Presumably due to binding of the substrate to the enzyme, a conformational change induces in the enzyme and this establishes a proper site for binding of the inhibitor.
Figure 3. (a) Double reciprocal Lineweaver-Burk plots of MT kinetic assays for cresolase reactions with MePAPh in 10 mM PBS, 20 °C and pH 6.8, in the presence of different fixed concentrations of propanoic acid: 0 (♦), 0.5 (□), 1.0 (▴), 1.25 (×) and 1.5 mM (*). (b) The secondary plot of reciprocal apparent maximum velocity (1/ V′max) versus the concentration of inhibitor [I], where the abscissa-intercept is −Ki.
![Figure 3. (a) Double reciprocal Lineweaver-Burk plots of MT kinetic assays for cresolase reactions with MePAPh in 10 mM PBS, 20 °C and pH 6.8, in the presence of different fixed concentrations of propanoic acid: 0 (♦), 0.5 (□), 1.0 (▴), 1.25 (×) and 1.5 mM (*). (b) The secondary plot of reciprocal apparent maximum velocity (1/ V′max) versus the concentration of inhibitor [I], where the abscissa-intercept is −Ki.](/cms/asset/979e81b3-a469-4205-aaca-53a5f79b9541/ienz_a_363435_f0003_b.gif)
Figure 4. Double reciprocal Lineweaver-Burk plots of MT kinetic assays for cresolase reactions with Me-PAPh in 10 mM PBS, 20 °C and pH 6.8, in the presence of different fixed concentrations of 0 (♦), 0.1 (□), 0.2 (▴), 0.4 (×) and 0.75 mM (*) for pyruvic acid (a); 0 (♦), 0.2 (□), 0.4 (▴), 0.8 (×) and 1.2 mM (*) for acrylic acid (b); 0 (♦), 0.4 (□), 1 (▴), 1.5 (×) and 2.5 mM (*) for 2-oxo-butanoic acid (c) and 0 (♦), 0.5 (□), 1 (▴), 1.5 (×) and 2 mM (*) for 2-oxo-octanoic acid (d).
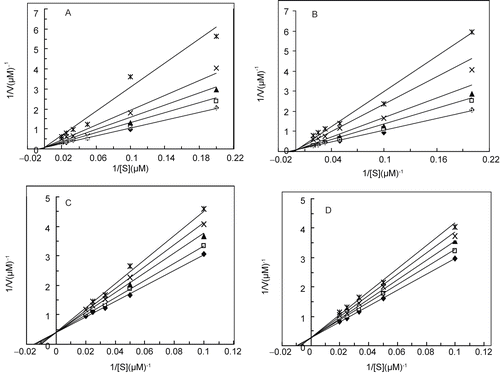
Figure 5. The secondary plots of the slopes for the lines in against the concentration of inhibitor, [I], gives a straight line with an abscissa intercept of inhibition constant (−Ki) for pyruvic acid (a), acrylic acid (b), 2-oxo-butanoic acid (c) and 2-oxo-octanoic acid (d).
![Figure 5. The secondary plots of the slopes for the lines in Figure 4 (a–d) against the concentration of inhibitor, [I], gives a straight line with an abscissa intercept of inhibition constant (−Ki) for pyruvic acid (a), acrylic acid (b), 2-oxo-butanoic acid (c) and 2-oxo-octanoic acid (d).](/cms/asset/cda9901a-b43c-4150-833f-3ada2dc5237f/ienz_a_363435_f0005_b.gif)
The availability of crystallographic data of hemocyanins and recently of sweet potato catechol oxidase showed that in the model of the three-dimensional structure of the tyrosinase family, the catalytic copper center is accommodated in a central four-helix-bundle located in a hydrophobic pocket close to the surface [Citation35]. In the cases of hemocyanine, the most conservative central domain that is also found in the tyrosinase family, the two copper ions in the active site are labeled as CuA and CuB. They are not equivalent and are likely to play a different role in biological function. The CuA copper center seems to be more accessible to the solvent in the binuclear site controls the reactivity of the site and whereas the second copper center (CuB) plays a complex role in controlling the local conformation and electrostatic effects in the oxygen cycle [Citation36]. It is proposed, that there is a physical difference in the docking of mono- and o-diphenols to the tyrosinase active site and that this difference would be an essential determinant for the course of the catalytic cycle. Thus monophenols would dock to CuA and o-diphenols to CuB due to the orientation of one of their hydroxyl groups [Citation6,Citation37]. Wilcox and colleagues proposed detail of carboxylic acid effects on Neurospora tyrosinase [Citation38]. Monophenols are proposed to bind only to the oxyform of tyrosinase and benzoic acid is believed to function as a monophenol analog, binding to one copper ion of the binuclear site via its carboxylate group [Citation38].
