ABSTRACT
Introduction
Anti-neuraminidase (NA) immunity correlates with the protection against influenza virus infection in both human and animal models. The aim of this review is to better understand the mechanism of anti-NA immunity, and also to evaluate the approaches on developing NA-based influenza vaccines or enhancing immune responses against NA for current influenza vaccines.
Areas covered
In this review, the structure of influenza neuraminidase, the contribution of anti-NA immunity to protection, as well as the efforts and challenges of targeting the immune responses to NA were discussed. We also listed some of the newly discovered anti-NA monoclonal antibodies and discussed their contribution in therapeutic as well as the antigen design of a broadly protective NA vaccine.
Expert opinion
Targeting the immune response to both HA and NA may be critical for achieving the optimal protection since there are different mechanisms of HA and NA elicited protective immunity. Monoclonal antibodies (mAbs) that target the conserved protective lateral face or catalytic sites are effective therapeutics. The epitope discovery using monoclonal antibodies may benefit NA-based vaccine elicited broadly reactive antibody responses. Therefore, the potential for a vaccine that elicits cross-reactive antibodies against neuraminidase is a high priority for next-generation influenza vaccines.
1. Introduction
Influenza is a respiratory disease, caused by influenza viruses, resulting in 290,000–650,000 annual deaths worldwide according to the World Health Organization (WHO) [Citation1,Citation2]. Vaccination is the recommended measurement for controlling influenza virus infections in humans during annual outbreaks. The hemagglutinin (HA) and neuraminidase (NA) are two surface glycoproteins on the surface of virions, and both are targets for vaccine development. Compared to HA, NA is the second-most abundant surface glycoprotein, often undergoes less antigenic drift compared to the HA protein for both H1N1 and H3N2 subtype influenza viruses [Citation3]. Multiple subtypes of HA share the same subtype of NA (e.g. N1 NAs are usually found in H1 and H5 viruses, while N2 NAs are usually found in H1, H2, H3 and H9 viruses). Anti-NA antibodies potentially have broader cross reactivity to heterologous strains than anti-HA antibodies, indicating its value as a supplement to anti-HA antibodies in the prevention against novel influenza viruses [Citation4]. For example, the seasonal influenza vaccine induced partial immunity against lethal H5N1 challenge and complete protection can be achieved with use of adjuvants [Citation5]. Therefore, by targeting only two antigenic subtypes of NA (N1 and N2), the protective breadth is widened to cover human seasonal epidemics strains (H1N1 and H3N2) and potential pandemic strains (H5N1, H2N2, H5N2, H7N2, and H9N2), indicating the potential of NA to be a complementary component in a multivalent universal vaccine with broad immune reactivity and longer-term protection [Citation5,Citation6].
On June 7 of 2023, we conducted a literature search of the PubMed with terms: influenza neuraminidase antibodies and influenza neuraminidase vaccine. By going through the title and abstract for influenza neuraminidase vaccine development and antibody responses, a total of 123 articles were selected from database. In this review, we aim to cover the structure of NA, immunity provided by NA, approaches and challenges in developing anti-NA immunity, and NA-specific broadly protective monoclonal antibodies in therapeutics as well as in influenza universal vaccine development.
2. The neuraminidase of influenza
The neuraminidase is a type II membrane-anchored homo-tetramer glycoprotein on the surface of the influenza virions. The protein is 450–470 amino acids in length, depending upon the subtype and host-lineage [Citation7]. Each NA monomer consists of a head domain, a stalk domain, a transmembrane domain, and a cytoplasmic tail (). The globular head is supported by a thin stalk portion which stands on the surface of virion. The stalk connects to the transmembrane domain through the apical transport, and lastly the transmembrane domain reaches the intra-virion region with a cytoplasmic tail [Citation8,Citation9]. The NA head region but no other regions (stalk, transmembrane domain, and cytoplasmic tail) has been successfully crystallized. The NA head protomer appears to be a six-bladed propeller three-dimensional (3D) structure as revealed by X-ray crystallography [Citation10]. Both intrachain and interchain disulfide bonds as well as the internal protein interactions are all involved to form the homo-tetramer conformation [Citation11–13].
Figure 1. Structure of neuraminidase and its catalytic sites. Reproduced with permission from Sarah Creytens et al. [Citation113].
![Figure 1. Structure of neuraminidase and its catalytic sites. Reproduced with permission from Sarah Creytens et al. [Citation113].](/cms/asset/d10ef85a-5404-4f09-ad52-a74c0fd41079/ierv_a_2343689_f0001_oc.jpg)
The catalytic site locates in the head domain of each promotor, orientating away from the center of the tetramer (). The catalytic site (sialic acids direct contact residues and framework residues) is highly conserved and is the target of antiviral drugs and monoclonal antibody development. Conversely, the head of some avian-like but not human-like NA subtypes, also contains a secondary SA⍺-2,3 Gal-preferential sialic acid-binding site (2SBS), which is different from the HA binding site [Citation14–17]. Avian-like NAs bearing this functional 2SBS exhibited higher enzyme activity in cleaving multivalent substrates than those NAs of human-like, indicating a potential adaptation from avian influenza to human influenza viruses [Citation18]. The ‘Bind and trans-cleave’ or ‘Bind and transfer’ models were proposed as the possible mechanism of how this 2SBS helps to shuttle sialic acid terminals into the cleavage active site [Citation19,Citation20]. This 2SBS may also contribute to the balance between HA and NA since the compensatory mutations in the HA protein were detected if there have been mutations within the 2SBS [Citation18]. Additionally, calcium ions are also important for enzymatic activity and thermostability. For some NA subtypes, at least four Ca2+ binding sites are needed to maintain the thermostability [Citation21–25]. Although, the structure of the NA stalk domain remains unknown, the length of this domain varies. The length of the stalk alters the enzymatic activity by adjusting the NA accessibility to bind to sialic acid terminals on the cell surface [Citation26–29]. Alternatively, there are differences in NA globular head exposure and alter viral infectivity and transmission, as well as immunogenicity of the protein [Citation28,Citation30–34]. The NA proteins isolated from avian hosts may contain a deletion of ~ 20 to 30 amino acids in the stalk region as a compensatory deletion for poultry adaptation from aquatic avian hosts [Citation26,Citation28,Citation29].
The surface of the NA protein (head and stalk) may be highly glycosylated depending on the specific amino acid sequence, the host, and the evolutionary lineage of the virus. Depending on the sites and domain, the glycosylation motifs may contribute to NA structure, activity, specificity, and thermostability [Citation35] as well as the antigenicity and immunogenicity. The addition of a glycosylation site increases the ability of the virus to avoid the immune system by sterically hindering antibodies from binding to immunogenic antigenic epitopes and also making the protein inaccessible to antiviral drugs [Citation36–43]. In support of this, HA proteins without the structurally nonessential glycans elicit antibodies with better binding affinity and neutralization activity against influenza subtypes than the fully glycosylated HA proteins [Citation44]. Removal of glycans at the top of neuraminidase results in improved protection [Citation38]. The addition of N-glycans decreases the overall stability of the N1 NA, but does not affect N2 NA stability [Citation45]. The neuraminidase of A(H3N2) influenza viruses that have circulated since 2016 are antigenically distinct from the A/Hong Kong/4801/2014 vaccine strain used in the previous season due to amino acid mutations at NA residues 245, 247 (S245N/S247T; introducing an N-linked glycosylation site at residue 245) and 468 [Citation40]. In addition, the glycosylation at 245 is also associated with decreased virus replication in human nasal epithelial cells (hNECs) and decreased enzymatic activity of H3N2 clade 3c.2a viruses. The addition of this glycosylation site alters virus replication, enzymatic activity and inhibitory antibody binding [Citation41]. Conversely, losing the glycosylation motif at residue 329 and the E344 in the neuraminidase of H3N2 influenza viruses contribute to antigenic drift [Citation43]. For sites not protected by glycans, the changes to the lateral surface of the NA head result in antigenic drift, which also contributes to evasion from the host immune system [Citation46].
