Abstract
Through cysteine-scanning mutagenesis, the authors have compared sites within the transmembrane domains of two connexins, one from the α-class (Cx50) and one from the β-class (Cx32), where amino acid substitution disrupts the function of gap junction channels. In Cx32, 11 sites resulted in no channel function, or an aberrant voltage gating phenotype referred to as “reverse gating,” whereas in Cx50, 7 such sites were identified. In both connexins, the sites lie along specific faces of transmembrane helices, suggesting that these may be sites of transmembrane domain interactions. In Cx32, one broad face of the M1 transmembrane domain and a narrower, polar face of M3 were identified, including one site that was shown to come into close apposition with M4 in the closed state. In Cx50, the same face of M3 was identified, but sensitive sites in M1 differed from Cx32. Many fewer sites in M1 disrupted channel function in Cx50, and those that did were on a different helical face to the sensitive sites in Cx32. A more in depth study of two sites in M1 and M2 of Cx32 showed that side-chain length or branching are important for maintenance of normal channel behavior, consistent with this being a site of transmembrane domain interaction.
INTRODUCTION
Gap junctions mediate direct intercellular communication between animal cells. The connexin protein family constitutes gap junction channels in mammalian tissues, and at least 20 different connexin genes have been identified. The membrane topology of connexin proteins has been well characterized (Milks et al. Citation1988). The amino and carboxyl termini are cytoplasmically located and each connexin traverses the plasma membrane four times, with the membrane-spanning segments referred to as M1 to M4. Six connexins oligomerize to form a connexon, or hemichannel, in one cell. A similar complex forms in an adjacent cell, and the two halves “dock” in the extracellular space to establish the intercellular channel of a gap junction (Bruzzone et al. Citation1996; Foote et al. Citation1998).
In hemichannels, residues in M1 and the first extracellular loop (Kronengold et al. Citation2003), as well as M3 (Zhou et al. 1999), have been identified as lining the channel. However, once an intercellular channel forms, the pore of the channel is lined by amino acids from the second and third transmembrane domains (Skerrett et al. Citation2002), as well as parts of the extracellular loops and possibly cytoplasmic domains that have not yet been identified. Identification of the pore-lining residues in these studies was achieved through the substituted cysteine accessibility method (SCAM), where cysteines are substituted one at a time at multiple positions in the transmembrane domains, and tested for channel block by sulfhydryl reagents.
Although published two-dimensional crystal structures of gap junction channels confirm the dodecameric nature of the connexin protein complex, and an alpha-helical secondary structure for the membrane-spanning domains, neither provides the resolution required to identify sites of helical interaction or to unequivocally assign helical densities to individual transmembrane domains (Cx43, Unger et al. Citation1999; Cx26, Oshima et al. Citation2007). However, several models of transmembrane domain architecture have assigned the domains visualized in the crystal structure of Cx43 to specific transmembrane helices, M1 to M4. Skerrett et al. (Citation2002) tentatively assigned the transmembrane domains based on SCAM. A more recent model published by Fleishman et al. (Citation2004) predicts the location of α-carbons for amino acids within all four transmembrane helices of a generic connexin, and assigns specific helices based on criteria that include patterns of evolutionary conservation of residues, distribution of disease-related mutations, and effects of site directed mutagenesis. These two models agree on the assignment of M3 (the main pore-lining helix) and M4, but reverse the assignments of M1 and M2. The recently published crystal structure of Cx26 provides support for the assignments proposed in Skerrett et al. (Citation2002), based on the location of an electron dense cytoplasmic structure that is likely to be the cytoplasmic loop connecting M2 and M3 (Oshima et al. Citation2007).