In the case of acid derivatives, the mechanism of inhibition involves the formation of copper-carboxylic acid complex at binuclear copper site of the enzyme [Citation10,Citation39]. P-coumaric acid and its corresponding dihydroxy derivative, caffeic acid bind to the free copper through their carboxylic terminal that is attached to a sp2 carbon. [Citation40-Citation41]. In this study, pyruvic, acrylic, 2-oxo-butanoic and 2-oxo-octanoic acids, with a similar carboxylic moiety attached to a sp2 carbon confirm competitive inhibition in cresolase reactions. Ki is a dissociation constant of the enzyme-inhibitor complex and its values increase by increasing the length of the aliphatic tail of the inhibitor. Such increasing m y be related to changes in copper chelating effect of carboxylic moiety with different substitution in the tail (). In our previous study same manner of increasing in Ki was observed for n-butyl, n-hexyl and n-octyl dithiocarbamates, respectively, in MT inhibition [Citation20]. In the aliphatic carboxylic acids of this study the carboxylic moiety attached to sp2 carbon show a proper coordination and compete with monophenols in their accessibility for the copper (more probable CuA) in the active site of the enzyme. Thus, it is conceivable that binding of these acids can completely block the cresolase reaction, by preventing monophenol binding to the enzyme by a competitive mode of inhibition. Such an inhibition pattern has not seen for propanoic acid as a saturated aliphatic acid without a sp2 carbon bound to its carboxylic moiety.
Overall conclusion from different mode of inhibition, competitive for pyruvic, acrylic, 2-oxo-butanoic and 2-oxo-octanoic acids, and uncompetitive for propanoic acid may be related to docking of the former compounds to the copper in the active site and competition with monophenol substrate in the cresolase reactions. Their carboxylic terminal that is attached to a sp2 carbon should be properly coordinated to the copper. Propanoic acid without a sp2 carbon showed uncompetitive mode of inhibition, hence supporting our assumption. Activation by such acids in catecholase reactions need more investigation especially from an allosteric point of view.
Acknowledgements
The financial support provided by the Research Council of the University of Tehran is gratefully acknowledged.
Declaration of interest: The authors report no conflicts of interest. The authors alone are responsible for the content and writing of the paper.
References
- Mason HS. Mechanisms of oxygen metabolism, In: Advance Enzymol, F.F. Nord, editor. NewYork, Academic Press, (1957); pp:79–234.
- Yasunobu KT. In: Pigment cell biology, M. Gordon, editor. NewYork, Academic Press (1959); pp 583.
- Raper HS. The anaerobic oxidases. Physiol Rev (1928); 8: 245–282.
- Priestly GC. Molecular Aspects of Dermatology. Wiley: Chichester, U.K., (1993).
- Martinez MV, Whitaker JR. The biochemistry and control of enzymatic browning. Trends Food Sci Technol (1995); 6:195–200.
- Duckworth HW, Coleman JE. Physicochemical and kinetic properties of mushroom tyrosinase. J Biol Chem (1970); 245:1613–1625.
- Kuttner R, Wagreich H. Some inhibitors of mushroom catecholase. Arch Biochem Biophys (1953); 43:80–87.
- Krueger RC. The effect of beta-keto acids on the action of tyrosinase. Arch Biochem (1955);56:394–404.
- Martinez-Cayuela M, Plata MC, Faus MJ, Gil A. Effect of some phenolic carboxylic acids on cherimoya (Annona cherimolia) polyphenoloxidase activity. J Sci Food and Agric (1988);45:215–222.
- Pifferi PG, Baldassari L, Cultrera R. Inhibition of carboxylic acids of an o-diphenol oxidase from Prunus avium fruits. J Sci Food Agric (1974);25:263–270.
- Mishima Y, Oyama Y, Kurimoto M. Enzyme formation suppressing carboxylic acids. Eur Pat Appl (1989); pp:31.
- Kato H, Shimizu M, Ozasa Y. Cosmetics containing unsaturated fatty acids, antioxidants, amino acids, and polybasic acids. (Sunstar, Inc., Japan). Jpn Kokai Tokkyo Koho (1989); pp:6.
- Prota G. Melanins and melanogenesis, San Diego, Academic Press, (1992).
- Haghbeen K, Saboury AA, Karbassi F. Substrate share in the suicide inactivation of mushroom tyrosinase. Biochim Biophys Acta (2004);1675:139–146.
- Shareefi Borojerdi S, Haghbeen K, Karkhane AA, Fazli M, Saboury AA. Successful resonance raman study of cresolase activity of mushroom tyrosinase. Biochem Biophys Res Commun (2004);314:925–930.
- Gheibi N, Saboury AA, Haghbeen K, Moosavi-Movahedi AA. Activity and structural changes of mushroom tyrosinase induced by n-alkyl sulfates. Colloids and Surface B: Biointerfaces (2005); 45:104–107.
- Gheibi N, Saboury AA, Haghbeen K, Moosavi-Movahedi AA. The effect of some osmolytes on the activity and stability of mushroom tyrosinase. J Biosci (2006);31:355–362.
- Gheibi N, Saboury AA, Haghbeen K. Substrate Construes the Copper and Nickel Ions Impacts on the mushroom tyrosinase activities. Bull Korean Chem Soc (2006);27:642–648.