3. Anti-neuraminidase immunity contributes to protection
Both the innate and adaptive immune systems play important roles in controlling and clearing the viral infection (reviewed in [Citation47,Citation48]). Upon detection of foreign antigens, the innate immune system is activated to produce and release more cytokines and chemokines in order to recruit more immune cells (e.g. monocytes, neutrophils, blood borne dendritic cells, and natural killer cells) to the infection site (reviewed in [Citation49,Citation50]). Antigen-presenting to naïve and memory T-cells by antigen-presenting cells (APCs) is a major step for inducing adaptive immunity against viral antigens as this activates both CD4+ and CD8+ T-cells. The activation of adaptive immune system is important for clearance of influenza virus and prevention of reinfection [Citation51,Citation52]. Thus, influenza virus infection builds strong, but strain-specific immunity and memory against influenza viruses by inducing both humoral and cellular immune responses. And influenza virus infection induces balanced immune responses by eliciting antibody responses to both HA and NA proteins [Citation53]. Inhibiting antibodies to the NA protein are created and reach peak NAI titers, as soon as 7 days post the onset of disease [Citation54]. Compared to influenza virus infection, the immune response induced by influenza vaccination is narrow (reviewed in [Citation53]). Both HA- and NA-specific antibody responses can be induced by influenza vaccination depending on the amount and stability of the antigen contents in the vaccine formula ().
Figure 2. Eliciting NA-specific immunity. the anti-NA immunity can be induced by (i) pulmonary infection with influenza virus, (ii) immunization with variety of vaccines, and (iii) passive immunization with NA-specific polyclonal or monoclonal antibodies. NA-specific immunity provides protection against severe disease with reduced morbidity and mortality, decreased pulmonary virus titers and diminished lung lesions.
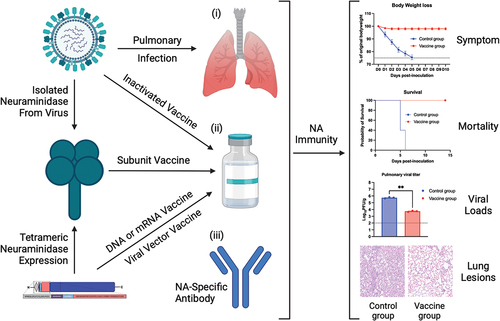
Early studies have demonstrated that the optimal protection can be achieved if both anti-NA and anti-NA immunity were induced [Citation55,Citation56]. Since the types of immunity against HA and NA are different with anti-HA immunity clear virus infection, while the anti-NA immunity is infection permissive but can significantly reduce the incidence of illness or shorten the duration of disease caused by influenza infection in humans [Citation55]. Results from animal studies confirmed this observation, HA immunization prevented manifest infection, while NA immunization reduced the pulmonary virus loads in a dose depended manner [Citation57]. This balanced and broadened immune response to both surface antigens seems critical to fight homotypic and heterotypic influenza virus infections [Citation58–61]. This is true for both type A and B influenza vaccines.
Studies in both animal models and humans have demonstrated the correlation between NA-specific antibodies and decreased risk of infection (). In animal models, immunity directed to NA contributes to decreased pulmonary viral loads thus confer protection against influenza viral infection [Citation57,Citation62,Citation63]. In humans, NA-specific antibodies mitigate clinical symptoms and viral shedding thus further shortened the duration of infection [Citation64]. It was also reported that the NA inhibiting antibody titers superior to both the HA inhibiting antibody titer and the HA-stem binding antibody titer in reducing viral shedding and symptom severity in humans [Citation65–68]. The gene expression profile in the peripheral blood leukocytes seems different as in the presence of anti-NA, anti-HA head or anti-HA stalk antibodies during infection, this may be associated with the types of protection offered by these antibodies [Citation69].
Table 1. NA-based vaccine studies.
Antibodies targeting the conserved active site or lateral surface of NA may inhibit the enzymatic activity by direct binding or steric hinderance which may serve as the main mechanism of action for anti-NA antibodies [Citation70,Citation71]. Other mechanisms such as antibody-dependent cell-mediated cytotoxicity (ADCC) or complement-dependent cytotoxicity were also identified [Citation72]. It has been shown that both N1 or N2 viral proteins can boost ADCC antibodies in H1N1 or H3N2 infected people [Citation73]. And the reduced protective efficacy of anti-NA immune sera in Fc receptor common gamma-chain deficient mice further confirmed the role of Fc receptor in NA immunity [Citation74].
4. NA-based vaccine development
NA proteins isolated from influenza virions is as immunogenic as HA protein and induces robust antibody response after vaccination [Citation57]. In addition, there is no antigenic competition between N1 and N2 when they were administered together [Citation75]. NA proteins purified from intact influenza viruses, baculovirus, yeast, or HEK-293T cell expression systems were immunogenic and safe when administer in humans and animals [Citation24,Citation38,Citation76–87]. A more balanced and broadened immune response can be achieved through supplementing the conventional influenza vaccines with purified viral neuraminidase [Citation58,Citation60] or using the disassociated HA and NA protein from intact virions or recombinant HA and NA protein produced in baculovirus as the vaccine [Citation59,Citation61,Citation88].
Structure design of the neuraminidase plays a larger role in inducing protective antibody response than the enzyme activity. The recombinant NA (rNA) possessing the full ectodomain and the tetramerization motif from the human vasodilator-stimulated phosphoprotein was superior to that of rNA with the same tetramerization motif, but higher enzymatic activity in eliciting protective immune responses [Citation84]. A measles virus phosphoprotein tetramerization domain, as well as the addition of cysteines in the stalk domain, can stabilize the influenza virus rNA vaccine candidate and enhance the protection from virus challenge in the mouse model [Citation83,Citation85]. Besides stabilizing the NA tetramer, swapping the 5’ and 3’ terminal packaging signals of the HA and NA genomic segments results in more NA and less HA expressed in rescued influenza viruses. And the anti-NA antibody response was enhanced by using the modified viruses as antigen [Citation86].
In addition to subunit neuraminidase vaccines, neuraminidase-expressing DNA vaccines used to immunize mice elicited optimal protective immunity suckling offspring following vaccination in mother mice to only when mice were co-administratered of both neuraminidase-expressing and hemagglutinin-expressing DNA vaccines [Citation89]. The modified vaccinia virus Ankara and vesicular stomatitis virus-based replicons expressing the influenza neuraminidase as the vaccine effectively controlled influenza virus following vaccination [Citation90,Citation91]. Vaccination with virus-like particles (VLPs) expressing both HA and NA induced the highest CD4(+) T cell, CD8(+) T cell, and germinal center B cells, while strongly limiting inflammatory cytokine production in the lungs compared to other VLP immunizations [Citation92]. There was no significant difference in anti-NA IgG responses against N1 and N2 antigen following vaccination with bivalent N1 and N2 VLPs vaccines and these VLPs vaccines protected against lethal H1N1 and H3N2 viruses [Citation93]. The potential of intranasally delivered NA vaccines elicited broader protective immunity in mice than the HA-based vaccines. The NA-specific IgA in the upper respiratory tract induced by intranasal immunization recognized more epitopes than did the NA-specific IgG and IgA in plasma, indicating increased cross-protection [Citation82]. The computationally optimized broadly reactive antigen (COBRA) methodology helps to overcome the diversity of NA antigenicity, enhancing the broadly reactive antibody responses in both mice and ferrets [Citation80,Citation81].