Interactions between transmembrane domains are likely to play important roles in channel stabilization, conformational changes associated with voltage gating, and subunit interactions during connexin oligomerization. Thus, sites of close interactions between helices, either within a subunit or between them, would be highly sensitive to mutagenesis, resulting in nonfunctional channels due to failure in assembly or channels with aberrant properties (Minor et al. Citation1999). In gap junctions, this may particularly affect voltage gating, which has been proposed to involve propagated conformational changes among transmembrane helices. This is, indeed, the premise upon which the Fleishman et al. (Citation2004, Citation2006) models were generated, relying predominantly on the distribution of disease associated mutations in Cx32 applied to a Cα model based on Cx43 structure. This model depicts helical faces that interact with nearby helices and suggests sites of pairwise interaction. A pairwise interaction between R32 (in M1) and E146 (in M3) was supported experimentally, through a charge swap experiment. A proposed interaction between K22 (in M1) and E209 (in M4) was also consistent with other mutation studies. However, in dealing with disease mutants, multiple amino acid properties are changed, possibly altering function by way of what was introduced, rather than what was taken out. Systematic mutation to a relatively neutral amino acid like alanine (Braun et al. Citation1997) or cysteine, as done in the SCAM studies, is a more interpretable approach, particularly if the overall distribution of sensitive sites on the helices are considered as an indicator of transmembrane domain interactions. Thus, the distribution of cysteine substitutions in the SCAM analysis of Skerrett et al. (Citation2002) and Toloue (Citation2007) that led to nonfunctional gap junctions or channels with aberrant properties may provide the strongest indication yet as to the likely sites of interhelical interaction within the connexin. In one specialized example, the demonstration of disulfide formation between E146C (in M3) and endogenous C201 (in M4) in Cx32 that locked the channel in a closed state until reduced provided the most direct evidence for a close interaction between these helices (Skerrett et al. Citation2002). We present here a comprehensive assessment of the functional effects of substitution at over 80 sites in Cx32 and Cx50. This provides a mapping of helical interfaces that will help in developing a higher resolution structure of gap junction channels, and pave the way for future studies of channel stability, conformational changes that are associated with gating, and determinants of subunit assembly including heteromeric connexin interactions.
MATERIALS AND METHODS
Preparation of Cysteine Mutants and cRNA
RatCx32 (Paul 1986) was cloned into the EcoR1 site of PGem7zf (+) (Promega, Madison, WI). MouseCx50 (White et al. Citation1992) was cloned into pSP64T and linearized with BamHI for the in vitro transcription of mRNA with SP6 RNA polymerase. For both constructs, mutants were made by site-directed mutagenesis using overlap extension by polymerase chain reaction as described by Ho et al. (1989) with PWO DNA polymerase (Boehringer Mannheim) in a PTC-100 Programmable Thermal Controller (MJ Research). Typical amplification of DNA fragments was achieved by adding 100 to 200 ng of template DNA, 1 mM Tris-HCl (pH 8.85), 5 mM KCl, 1 mM (NH4)2SO4, 0.4 mM MgSO4, 200 μ M of each dNTP, 1 μ M of each primer, and 0.5 units of DNA polymerase in a final volume of 50 μ l. Primer length was 19 to 21 for universal primers and 21 to 24 for mutant primers. Samples were subjected to 30 cycles of PCR, with annealing temperatures dictated by mutant primer length and composition. Sequencing of clones was performed using the fmol DNA Cycle Sequencing System (Promega, Madison, WI) with [γ-32P]ATP from NEN (Perkin Elmer/NEN, Norwalk, CT) and separation of oligonucleotides on 6% Long Ranger gels (FMC Bioproducts, Rockland, ME) or by the CAMBI Nucleic Acid Facility (SUNY, Buffalo). Each mutant was sequenced across the entire coding region. cRNA was prepared using mMessage mMachine RNA kits (Ambion, Austin, TX) or Ampliscribe TM SP6 High Yield Transcription kits (Epicentre Technologies, Madison, WI).