- Karbassi F, Saboury AA, Hassan MT, Khan Iqbal Choudhary M, Saifi ZS. Mushroom tyrosinase inhibition by two potent uncompetitive inhibitors. J Enz Inhib Med Chem (2004);19:349–353.
- Gheibi N, Saboury AA, Mansury-Torshizy H, Haghbeen K, Moosavi-movahedi A.A. The inhibition effect of some n-alkyl dithiocarbamates on mushroom tyrosinase. J Enz Inhib Med Chem (2004);20:393–399.
- Saboury AA, Zolghadri S, Haghbeen K, Moosavi-movahedi A.A. The inhibitory effect of benzenethiol on the cresolase and catecholase activities of mushroom tyrosinase. J Enz Inhib Med Chem (2006); 21, 711–717.
- Haghbeen K, Tan EW. Facile synthesis of catechol azo dyes. J Org Chem (1998); 63, 4503–4505.
- Haghbeen K, Tan EW. Direct spectrophotometric assay of mono-oxygenase and oxidase activities of mushroom tyrosinase in the presence of synthetic and natural substrates. Anal Biochem (2003); 312, 23–32.
- Penafiel R, Galindo JD, Pedreno E, Lozano JA. The process for the activation of frog epidermis pro-tyrosinase. Biochem J (1982); 205, 397–404.
- Espin JC, Harry JW. Kinetics of Activation of Latent Mushroom (Agaricus bisporus) Tyrosinase by Benzyl Alcohol. J Agric Food Chem (1999); 47(9), 3503–3508.
- Jime¢nez M, Garcı¢a-Carmona F. The effect of sodium dodecyl sulphate on polyphenol oxidase. Phytochemistry (1996); 42, 1503–1509.
- Jime¢nez-Cervantes C, Garcı¢a-Borro¢n JC, Lozano JA, Solano F. Effect of detergents and endogenous lipids on the activity and properties of tyrosinase and its related proteins. Biochim Biophys Acta (1995); 1243, 421–430.
- Asada N, Fukumitsu T, Fujimoto K, Masuda K. Activation of pro- phenoloxidase with 2-propanol and other organic compounds in Drosophila melanogaster. Insect Biochem Mol Biol (1993); 23, 515–520.
- Robinson SP, Dry IB. Broad bean leaf polyphenol oxidase is a 60-kilodalton protein susceptible to proteolytic cleavage. Plant Physiol (1992); 99, 317–323.
- Tataral Y, Namba1 T, Yamagata Y, T Yoshida, Uchida T, Ichishima E. Acid activation of protyrosinase from Aspergillus oryzae: homo-tetrameric protyrosinase is converted to active dimers with an essential intersubunit disulfide bond at acidic pH. Pigment Cell Melanoma Res (2007); 21, 89–96.
- Hearing VJ, Ekel TM. Mammalian tyrosinase: a comparison of tyrosine hydroxylation and melanin formation. Biochem J (1976);157:549–557.
- Monod J, Changeux P, Jacob F. Allosteric proteins and cellular control systems. J Mol Biol (1963); 6: 306–329.
- Cornish-Bowden A. Fundamentals of Enzyme Kinetics. (1995); pp.203–237, Portland Press.
- Alijanzadeh M, Saboury AA, Mansuri-Torshizi H, Haghbeen K, Moosavi-Movahedi AA. The inhibitory effect of some new synthesized xanthates on mushroom tyrosinase activities. J Enz Inh Med Chem (2007); 22, 239–246.
- Klabunde T, Eicken C, Sacchettini JC, Krebs B. Crystal structure of a plant catechol oxidase containing a dicopper center. Nat Struct Biol (1998);5:1084–1090.
- Ling J, Nestor LP, Czeruszewicz RS, Spiro TC, Fraczkiewicz R, Sharma KD, Loehr TM, Sanders-Loehr J. Common oxygen binding site in hemocyanins from arthropods and molluse: Evidence from Raman spectroscopy and normal coordinate analysis. J Am Chem Soc (1994);116:7628–7691.
- Olivares C, Garcia-Boron JC, Solano F. Identification of active site residues involved in metal cofactor binding and stereospecific substrate recognition in mammalian tyrosinase: Implication to the catalytic cycle. Biochemistry (2002);41:679–686.
- Wilcox DE, Porras AG, Hawang YT, Lerch K, Winker ME, Solomon EI. Substrate analogue binding to coupled binuclear copper active site in tyrosinase. J Am Chem Soc (1985);107:4015–4027.
- Conard JS, Dawson SR, Hubbard ER, Meyers TE, Strothkamp KG. Inhibitor binding to the binuclear active site of tyrosinase: Temperature, pH, and solvent deuterium isotope effects. Biochemistry (1994);33:5739–5744.
- Janovitz-Klapp AH, Richard FC, Goupy PM, Nicolas JJ. Inhibition studies on apple polyphenol oxidase. J Agric Food Chem (1990);38:926–931.
- Janovitz-Klapp A, Richard F, Nicolas JJ. Polyphenol oxidase from apple: Partial purification and some properties. Phytochemistry (1989);28:2903–2907.