5. Challenges in inducing NA-specific immunity
Antiviral drugs and therapeutic antibodies usually target to NA catalytic site since the residues within this site are highly conserved. However, the proportion of broadly inhibitory anti-NA antibodies induced by a NA-containing vaccine remains unknown. One of the challenges in developing a universal influenza vaccine is the antigenicity diversity of protective antigens (e.g. HA and NA). As compared to HA, NA usually has a lower rate of evolution but still can be characterized into two major phylogenetic groups (Group 1: N1, N4, N5, and N8; Group 2: N2, N3, N6, N7) and two special categories: bat-like NA (N10 and N11) and influenza B NA [Citation7,Citation94,Citation95]. Among which, N1 and N2 subtype NAs are found to have both pandemic and epidemic potentials while the influenza B NA is only associated with the epidemics of influenza in humans. The N1 subtype NA can be further characterized into three main lineages: N1.1, N1.2, and N1.3, corresponding to avian, human, and classical swine lineage [Citation96]. The N1.1 avian lineage is more diverse in hosts, while the other two lineages are not. The N1 subtype NAs of avian HxN1, the highly pathogenic avian H5N1, the Eurasian swine HxN1, and human 2009 pandemic-like H1N1 influenza viruses all belong to N1.1 avian lineage. While the N1 subtype NAs of human seasonal H1N1 (prior to the 2009 pandemic) influenza viruses and swine H1N1 influenza viruses from North American belong to N1.2 human lineage and N1.3 classical swine lineage, respectively [Citation39,Citation96,Citation97]. NAs of N1 subtype can be further categorized into more sub-lineages based on geography and host indicates the antigenicity diversity for N1 NAs [Citation39,Citation97]. The N2 subtype NA can be characterized into two main lineages: N2.1 and N2.2, corresponding to avian and human/swine lineage [Citation96]. The N2.1 avian lineage is comprised of the N2.1a (corresponding to many avian influenza viruses circulating worldwide) and N2.1b (corresponding to many H9N2 subtype avian influenza viruses circulating in Asia after 1994). The N2.2 mainly corresponded to human influenza viruses and swine influenza viruses. And the swine influenza viruses is highly diversified, distributed among the human influenza viruses [Citation96].
The frequencies of NA antibody responses induced by conventional influenza vaccines are lower than the frequencies of HA antibody responses due to the competition between HA and NA, as well as the low content of NA caused by low stability of the protein [Citation88]. The NA activity in vaccine preparations wane quickly during storage, thus resulting in low frequencies of neuraminidase antibody responses after vaccination [Citation98]. Also, the enzyme activity of influenza NA does not relate to the level of the antibody responses, but to the amount of the tetrameric NA in the vaccine [Citation24]. High doses of influenza vaccine induce significantly more NA antibody than the standard vaccine doses in vaccinated elderly subjects, indicating that the amount of NA content in the vaccine is critical in inducing NA-specific antibodies [Citation99]. Unfortunately, the NA potency is not standardized in current influenza vaccines, thus the anti-NA immunity induced by seasonal influenza vaccines varies due to various amount and stability of the NA content in the vaccine [Citation100–103]. Thus, conventional influenza vaccines (inactivated or live-attenuated influenza vaccines) are not optimal in eliciting NA-specific antibody response due to various issues, including standardization and stability of NA antigen in the vaccine as well as the strain-specific immunity induced by the vaccine. Fortunately, the knowledge gained in studying NA-specific monoclonal antibodies gave us more information for designing a broadly protective NA antigen that could be a component for a universal influenza vaccine. Besides, the tetrameric design of the recombinant influenza NA antigen is also crucial for eliciting protective antibody responses and the use of the full ectodomain and the tetramerization motif from the human vasodilator-stimulated phosphoprotein contributes to the better protection of the rNA vaccine [Citation84].
The NA specific antibody responses are influenced by the age of the individual, as well as the NA subtype elicited [Citation104]. Influenza-binding antibodies are present in the human population and termed ‘immune imprinting’ [Citation105], due to the prevalence of circulating influenza viruses and wide use of the vaccine. This influenza immune imprinting has long-lasting effects on the immune responses to subsequent influenza infections and vaccination, usually boosting the antibody responses to the cross-reactive epitopes on HA and NA proteins [Citation106,Citation107]. Therefore, there is an improved antibody responses against neuraminidase for the ‘NA-specific’ vaccine immunogen with a HA to which the study population has not been previously exposed [Citation108]. Conversely, the NA antibody responses to influenza vaccines can be suppressed if subjects receiving the vaccine are immunologically primed to its hemagglutinin component [Citation109]. This may be due to the intravirionic HA that is dominant over NA in both B- and T-cell priming [Citation110]. This intravirionic antigenic competition can be eliminated by dissociating the influenza HA and NA, with the higher doses of purified NA to achieve a more balanced HA and NA immune response [Citation58,Citation60,Citation88].
6. Anti-neuraminidase antibodies and epitopes
The NA of influenza viruses are targeted by three NA inhibitors (oseltamivir, zanamivir, and peramivir) that are licensed in the U.S. for the treatment and prophylaxis of influenza (https://www.cdc.gov/flu/professionals/antivirals/summary-clinicians.htm). The conserved and protective epitopes may be effective targets for antibodies selected for development as therapeutic agents against circulating influenza viruses.
On one hand, many monoclonal antibodies (mAbs) that targeting either the catalytic site or lateral surface as well as the underside of NA protein have been discovered (reviewed in [Citation111,Citation112]). For example, the mAb CD6 binds to a conserved 30-amino-acid spanning epitope on the lateral face of a NA dimer [Citation113]. Conversely, the mAb N1-C4 bound to a conserved conformational epitope in N1 subtype NA of human 2009 pandemic-like H1N1 and avian H5N1 influenza viruses, and has potent in vitro and in vivo antiviral activities against these two subtypes of influenza viruses [Citation114]. The human monoclonal antibody DA03E17 is directly targeting to the enzyme active site, and has shown both in vitro and in vivo protection against infection with influenza viruses with NAs of both group 1 and 2 as well as both lineages of influenza B [Citation115]. And a monoclonal antibody, Z2B3, isolated from an H7N9-infected patient that exhibited cross-reactivity to both N9 (group 2) and a broad range of seasonal and avian N1 (group 1) proteins [Citation116]. More recently, a mAb FNI9 that targeting the catalytic site of NA was described to have a broader neutralizing spectrum of both seasonal influenza A and B viruses [Citation117]. Instead of targeting the NA active site, three mAbs were found to recognize the highly conserved underside of NA head domain and effecting through both Fc effector functions and NA inhibition by steric hindrance [Citation118]. All these mAbs inhibit NA activity in enzyme-linked lectin assay (ELLA) with large substrates (e.g. Fetuin), while only the mAbs that are targeting the catalytic site inhibit NA activity in small substrate (MUNANA or NA-STAR) assays [Citation111].