Expression and Recording from Oocytes
The technique of recording intercellular currents from paired Xenopus oocytes was carried out as described by Skerrett et al. (Citation2000). Oocytes were removed from ovulating, Xenopus laevis females and the follicular layer partially removed with collagenase. The remaining follicular cells were removed using fine forceps before oocytes were coinjected with 4 to 20 ng of connexin RNA mixed with 4 ng of antisense oligonucleotide directed against nucleotides 327 to 353 of the endogenous Xenopus Cx38 (Barrio et al. 1991). Approximately 24 h after injection, oocytes were stripped of their vitelline membrane and paired overnight either in agar wells, or in specialized perfusion chambers. Oocytes were continuously bathed in half-strength L15 medium, which included 89 mM KCl and 0.6 mM CaCl2 (Sigma, St. Louis, MO) or in Oocyte Ringers 2 (OR2; 82.5 mM NaCl, 2 mM KCl, 1 mM MgCl2, 5 mM HEPES, pH 7.4)
To assess junctional conductance, oocytes were clamped between −20 and −40 mV using two Geneclamp Amplifiers (Molecular Devices, Sunnyvale, CA). One cell was then pulsed to more positive and negative potentials in order to elicit a junctional current in the clamped cell. Currents were measured within the first 100 ms of the voltage pulse (instantaneous current) for normal gating channels, or at the end of a 4-s pulse (steady-state current) for mutants with a Vj-activated phenotype. A more detailed characterization of Vj sensitivity was obtained by recording current in response to longer voltage steps (10 to 20 s) of 10-or 20-mV increments to maximum Vj's of +/−100 mV. Data were acquired and analyzed using PClamp software (versions 8, 9, or 10; Molecular Devices, Union City, CA, USA).
RESULTS AND DISCUSSION
Original studies aimed at identifying pore-lining residues in gap junction channels led to the creation of 50 cysteine-substitutions mutants within the transmembrane domains of Cx32 (Skerrett et al. 2002) and 38 cysteine-substitution mutants within the transmembrane domains of Cx50 (Toloue Citation2007). Functional characterization of each mutant in paired Xenopus oocytes revealed that many of these substitutions disrupted gap junction channel function. In some cases the disruption in function could be attributed to removal of a property that was unique to the native amino acid, such as removal of the conserved proline in M2. However, the overall pattern revealed specific faces of the transmembrane helices that were sensitive to substitution. In Cx32, 14 nonfunctional mutants defined mutation sensitive faces on all four transmembrane helices. In Cx50, five nonfunctional cysteine-substitution mutants were identified in three transmembrane helices tested. Three of these were located on the same non–pore-lining polar face of M3 as the residues identified in Cx32. The overall distribution of sensitive sites within the transmembrane domains of Cx32 and Cx50 is shown in and further details regarding the location and properties of these sites are provided below.
Figure 1 Alignment of Cx50 and Cx32 protein sequences outlining the four transmembrane domains, M1, M2, M3, and M4 with a dashed box. Letters in red and blue text are sites that where cysteine substitutions were created for pore-lining studies of Cx32 and Cx50 respectively. Helical net plots are shown below for transmembrane domains of each connexin. Non-functional sites are highlighted with red circles and blue circles (Cx32 and Cx50, respectively) and indicate sites where cysteine substitution rendered the channels nonfunctional during scanning cysteine mutagenesis. Pore-lining residues in Cx32 are highlighted in light blue (Skerrett et al. Citation2002). Sequences were aligned using the ALIGN Optimal Global Alignment function (Biology Workbench, San Diego Super Computer Center).
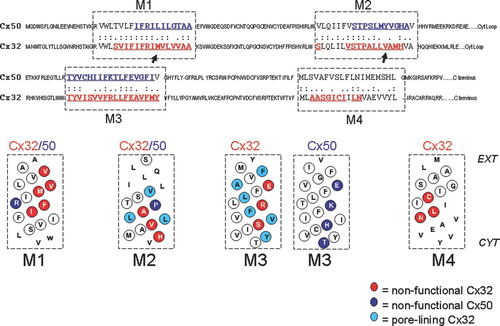
The First Transmembrane Domain
Five substitution-sensitive sites were identified within the first transmembrane domain of Cx32, occupying abroad helical surface (). Cysteine substitution at all these sites induced a phenotype characterized by a lack of coupling in homotypic mutant pairings, whereas in heterotypic pairings with wtCx32, the mutant channel could be induced to open with the application of positive transjunctional voltage (Vj). This gating phenotype is termed “reverse-gating” because the channels show a reversed response to Vj, opening rather than closing. A typical reverse-gating phenotype is shown in , although it is important to note that the voltage dependence and time dependence of these currents vary slightly between mutants. This phenotype was first reported after removal of the conserved proline in M2 of Cx26 (Suchyna et al. Citation1993) and later for the CMTX mutants M34T, V35M, and V38M in the first transmembrane domain of Cx32 (Oh et al. Citation1997). In all cases, an accompanying shift in the conductance versus voltage relationship suggests that the closed state (or a residual low conductance state) is favored in the absence of an applied transjunctional field, a condition that favors the open state of wt channels (Suchyna et al. Citation1993; Oh et al. Citation1997; Skerrett et al. Citation1999). Based on these observations, it appears as though the first transmembrane domain plays a critical role in the conformational changes that accompany Vj-dependent gating. Interactions between M1 and other transmembrane helices are likely to underlie such transduction mechanisms in Cx32, and changes in residues at such interfaces would be expected to modify gating behavior.
Figure 2 Junctional currents recorded from paired Xenopus oocytes after expression of Cx32 mutants demonstrate that side-chain properties are critical for channel function. Upper traces represent the “reserve-gating” phenotype observed when mutant channels are paired heterotypically with wtCx32. In both cases currents activate when the mutant-expressing oocyte experiences relatively positive transjunctional voltage. Lower traces represent amino substitutions at the same two sites, M34 and V91, which did not significantly alter channel function. At position M34, substitution of amino acids with side chains shorter than the native methionine induced the “reverse-gating” phenotype, whereas side chains that were comparable in length to those of methionine did not disrupt function. At position V91, only substitution of amino acid with β-branched side-chains produced functional channels. Currents were recorded from an oocyte clamped at −40 mV, whereas its partner was pulsed in 10 mV increments (left hand traces) or 20-mV increments (right hand traces) to +60 and −140 mV.
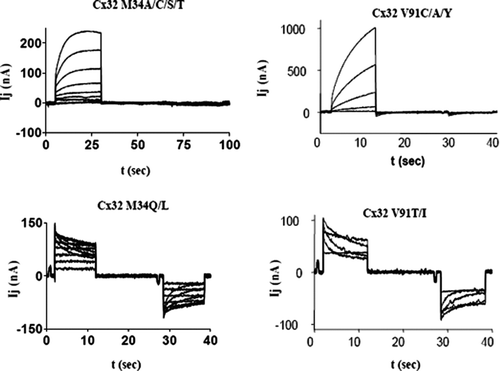
In contrast to the broad distribution of sensitive sites identified in M1 of Cx32, only one residue in the first transmembrane domain of Cx50 was sensitive to cysteine substitution, suggesting that close helical interactions of M1 with other helices is less critical for function in Cx50 than Cx32. Furthermore, rather than showing reverse gating, Cx50 R33C failed to form functional channels homotypically, or in heterotypic pairings with wild type. This residue is located on the face of the helix that displayed little sensitivity to cysteine substitution in Cx32 (), suggesting that M1 could play very different functions in these two connexins. Also, because the charged side chain of arginine is replaced by the shorter, polar side chain of cysteine, this is a case where either the property or location of the residue may be critical for channel function. This question was addressed by Fleishman et al. (Citation2006). The proper localization of green fluorescent protein (GFP)-tagged Cx32 within a cell depended on an interaction between R32 and E146. Removing the charge at either site disrupted channel assembly, while swapping the charges at these sites (arginine to glutamate and vice versa), rescued the assembly of channels. Fleishman et al (Citation2006) concluded that these residues form a salt bridge that is critical for gap junction biosythesis. Although this hypothesis is consistent with our observation that both R33C and E169C are nonfunctional in Cx50 (Toloue Citation2007), it contradicts our results with Cx32. In Cx32, functional channels resulted from the R32C substitution whereas E146C formed a disulfide bond with endogenous C201 in M4 that stabilized the channel in the closed state. The discrepancy in studies of Cx32 may be related to the fact that Fleishman et al. (Citation2006) studied assembly of gap junction plaques rather than the functional status of mutant channels. It is also possible that a given site, like E146 in M3, could interact with different helices in open and closed states of the pore, during intermediate folding states of the protein, or at different times during channel assembly.