On the other hand, the key residues that affect binding of these mAbs to the epitopes on NA have also been identified. For example, glutamic acid residue 311 in the N1 NA was found to be critical for the NA binding and antiviral activity of monoclonal antibody N1-C4 [Citation114]. And a predicted glycosylation site at amino acid 386 in NA emerged in 2013 viruses and associated with decreased in vitro inhibition of enzyme activity of polyclonal antisera [Citation39]. The residue at 432 in N1 subtype NA and the residue at 102 in heavy chain of mAb Z2B3 seems to be important for the binding activity of Z2B3 due to the salt bridge formed between K432 in NA and D102 in Z2B3. Unfortunately, over 99% of seasonal influenza isolates after 2013 bearing the substitution K432E results in no binding activity for Z2B3. While the salt bridge can be reversed between E432 in NA and R102 in Z2B3 and the binding and inhibition of Z2B3 to N1 with E432 can be restored [Citation116]. Besides, the D107 in FNI9 heavy chain complementarity-determinant region 3 (HCDR3) was confirmed to mimic the interaction of the sialic acid carboxyl group with the three highly conserved arginine residues (R118, R292 and R371) of the neuraminidase catalytic site [Citation117]. More mutations (K199E/T, E258K, A272D, and S331N) were identified by sequencing the escape mutant viruses. Of them, K199 and E258 in NA have potent influence on binding and inhibition of mAbs. Importantly, a wildtype strain bearing E258K in NA has shown resistance against numerous antibodies tested [Citation119]. Lastly, the mAbs targeting to N2 subtype NA of H9N2 influenza viruses from 1999 to 2019 can be characterized into three main groups: group 1, group 2, and group 3. And each group of mAbs recognizes a specific pattern of residue(s) in N2 subtype NA [Citation120]. All these identified key residues should be taken into consideration for the design of broadly protective influenza vaccines that target influenza virus NA.
7. Expert opinion
Influenza viruses are common respiratory pathogens that cause seasonal influenza in humans, resulting in severe disease with high morbidity and mortality annually. Although, all types of influenza virus vaccines induced robust and comparable homotypic anti-hemagglutinin antibody responses, the effectiveness and coverage of the seasonal influenza vaccines are low, due to the strain dependent manner of vaccine formulation and extensive antigenic drift of influenza viruses. The neuraminidase of influenza viruses evolves slower compared to hemagglutinin, showing less antigenicity diversity and broader cross-reactivity among subtypes. Except for that, different types of neuraminidases shared the conserved catalytic site and framework residues. These features make neuraminidase an attractive target for a universal influenza vaccine.
Neuraminidase is as immunogenic as hemagglutinin, but was not standardized in current seasonal influenza vaccines, resulting in poor anti-NA immune responses. Therefore, the frequencies of anti-NA antibody responses induced by seasonal influenza vaccines were always lower than the frequencies of anti-HA antibody responses, due to the low or no potency of NA presented in the vaccines. Although, this can be overcome by use of subunit influenza vaccine with adjuvants or supplementing seasonal influenza vaccine with additional neuraminidase. Other approaches also like stabilizing neuraminidase tetramer by using a measles virus phosphoprotein tetramerization domain or increase expression of neuraminidase by swapping the 5’ and 3’ terminal packaging signals of the hemagglutinin and neuraminidase genomic segments have been tested.
As a potential target for a universal influenza vaccine, NA-based vaccines elicit virus infection permissive antibodies with broader breadth. Targeting the immune response to both hemagglutinin and neuraminidase may be critical for achieving the optimal protection since there are different mechanisms of hemagglutinin and neuraminidase elicited protective immunity. The neuraminidase is also a druggable target, and three NA inhibitors have been licensed in the U.S. for the treatment and prophylaxis of influenza. Monoclonal antibodies (mAbs) that target the conserved protective lateral face or catalytic sites are effective therapeutics. The epitope discovery using monoclonal antibodies may benefit NA-based vaccine elicited broadly reactive antibody responses. Therefore, the potential for a vaccine that elicits cross-reactive antibodies against neuraminidase is a high priority for next-generation influenza vaccines.
Article highlights
The conserved catalytic site and less antigenicity diversity makes neuraminidase an attractive target for a universal influenza vaccine.
Both anti-hemagglutinin and anti-neuraminidase immunity contribute to protection, with anti-hemagglutinin immunity clear virus infection, while the anti-neuraminidase immunity is infection permissive.
Targeting the immune response to both hemagglutinin and neuraminidase may be critical for achieving the optimal protection.
The low potency and stability of neuraminidase in current influenza vaccines are the main challenges in inducing strong immune responses against neuraminidase.
Different approaches that aim to enhance anti-neuraminidase immune responses have been tested.
Isolation and characterization of broadly reactive anti-neuraminidase monoclonal antibodies benefits influenza therapy and discovery of protective epitopes for influenza vaccine development.
Declaration of interests
The authors have no relevant affiliations or financial involvement with any organization or entity with a financial interest in or financial conflict with the subject matter or materials discussed in the manuscript. This includes employment, consultancies, honoraria, stock ownership or options, expert testimony, grants, patents received or pending, or royalties.
Reviewer disclosures
A reviewer on this manuscript has disclosed that their lab receives funding from Sanofi Pasteur to perform preclinical research on influenza vaccines. Peer reviewers on this manuscript have no other relevant financial or other relationships to disclose.
Author contributions
Conceptualization, X. Zhang and T.M.Ross.; formal analysis, X Zhang and T.M.Ross; investigation, X. Zhang; resources, T.M.Ross; data curation, X Zhang; writing – original draft preparation, X. Zhang; writing – review and editing, X. Zhang and T.M.R.; supervision, T.M.Ross; project administration, X. Zhang; funding acquisition, T.M.Ross. All authors have read and agreed to the published version of the manuscript.
Additional information
Funding
References
- Iuliano AD, Roguski KM, Chang HH, et al. Estimates of global seasonal influenza-associated respiratory mortality: a modelling study. Lancet. 2018 Mar 31;391(10127):1285–1300. doi: 10.1016/S0140-6736(17)33293-2
- Paget J, Spreeuwenberg P, Charu V, et al. Global mortality associated with seasonal influenza epidemics: new burden estimates and predictors from the GLaMOR project. J Glob Health. 2019 Dec;9(2):020421.
- Sandbulte MR, Westgeest KB, Gao J, et al. Discordant antigenic drift of neuraminidase and hemagglutinin in H1N1 and H3N2 influenza viruses. Proc Natl Acad Sci USA. 2011 Dec 20;108(51):20748–20753. doi: 10.1073/pnas.1113801108
- Jiang L, Changsom D, Lerdsamran H, et al. Cross-reactive antibodies against H7N9 and H5N1 avian influenza viruses in Thai population. Asian Pac J Allergy Immunol. 2017 Mar;35(1):20–26.
- Rockman S, Brown LE, Barr IG, et al. Neuraminidase-inhibiting antibody is a correlate of cross-protection against lethal H5N1 influenza virus in ferrets immunized with seasonal influenza vaccine. J Virol. 2013 Mar;87(6):3053–3061.