The Second Transmembrane Domain
Three sites, which delineated a diagonal face of the second transmembrane helix of Cx32 adjacent to that mapped to line the pore in Skerrett et al. (Citation2002), were sensitive to cysteine substitutions at A88, V91, and H94 (). Cysteine substitution at position 88 induced greatly increased hemichannel activity in Cx32, as oocytes injected with Cx32A88C showed an increase in membrane conductance associated with death of the oocytes, unless the extracellular solution was supplemented with 2 mM CaCl2 (Skerrett et al. Citation2002). In contrast, cysteine substitution at V91 induced a reverse-gating phenotype similar to that described for M1 substitution mutants in Cx32 (). The H94C substitution failed to produce functional channels, as previously reported for Cx43 (Ek et al. Citation1994), where a range of substitutions suggested that a properly positioned histidine was required for functional expression of channels.
In Cx50, only removal of the conserved proline disrupted channel function, and neither homotypic nor heterotypic pairings with wtCx50 induced intercellular currents in oocytes. It was initially suggested that the conserved proline in M2 of connexins was essential for voltage gating, as removal of this residue in Cx26 induced a “reverse-gating” phenotype (Suchyna et al. Citation1993). Since that time, the importance of this proline in gating has been correlated with positive-gating connexins, meaning connexins that gate closed when their hemichannel or connexon is located on the positive side of a transjunctional voltage gradient. The observation that this proline in Cx50 is essential for channel function is consistent with its positive-gating polarity. Removal of this proline from negative-gating connexins (e.g., Cx32) does not disrupt the normal gating mechanism of the channel (Ri et al. Citation1999; J. Smith, personal communication), although mutation of the adjacent site, T97, in Cx32 did affect gating, consistent with a role for proline in defining a specific bend angle in the M2 helix (Ri et al. Citation1999). A lack of sensitivity on the corresponding face of the M2 helix in the cysteine-scanning analysis of Cx50 suggests that either the unique properties of proline are required for channel function or that this residue represents a localized site of transmembrane domain interaction. This is in contrast to the results of cysteine substitution in M2 of Cx32 where sensitive sites are located on one helical face (not the one containing the proline) over three rotations of the helix. These differences provide strong evidence that the interhelical interactions and role of the second transmembrane domain in gating differ between Cx32 and Cx50, and possibly in general between positive-and negative-gating channels.
The Third Transmembrane Domain
Three sites within the third transmembrane domain of Cx32, S138, R142, and E146, were sensitive to cysteine substitution. Cysteine substitution at S138 and R142 produced channels that functioned only when paired heterotypically with wild-type (wt) Cx32 and produced the “reverse-gating” phenotype. Cysteine substitution at E146 produced channels that were locked in a closed state prior to perfusion of reducing agent (Skerrett et al. Citation2002), a similar effect to that observed after cysteine substitution at E146 in chimeric hemichannels (Zhou et al. Citation1997). Because removing the cysteine in M4 (Cx32E146C/C201S) restores channel function (Skerrett et al. Citation2002), it is likely that E146 faces M4 in close enough apposition in the closed state of the channel to form a disulfide. How this may correlate with the proposed interaction between E146 and R32 (Fleishman et al. Citation2006) is discussed above and may indicate that M1 undergoes rotation in different states or stages of channel assembly.
The third transmembrane domain is the main pore-lining helix in both Cx32 and Cx50. In Cx32, pore-lining residues include Y135, V139, L141, L144, F149, and Y151 (Skerrett et al. Citation2002) and these residues are highlighted with blue circles on the helical net plot in . Cysteine mutants that lead to aberrant function, as described above, are aligned along the non–pore-lining, polar face of the M3 helix, and are highlighted in red in and .