- Chen Z, Kim L, Subbarao K, et al. The 2009 pandemic H1N1 virus induces anti-neuraminidase (NA) antibodies that cross-react with the NA of H5N1 viruses in ferrets. Vaccine. 2012;30(15):2516–2522. doi: 10.1016/j.vaccine.2012.01.090
- Air GM. Influenza neuraminidase. Influenza Other Respir Viruses. 2012 Jul;6(4):245–256. doi: 10.1111/j.1750-2659.2011.00304.x
- Laver WG, Valentine RC. Morphology of the isolated hemagglutinin and neuraminidase subunits of influenza virus. Virology. 1969;38(1):105–119. doi: 10.1016/0042-6822(69)90132-9
- Bateman A, Martin M-J, Orchard S, et al. UniProt: the universal protein knowledgebase in 2021. Nucleic Acids Res.2021 Jan 8;49(D1):D480–D489. doi: 10.1093/nar/gkaa1100
- Bossart-Whitaker P, Carson M, Babu YS, et al. Three-dimensional structure of influenza a N9 neuraminidase and its complex with the inhibitor 2-deoxy 2,3-dehydro-N-acetyl neuraminic acid. J Mol Biol. 1993 Aug 20;232(4):1069–1083. doi: 10.1006/jmbi.1993.1461
- Shtyrya YA, Mochalova LV, Bovin NV. Influenza virus neuraminidase: structure and function. Acta Naturae. 2009 Jul;1(2):26–32. doi: 10.32607/20758251-2009-1-2-26-32
- Ward CW, Colman PM, Laver WG. The disulphide bonds of an Asian influenza virus neuraminidase. FEBS Lett. 1983;153(1):29–33. doi: 10.1016/0014-5793(83)80113-6
- Selimova LM, Zaides VM, Zhdanov VM. Disulfide bonding in influenza virus proteins as revealed by polyacrylamide gel electrophoresis. J Virol. 1982 Nov;44(2):450–457. doi: 10.1128/jvi.44.2.450-457.1982
- Du W, de Vries E, van Kuppeveld FJM, et al. Second sialic acid-binding site of influenza a virus neuraminidase: binding receptors for efficient release. FEBS J. 2020 Dec 14;288(19):5598–5612. doi: 10.1111/febs.15668
- Du W, Guo H, Nijman VS, et al. The 2nd sialic acid-binding site of influenza a virus neuraminidase is an important determinant of the hemagglutinin-neuraminidase-receptor balance. PLOS Pathog. 2019 Jun;15(6):e1007860.
- Varghese JN, Colman PM, van Donkelaar A, et al. Structural evidence for a second sialic acid binding site in avian influenza virus neuraminidases. Proc Natl Acad Sci USA. 1997 Oct 28;94(22):11808–11812. doi: 10.1073/pnas.94.22.11808
- Sun X, Li Q, Wu Y, et al. Structure of influenza virus N7: the last piece of the neuraminidase “jigsaw” puzzle. J Virol. 2014 Aug;88(16):9197–9207.
- Du W, Wolfert MA, Peeters B, et al. Mutation of the second sialic acid-binding site of influenza a virus neuraminidase drives compensatory mutations in hemagglutinin. PLOS Pathog. 2020 Aug;16(8):e1008816.
- Dai M, McBride R, Dortmans J, et al. Mutation of the second sialic acid-binding site, resulting in reduced neuraminidase activity, preceded the emergence of H7N9 influenza a virus. J Virol. 2017 May 1;91(9). doi: 10.1128/JVI.00049-17
- Du W, de Vries E, van Kuppeveld FJM, et al. Second sialic acid-binding site of influenza A virus neuraminidase: binding receptors for efficient release. FEBS J. 2021 Oct;288(19):5598–5612.
- Johansson BE, Brett IC. Variation in the divalent cation requirements of influenza a virus N2 neuraminidases. J Biochem. 2003 Sep;134(3):345–352. doi: 10.1093/jb/mvg151
- Baker NJ, Gandhi SS. Effect of Ca++ on the stability of influenza virus neuraminidase. Arch Virol. 1976;52(1–2):7–18. doi: 10.1007/BF01317860
- Brett IC, Johansson BE. Variation in the divalent cation requirements of influenza a virus N1 neuraminidases. J Biochem. 2006 Mar;139(3):439–447. doi: 10.1093/jb/mvj051
- Giurgea LT, Park JK, Walters KA, et al. The effect of calcium and magnesium on activity, immunogenicity, and efficacy of a recombinant N1/N2 neuraminidase vaccine. NPJ Vaccin. 2021 Apr 6;6(1):48. doi: 10.1038/s41541-021-00310-x
- Wang H, Dou D, Ostbye H, et al. Structural restrictions for influenza neuraminidase activity promote adaptation and diversification. Nat Microbiol. 2019 Dec;4(12):2565–2577.
- Sun Y, Tan Y, Wei K, et al. Amino acid 316 of hemagglutinin and the neuraminidase stalk length influence virulence of H9N2 influenza virus in chickens and mice. J Virol. 2013 Mar;87(5):2963–2968.
- Castrucci MR, Kawaoka Y. Biologic importance of neuraminidase stalk length in influenza A virus. J Virol. 1993 Feb;67(2):759–764. doi: 10.1128/jvi.67.2.759-764.1993
- Blumenkrantz D, Roberts KL, Shelton H, et al. The short stalk length of highly pathogenic avian influenza H5N1 virus neuraminidase limits transmission of pandemic H1N1 virus in ferrets. J Virol. 2013 Oct;87(19):10539–10551.
- Li Y, Chen S, Zhang X, et al. A 20-amino-acid deletion in the neuraminidase stalk and a five-amino-acid deletion in the NS1 protein both contribute to the pathogenicity of H5N1 avian influenza viruses in mallard ducks. PLOS ONE. 2014;9(4):e95539. doi: 10.1371/journal.pone.0095539
- Chen S, Quan K, Wang D, et al. Truncation or deglycosylation of the neuraminidase stalk enhances the pathogenicity of the H5N1 subtype avian influenza virus in mallard ducks. Front Microbiol. 2020;11:583588. doi: 10.3389/fmicb.2020.583588
- Park S, Il Kim J, Lee I, et al. Adaptive mutations of neuraminidase stalk truncation and deglycosylation confer enhanced pathogenicity of influenza a viruses. Sci Rep. 2017 Sep 7;7(1):10928. doi: 10.1038/s41598-017-11348-0
- Bi Y, Xiao H, Chen Q, et al. Changes in the length of the neuraminidase stalk region impact H7N9 virulence in mice. J Virol. 2016 Feb 15;90(4):2142–2149. doi: 10.1128/JVI.02553-15
- Stech O, Veits J, Abdelwhab EM, et al. The neuraminidase stalk deletion serves as major virulence determinant of H5N1 highly pathogenic avian influenza viruses in chicken. Sci Rep. 2015 Aug 26;5(1):13493. doi: 10.1038/srep13493
- Munier S, Larcher T, Cormier-Aline F, et al. A genetically engineered waterfowl influenza virus with a deletion in the stalk of the neuraminidase has increased virulence for chickens. J Virol. 2010 Jan;84(2):940–952.
- Wu CY, Lin CW, Tsai TI, et al. Influenza A surface glycosylation and vaccine design. Proc Natl Acad Sci USA. 2017 Jan 10;114(2):280–285. doi: 10.1073/pnas.1617174114
- Kendal AP. Epidemiologic implications of changes in the influenza virus genome. Am J Med. 1987;82(6):4–14. doi: 10.1016/0002-9343(87)90554-7
- Seitz C, Casalino L, Konecny R, et al. Multiscale simulations examining glycan shield effects on drug binding to influenza neuraminidase. Biophys J. 2020 Dec 1;119(11):2275–2289. doi: 10.1016/j.bpj.2020.10.024
- Martinet W, Saelens X, Deroo T, et al. Protection of mice against a lethal influenza challenge by immunization with yeast-derived recombinant influenza neuraminidase. Eur J Biochem. 1997 Jul 1;247(1):332–338. doi: 10.1111/j.1432-1033.1997.00332.x
- Gao J, Couzens L, Burke DF, et al. Antigenic drift of the influenza A(H1N1)pdm09 virus neuraminidase results in reduced effectiveness of A/California/7/2009 (H1N1pdm09)-specific antibodies. MBio. 2019 Apr 9;10(2). doi: 10.1128/mBio.00307-19
- Wan H, Gao J, Yang H, et al. The neuraminidase of A(H3N2) influenza viruses circulating since 2016 is antigenically distinct from the A/Hong Kong/4801/2014 vaccine strain. Nat Microbiol. 2019 Dec;4(12):2216–2225.