Figure 3 Tentative arrangement of transmembrane helices based on the location of mutation-sensitive sites within the helices. Helical wheel plots are used to show the relative location of substitution-sensitive sites in Cx32 (highlighted with red circles), Cx50 (highlighted with blue circles), as well as substitution-sensitive sites common to both (highlighted with red/blue shading). The two sites in M1 highlighted in red/yellow ovals were shown to be accessible to aqueous thiol reagents added from the outside of intact oocytes, and are thus likely to face a aqueous crevice between helices M1 and M4. For simplicity amino acid numbers correspond to the amino acid positions in Cx32 (see for corresponding Cx50 residues). Helices are arranged as proposed earlier by Skerrett et al. (Citation2002), including the close apposition documented for E146 and C201 in the closed state. This arrangement of helices is in general agreement with the recently published crystal structure of Cx26 (Oshima et al. Citation2007). Protein function was unaffected by tryptophan insertion at positions highlighted in green, suggesting that these residues face a lipid or aqueous environment.
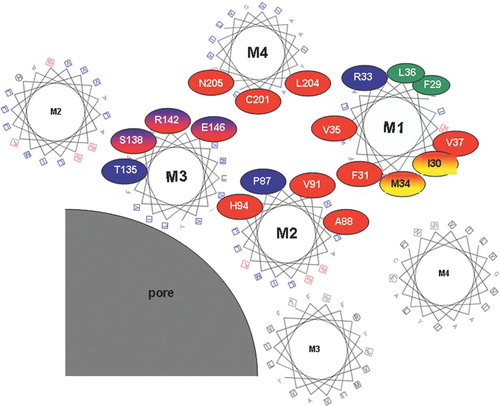
In Cx50, four nonfunctional cysteine-substitution mutants (T157, H161, K165, and E169) were identified in the third transmembrane domain (highlighted in red in and in blue in ). Three of these four sites correspond to those identified in the Cx32 cysteine-scanning study, and all four define the same polar face of the M3 helix, representing a diagonal stripe orthogonal to the pore lining face, which is generally conserved between Cx50 and Cx32 (unpublished observations). It appears as if the two faces cross, indicating that, if these sites reflect interacting surfaces with other helices, they must involve two helices on either side of the pore surface.
Three of the nonfunctional mutants in Cx50, T157C, H161C, and E169C, failed to function homotypically or in heterotypic pairings with wt Cx50. The threonine at position 157 is highly conserved and it has been shown previously that this residue is essential for proper function of all connexins tested, including Cx26, Cx43, and Cx50 (Beahm et al. Citation2006). This site was not tested in the Cx32 study. K165C produced a “reverse-gating” phenotype similar to the “reverse-gating” phenotype observed for M34 and V91 in Cx32 (), This was the only “reverse-gating” mutant identified during functional tests of cysteine mutants in Cx50, and suggests that, although there may be differences in the voltage gating mechanisms of Cx50 and Cx32 with respect to M1, there may be common aspects to this mechanism with regard to M3.
Sites within the Fourth Transmembrane Domain
Two sites within the fourth transmembrane domain of Cx32 were sensitive to cysteine substitution and these were L204 and N205. These are highlighted in red on the alignment in . In addition, C201 is highlighted because this residue appears to form a disulfide with a substituted cysteine at position E146 in M3 that stabilizes the closed state (as described above). Both of the cysteine substitution mutants, L204 and N205, induced a typical “reverse-gating” phenotype, activating in response to application of positive Vj when paired heterotypically with wtCx32. The N205C reverse-gating channels could be activated with relatively small Vj in the order of 20 mV, and subsequently recovered very slowly, requiring many minutes for the channel to return to its original closed state (data not shown).