- Powell H, Pekosz A, Lowen AC. Neuraminidase antigenic drift of H3N2 clade 3c.2a viruses alters virus replication, enzymatic activity and inhibitory antibody binding. PLOS Pathog. 2020 Jun;16(6):e1008411. doi: 10.1371/journal.ppat.1008411
- Wang F, Wan Z, Wu J, et al. A cross-reactive monoclonal antibody against neuraminidases of both H9N2 and H3N2 Influenza viruses shows protection in mice challenging models. Front Microbiol. 2021;12:730449. doi: 10.3389/fmicb.2021.730449
- Ge J, Lin X, Guo J, et al. The antibody response against neuraminidase in human influenza a (H3N2) virus infections during 2018/2019 Flu Season: focusing on the epitopes of 329-N-Glycosylation and E344 in N2. Front Microbiol. 2022;13:845088. doi: 10.3389/fmicb.2022.845088
- Wang CC, Chen JR, Tseng YC, et al. Glycans on influenza hemagglutinin affect receptor binding and immune response. Proc Natl Acad Sci USA. 2009 Oct 27;106(43):18137–18142. doi: 10.1073/pnas.0909696106
- Ostbye H, Gao J, Martinez MR, et al. N-Linked glycan sites on the influenza a virus neuraminidase head domain are required for efficient viral incorporation and replication. J Virol. 2020 Sep 15;94(19). doi: 10.1128/JVI.00874-20
- Yasuhara A, Yamayoshi S, Kiso M, et al. Antigenic drift originating from changes to the lateral surface of the neuraminidase head of influenza a virus. Nat Microbiol. 2019 Jun;4(6):1024–1034.
- Chen X, Liu S, Goraya MU, et al. Host immune response to influenza a virus infection. Front Immunol. 2018;9:320. doi: 10.3389/fimmu.2018.00320
- Iwasaki A, Pillai PS. Innate immunity to influenza virus infection. Nat Rev Immunol. 2014 May;14(5):315–328. doi: 10.1038/nri3665
- Mifsud EJ, Kuba M, Barr IG. Innate immune responses to influenza virus infections in the upper respiratory tract. Viruses. 2021 Oct 17;13(10):2090.
- Bahadoran A, Lee SH, Wang SM, et al. Immune responses to influenza virus and its correlation to age and inherited factors. Front Microbiol. 2016;7:1841. doi: 10.3389/fmicb.2016.01841
- Ho AW, Prabhu N, Betts RJ, et al. Lung CD103+ dendritic cells efficiently transport influenza virus to the lymph node and load viral antigen onto MHC class I for presentation to CD8 T cells. J Immunol. 2011 Dec 1;187(11):6011–6021. doi: 10.4049/jimmunol.1100987
- Brown DM, Lee S, Garcia-Hernandez Mde L, et al. Multifunctional CD4 cells expressing gamma interferon and perforin mediate protection against lethal influenza virus infection. J Virol. 2012 Jun;86(12):6792–6803.
- Krammer F. The human antibody response to influenza a virus infection and vaccination. Nat Rev Immunol. 2019 Jun;19(6):383–397. doi: 10.1038/s41577-019-0143-6
- Changsom D, Jiang L, Lerdsamran H, et al. Kinetics, longevity, and cross-reactivity of antineuraminidase antibody after natural infection with influenza a viruses. Clin Vaccine Immunol. 2017 Dec;24(12). doi: 10.1128/CVI.00248-17
- Couch RB, Kasel JA, Gerin JL, et al. Induction of partial immunity to influenza by a neuraminidase-specific vaccine. J Infect Dis. 1974 Apr;129(4):411–420.
- Bosch BJ, Bodewes R, de Vries RP, et al. Recombinant soluble, multimeric HA and NA exhibit distinctive types of protection against pandemic swine-origin 2009 A(H1N1) influenza virus infection in ferrets. J Virol. 2010 Oct;84(19):10366–10374.
- Johansson BE, Bucher DJ, Kilbourne ED. Purified influenza virus hemagglutinin and neuraminidase are equivalent in stimulation of antibody response but induce contrasting types of immunity to infection. J Virol. 1989 Mar;63(3):1239–1246. doi: 10.1128/jvi.63.3.1239-1246.1989
- Johansson BE, Matthews JT, Kilbourne ED. Supplementation of conventional influenza a vaccine with purified viral neuraminidase results in a balanced and broadened immune response. Vaccine. 1998 May;16(9–10):1009–1015. doi: 10.1016/S0264-410X(97)00279-X
- Johansson BE. Immunization with influenza a virus hemagglutinin and neuraminidase produced in recombinant baculovirus results in a balanced and broadened immune response superior to conventional vaccine. Vaccine. 1999 Apr 9;17(15–16):2073–2080.
- Johansson BE, Pokorny BA, Tiso VA. Supplementation of conventional trivalent influenza vaccine with purified viral N1 and N2 neuraminidases induces a balanced immune response without antigenic competition. Vaccine. 2002 Feb 22;20(11–12):1670–1674.