The three residues C201, L204, and N205 lie along the same broad face of the M4 helix and cover two rotations of the helix, although it is important to note that more cytoplasmic residues were not involved in the cysteine scanning study. Evidence for disulfide formation between C201 and E146 suggest that M4 interacts closely with the polar face of the M3 helix. It is thus reasonable to speculate that L204 and N205 interact with this same face of M3. Unfortunately, no comparison of these sites was performed in Cx50. However, based on the similarity of mutation sensitive sites in M3 of Cx32 and Cx50, one might predict that orthologous sites would be sensitive to cysteine substitution.
Expansion of the Investigation Beyond Cysteine
The tendency for cysteine substitution-sensitive sites to lie along specific faces of all transmembrane domains in Cx32, and in M3 of Cx50, suggests that these sites may be regions of protein interaction within the membrane. In order to further test this hypothesis, a series of amino acid substitutions were made at two sites in Cx32 (M34 and V91). In both cases, these substitutions revealed that specific side-chain properties are essential for channel function. At position M34 in the first transmembrane domain, side-chain length was a critical determinant of channel function. Substitition of residues with side chains shorter than that of the native methionine (Ala, Ser, Thr, and Cys) resulted in channels with a “reverse-gating” phenotype, whereas substitution of residues with side-chain lengths similar to that of methionine (Leu, Gln) resulted in channels with a normal phenotype. These data are summarized in . At position V91 in the second transmembrane domain, side-chain branching was a critical determinant of channel function. Substitution of residues that lacked β-carbon branching (Ala, Tyr, Cys) resulted in channels with the ‘reverse-gating” phenotype, whereas β-branched side chains (Thr, Ile) resulted in channels with a normal phenotype. These results are consistent with the involvement of M34 and V91 in protein interactions within Cx32 channels that are sensitive to the size, shape or rotational flexibility (restrictions in β-branched residues) of the side chains. It would then logically follow that other mutation sensitive sites on the same face of the helix define a surface of interaction between two helices, either intra-or intersubunit in nature.
A common method of investigating transmembrane domain interactions in membrane proteins involves functional analysis of tryptophan substitution mutants. The large, bulky side chain of tryptophan is expected to be well tolerated when facing an aqueous or lipid environment but not when positioned at sites of protein interaction (Sharp et al. Citation1995). To further test the hypothesis that M34 is a site of transmembrane domain interaction in Cx32, tryptophan was substituted at this site (M34W) and gap junction channel behavior was tested in Xenopus oocytes. An additional site on the opposite face of the helix (F29W) was also tested. The results of tryptophan substitution are summarized in . Cx32M34W failed to form functional channels even in heterotypic pairings and thus represents a more severe disruption of channel function than any of the six substitutions tested in , all of which had maintained or reduced the size of the original mutated side chain. In contrast, Cx32F29W showed no significant changes in channel function. In addition, tryptophan substitution at another site on the same helical face (L36) also failed to disrupt channel function (green ovals in ). These results provide further support for the hypothesis that the M34 side of M1 represents a helix-helix interaction surface, whereas the F29 surface may face the lipid, consistent with the interpretation of other studies where tryptophan scanning was utilized to map transmembrane domain interactions (Subbiah et al. Citation2005).
Figure 4 Junctional conductance induced by tryptophan substitution in Cx32 shows no effect at position F29, but a complete loss of function at M34 on the other side of the M1 helix. Each mutant was tested heterotypically with wtCx32 and conductance was determined from the maximum current induced during a +/−100-mV voltage step. Numbers displayed above the bars represent the number of pairs tested. RNA was quantitated by gel electrophoresis and equal amounts of RNA were injected into stage VI oocytes for each mutant, as well as for wtCx32. Oocytes were preinjected with 3 ng of morpholino antisense directed against endogenous Xenopus Cx38 1 or 2 days prior to recording.