- Johansson BE, Brett IC. Recombinant influenza B virus HA and NA antigens administered in equivalent amounts are immunogenically equivalent and induce equivalent homotypic and broader heterovariant protection in mice than conventional and live influenza vaccines. Hum Vaccin. 2008 Nov;4(6):420–424. doi: 10.4161/hv.4.6.6201
- Schulman JL, Khakpour M, Kilbourne ED. Protective effects of specific immunity to viral neuraminidase on influenza virus infection of mice. J Virol. 1968 Aug;2(8):778–786. doi: 10.1128/jvi.2.8.778-786.1968
- Johansson BE, Kilbourne ED. Programmed antigenic stimulation: kinetics of the immune response to challenge infections of mice primed with influenza inactivated whole virus or neuraminidase vaccine. Vaccine. 1991;9(5):330–333. doi: 10.1016/0264-410X(91)90059-F
- Murphy BM, Kasel A, Chanock RM. Association of serum anti-neuraminidase antibody with resistance to INfluenza in man. N Engl J Med. 1972;286(25):1329–1332. doi: 10.1056/NEJM197206222862502
- Memoli MJ, Shaw PA, Han A, et al. Evaluation of antihemagglutinin and antineuraminidase antibodies as correlates of protection in an influenza A/H1N1 virus healthy human challenge model. MBio. 2016 Apr 19;7(2):e00417–16. doi: 10.1128/mBio.00417-16
- Park JK, Han A, Czajkowski L, et al. Evaluation of preexisting anti-hemagglutinin stalk antibody as a correlate of protection in a healthy volunteer challenge with influenza A/H1N1pdm virus. MBio. 2018 Jan 23;9(1). doi: 10.1128/mBio.02284-17
- Weiss CD, Wang W, Lu Y, et al. Neutralizing and neuraminidase antibodies correlate with protection against influenza during a late season A/H3N2 outbreak among unvaccinated military recruits. Clin Infect Dis. 2020 Dec 15;71(12):3096–3102. doi: 10.1093/cid/ciz1198
- Maier HE, Nachbagauer R, Kuan G, et al. Pre-existing antineuraminidase antibodies are associated with shortened duration of influenza A(H1N1)pdm virus shedding and illness in naturally infected adults. Clin Infect Dis. 2020 May 23;70(11):2290–2297. doi: 10.1093/cid/ciz639
- Walters KA, Zhu R, Welge M, et al. Differential effects of influenza virus NA, HA head, and HA stalk antibodies on peripheral blood leukocyte gene expression during human infection. MBio. 2019 May 14;10(3). doi: 10.1128/mBio.00760-19
- Gilchuk IM, Bangaru S, Gilchuk P, et al. Influenza H7N9 Virus Neuraminidase-Specific Human Monoclonal Antibodies Inhibit Viral Egress and Protect from Lethal Influenza Infection in Mice. Cell Host Microbe. 2019 Dec 11;26(6):715–728 e8. doi: 10.1016/j.chom.2019.10.003
- Stadlbauer D, Zhu X, McMahon M, et al. Broadly protective human antibodies that target the active site of influenza virus neuraminidase. Science. 2019 Oct 25;366(6464):499–504. doi: 10.1126/science.aay0678
- Krammer F, Palese P. Advances in the development of influenza virus vaccines. Nat Rev Drug Discov. 2015 Mar;14(3):167–182. doi: 10.1038/nrd4529
- Valkenburg SA, Fang VJ, Leung NH, et al. Cross-reactive antibody-dependent cellular cytotoxicity antibodies are increased by recent infection in a household study of influenza transmission. Clin Transl Immunology. 2019;8(11):e1092. doi: 10.1002/cti2.1092
- Kim YJ, Ko EJ, Kim MC, et al. Roles of antibodies to influenza a virus hemagglutinin, neuraminidase, and M2e in conferring cross protection. Biochem Biophys Res Commun. 2017 Nov 4;493(1):393–398. doi: 10.1016/j.bbrc.2017.09.011
- Johansson B, Kilbourne E. Immunization with purified N1 and N2 influenza virus neuraminidases demonstrates cross-reactivity without antigenic competition. Proc Nat Acad Sci. 1994;91(6):2358–2361. doi: 10.1073/pnas.91.6.2358
- Kilbourne ED, Couch RB, Kasel JA, et al. Purified influenza a virus N2 neuraminidase vaccine is immunogenic and non-toxic in humans. Vaccine. 1995;13(18):1799–1803. doi: 10.1016/0264-410X(95)00127-M
- Johansson BE, Price PM, Kilbourne ED. Immunogenicity of influenza a virus N2 neuraminidase produced in insect larvae by baculovirus recombinants. Vaccine. 1995 Jun;13(9):841–845. doi: 10.1016/0264-410X(94)00071-T
- Hocart M, Grajower B, Donabedian A, et al. Preparation and characterization of a purified influenza virus neuraminidase vaccine. Vaccine. 1995 Dec;13(18):1793–1798.
- Deroo T, Jou WM, Fiers W. Recombinant neuraminidase vaccine protects against lethal influenza. Vaccine. 1996 Apr;14(6):561–569. doi: 10.1016/0264-410X(95)00157-V
- Skarlupka AL, Zhang X, Blas-Machado U, et al. Multi-influenza ha subtype protection of ferrets vaccinated with an N1 COBRA-based neuraminidase. Viruses. 2023 Jan 9;15(1):184. doi: 10.3390/v15010184
- Skarlupka AL, Bebin-Blackwell AG, Sumner SF, et al. Universal influenza virus neuraminidase vaccine elicits protective immune responses against human seasonal and pre-pandemic strains. J Virol. 2021 Aug 10;95(17):e0075921. doi: 10.1128/JVI.00759-21
- Kawai A, Yamamoto Y, Nogimori T, et al. The potential of neuraminidase as an antigen for nasal vaccines to increase cross-protection against influenza viruses. J Virol. 2021 Sep 27;95(20):e0118021. doi: 10.1128/JVI.01180-21
- Strohmeier S, Carreno JM, Brito RN, et al. Introduction of cysteines in the stalk domain of recombinant influenza virus N1 neuraminidase enhances protein stability and immunogenicity in mice. Vaccines (Basel). 2021 Apr 19;9(4):404. doi: 10.3390/vaccines9040404
- Gao J, Klenow L, Parsons L, et al. Design of the recombinant influenza Neuraminidase Antigen Is Crucial for Its Biochemical Properties and Protective Efficacy. J Virol. 2021 Nov 23;95(24):e0116021. doi: 10.1128/JVI.01160-21
- Strohmeier S, Amanat F, Zhu X, et al. A novel recombinant influenza virus neuraminidase vaccine candidate stabilized by a measles virus phosphoprotein tetramerization domain provides robust protection from virus challenge in the mouse model. MBio. 2021 Dec 21;12(6):e0224121. doi: 10.1128/mBio.02241-21
- Zheng A, Sun W, Xiong X, et al. Enhancing neuraminidase immunogenicity of influenza a viruses by rewiring RNA packaging signals. J Virol. 2020 Jul 30;94(16). doi: 10.1128/JVI.00742-20
- McMahon M, Strohmeier S, Rajendran M, et al. Correctly folded - but not necessarily functional - influenza virus neuraminidase is required to induce protective antibody responses in mice. Vaccine. 2020 Oct 21;38(45):7129–7137. doi: 10.1016/j.vaccine.2020.08.067
- Johansson B, Kilbourne E. Dissociation of influenza virus hemagglutinin and neuraminidase eliminates their intravirionic antigenic competition. J Virol. 1993;67(10):5721–5723. doi: 10.1128/jvi.67.10.5721-5723.1993
- Zhang F, Chen J, Fang F, et al. Maternal immunization with both hemagglutinin- and neuraminidase-expressing DNAs provides an enhanced protection against a lethal influenza virus challenge in infant and adult mice. DNA Cell Biol. 2005 Nov;24(11):758–765.
- Meseda CA, Atukorale V, Soto J, et al. Immunogenicity and protection against influenza H7N3 in mice by modified vaccinia virus ankara vectors expressing influenza virus hemagglutinin or neuraminidase. Sci Rep. 2018 Mar 29;8(1):5364. doi: 10.1038/s41598-018-23712-9
- Walz L, Kays SK, Zimmer G, et al. Neuraminidase-inhibiting antibody titers correlate with protection from heterologous influenza virus strains of the same neuraminidase subtype. J Virol. 2018 Sep 1;92(17). doi: 10.1128/JVI.01006-18
- Kang HJ, Chu KB, Yoon KW, et al. Neuraminidase in virus-like particles contributes to the protection against high dose of avian influenza virus challenge infection. Pathogens. 2021 Oct 7;10(10):1291. doi: 10.3390/pathogens10101291
- Menne Z, Pliasas VC, Compans RW, et al. Bivalent vaccination with NA1 and NA2 neuraminidase virus-like particles is protective against challenge with H1N1 and H3N2 influenza a viruses in a murine model. Virology. 2021 Oct;562:197–208. doi: 10.1016/j.virol.2021.08.001
- Li Q, Sun X, Li Z, et al. Structural and functional characterization of neuraminidase-like molecule N10 derived from bat influenza a virus. Proc Natl Acad Sci USA. 2012 Nov 13;109(46):18897–18902. doi: 10.1073/pnas.1211037109
- Krammer F, Fouchier RAM, Eichelberger MC, et al. Naction! How can neuraminidase-based immunity contribute to better influenza virus vaccines? mBio. MBio. 2018 Apr 3;9(2). doi: 10.1128/mBio.02332-17
- Zhuang Q, Wang S, Liu S, et al. Diversity and distribution of type a influenza viruses: an updated panorama analysis based on protein sequences. Virol J. 2019 Jun 26;16(1):85. doi: 10.1186/s12985-019-1188-7
- Shi W, Lei F, Zhu C, et al. A complete analysis of HA and NA genes of influenza a viruses. PLOS ONE. 2010 Dec 29;5(12):e14454. doi: 10.1371/journal.pone.0014454
- Kendal AP, Bozeman FM, Ennis FA. Further studies of the neuraminidase content of inactivated influenza vaccines and the neuraminidase antibody responses after vaccination of immunologically primed and unprimed populations. Infect Immun. 1980 Sep;29(3):966–971. doi: 10.1128/iai.29.3.966-971.1980
- Cate TR, Rayford Y, Niño D, et al. A high dosage influenza vaccine induced significantly more neuraminidase antibody than standard vaccine among elderly subjects. Vaccine. 2010;28(9):2076–2079. doi: 10.1016/j.vaccine.2009.12.041
- Couch RB, Atmar RL, Keitel WA, et al. Randomized comparative study of the serum antihemagglutinin and antineuraminidase antibody responses to six licensed trivalent influenza vaccines. Vaccine. 2012 Dec 17;31(1):190–195. doi: 10.1016/j.vaccine.2012.10.065
- Sergeeva MV, Romanovskaya-Romanko EA, Krivitskaya VZ, et al. Longitudinal analysis of neuraminidase and hemagglutinin antibodies to influenza a viruses after immunization with seasonal inactivated influenza vaccines. Vaccines (Basel). 2023 Nov 20;11(11):1731. doi: 10.3390/vaccines11111731
- Desheva Y, Smolonogina T, Donina S, et al. Study of Neuraminidase-inhibiting antibodies in clinical trials of live influenza vaccines. Antibodies (Basel). 2020 May 29;9(2):20. doi: 10.3390/antib9020020
- Wohlbold TJ, Nachbagauer R, Xu H, et al. Vaccination with adjuvanted recombinant neuraminidase induces broad heterologous, but not heterosubtypic, cross-protection against influenza virus infection in mice. MBio. 2015 Mar 10;6(2):e02556. doi: 10.1128/mBio.02556-14
- Wong SS, Waite B, Ralston J, et al. Hemagglutinin and neuraminidase antibodies are induced in an age- and subtype-dependent manner after influenza virus infection. J Virol. 2020 Mar 17;94(7). doi: 10.1128/JVI.01385-19
- Gostic KM, Ambrose M, Worobey M, et al. Potent protection against H5N1 and H7N9 influenza via childhood hemagglutinin imprinting. Science. 2016 Nov 11;354(6313):722–726. doi: 10.1126/science.aag1322
- Hansen L, Zhou F, Amdam H, et al. Repeated influenza vaccination boosts and maintains H1N1pdm09 neuraminidase antibody titers. Front Immunol. 2021;12:748264. doi: 10.3389/fimmu.2021.748264
- Kirchenbaum GA, Carter DM, Ross TM, et al. Sequential infection in ferrets with antigenically distinct seasonal H1N1 influenza viruses boosts hemagglutinin stalk-specific antibodies. J Virol. 2016 Jan 15;90(2):1116–1128.
- Kilbourne ED. Comparative efficacy of neuraminidase-specific and conventional influenza virus vaccines in induction of antibody to neuraminidase in humans. J Infect Dis. 1976 Oct;134(4):384–394. doi: 10.1093/infdis/134.4.384
- Kendal AP, Noble GR, Dowdle WR. Neuraminidase content of influenza vaccines and neuraminidase antibody responses after vaccination of immunologically primed and unprimed populations. J Infect Dis. 1977 Dec;136(Suppl: Supplement 3):S415–S424. doi: 10.1093/infdis/136.Supplement_3.S415
- Johansson BE, Moran TM, Kilbourne ED. Antigen-presenting B cells and helper T cells cooperatively mediate intravirionic antigenic competition between influenza a virus surface glycoproteins. Proc Natl Acad Sci USA. 1987 Oct;84(19):6869–6873. doi: 10.1073/pnas.84.19.6869
- Creytens S, Pascha MN, Ballegeer M, et al. Influenza neuraminidase characteristics and potential as a vaccine target. Front Immunol. 2021;12:786617. doi: 10.3389/fimmu.2021.786617
- Rajendran M, Krammer F, McMahon M. The human antibody response to the influenza virus neuraminidase following infection or vaccination. Vaccines (Basel). 2021 Aug 2;9(8):846.
- Wan H, Yang H, Shore DA, et al. Structural characterization of a protective epitope spanning A(H1N1)pdm09 influenza virus neuraminidase monomers. Nat Commun. 2015 Feb 10;6(1):6114. doi: 10.1038/ncomms7114
- Job ER, Schotsaert M, Ibanez LI, et al. Antibodies directed toward neuraminidase N1 control disease in a mouse model of influenza. J Virol. 2018 Feb 15;92(4). doi: 10.1128/JVI.01584-17
- Yasuhara A, Yamayoshi S, Kiso M, et al. A broadly protective human monoclonal antibody targeting the sialidase activity of influenza a and B virus neuraminidases. Nat Commun. 2022 Nov 3;13(1):6602. doi: 10.1038/s41467-022-34521-0
- Jiang H, Peng W, Qi J, et al. Structure-based modification of an anti-neuraminidase Human Antibody Restores Protection Efficacy against the drifted influenza virus. MBio. 2020 Oct 6;11(5). doi: 10.1128/mBio.02315-20
- Momont C, Dang HV, Zatta F, et al. A pan-influenza antibody inhibiting neuraminidase via receptor mimicry. Nature. 2023 Jun;618(7965):590–597.
- Lei R, Kim W, Lv H, et al. Leveraging vaccination-induced protective antibodies to define conserved epitopes on influenza N2 neuraminidase. Immunity. 2023 Nov 14;56(11):2621–2634 e6. doi: 10.1016/j.immuni.2023.10.005
- Kirkpatrick Roubidoux E, M M, Carreno JM, et al. Identification and characterization of novel antibody epitopes on the N2 neuraminidase. mSphere. 2021 Feb 10;6(1). doi: 10.1128/mSphere.00958-20
- Wang F, Wu J, Wang Y, et al. Identification of key residues involved in the neuraminidase antigenic variation of H9N2 influenza virus. Emerg Microbes Infect. 2021 Dec;10(1):210–219.
- Lu X, Liu F, Zeng H, et al. Evaluation of the antigenic relatedness and cross-protective immunity of the neuraminidase between human influenza a (H1N1) virus and highly pathogenic avian influenza a (H5N1) virus. Virology. 2014 Apr;454-455:169–175. doi: 10.1016/j.virol.2014.02.011
- Jiang L, Changsom D, Lerdsamran H, et al. Immunobiological properties of influenza A (H7N9) hemagglutinin and neuraminidase proteins. Arch Virol. 2016 Oct;161(10):2693–2704.
- Piepenbrink MS, Nogales A, Basu M, et al. Broad and protective influenza B virus neuraminidase antibodies in humans after vaccination and their clonal persistence as plasma cells. MBio. 2019 Mar 12;10(2). doi: 10.1128/mBio.00066-19