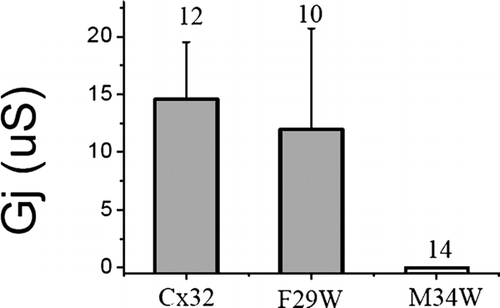
In summary, accumulated information regarding mutation-sensitive sites within the transmembrane domains of connexins has been used to map sites of transmembrane domain interaction in two different connexins. The relative location of mutation-sensitive sites within helices is shown in , providing a set of data that can be used in future models of gap junction structure and function. The model describes the arrangement of helices around the gap junction pore, consistent with data described above and also with pore-lining studies of Cx32 (Skerrett et al. Citation2002) and the crystal structure of Cx26 (Oshima et al. Citation2007). By using helix wheel representations, the tilts of the helices with respect to the membrane identified in the Unger (Citation1999) and Oshima (Citation2007) models are not reflected, which can affect the alignment of residues. Nonetheless, this representation serves to demonstrate that the mutation sensitive sites can be clustered at regions of helix-helix contact, predominantly within a subunit, but also between subunits. Although these sensitive sites are similarly distributed in M3 between Cx32 and Cx50, significant differences in the extent and location of sensitive sites between these connexins in M1 and M2 indicate that the packing of helices may differ significantly between connexins.
Two sites in M1 of Cx32, M34 and I30, which do not in this representation make close contact with another helix, were shown to be accessible to extracellular application of a maleimide based thiol reagent (Skerrett et al. Citation2002). This is consistent with an aqueous crevice that likely exists between M1 and M4 of adjacent subunits. Of the four sites shown to be exposed to the pore in M3, and three in M2, three and one, respectively, have been mapped as pore lining in the previous SCAM studies (Skerrett et al. Citation2002). It should be noted that rotations of the helices with respect to one another may also occur during assembly or gating. Such rotations may bring sites like V37, I30 and M34 into closer apposition to other helices, explaining why mutations at these sites affect gating dynamics, as well as proffering a an explanation as to how R33-E146 interactions proposed by Fleishman et al. (Citation2006) could occur, whereas E146-C201 proximity is observed in the closed state (Skerrett et al. Citation2002). This study should pave the way for future studies of transmembrane domain interactions and their roles in channel stability, conformational changes that are associated with gating, and subunit assembly.
REFERENCES
- Beahm D L, Oshima A, Gaietta G M, Hand G M, Smock A E, Zucker S N, Toloue M M, Chandrasekhar A, Nicholson B J, Sosinsky G E. Mutation of a conserved threonine in the third transmembrane helix of alpha-and beta-connexins creates a dominant-negative closed gap junction channel. J Biol Chem 2006; 281: 7994–8009
- Braun P, Persson B, Kaback H R, von Heijne G. Alanine insertion scanning mutagenesis of lactose permeasetransmembrane helices. J Biol Chem 1997; 272: 29566–29571
- Bruzzone R, White T W, Paul D L. Connections with connexins: The molecular basis of direct intercellular signaling. Eur J Biochem 1996; 238: 1–27
- Ek J F, Delmar M, Perzova R, Taffet S M. Role of histidine 95 on pH gating of the cardiac gap junction protein connexin43. Circ Res 1994; 74: 1058–1064
- Fleishman S J, Sabag A D, Ophir E, Avraham K B, Ben-Tal N. The structural context of disease-causing mutations in gap junctions. J Biol Chem 2006; 39: 28958–28963
- Fleishman S J, Unger V M, Yeager M, Ben-Tal N. A Cα model for the transmembrane α helices of gap junction intercellular channels. Mol Cell 2004; 15: 878–888
- Foote C I, Zhou L, Zhu X, Nicholson B J. The pattern of disulfide linkages in the extracellular loop regions of connexin 32 suggests a model for the docking interface of gap junctions. J Cell Biol 1998; 140: 1187–97
- Kronengold J, Trexler B, Bukauskas F F, Bargiello T A, Verselis V K. Pore-lining residues identified by single channel SCAM studies in Cx46 hemichannels. Cell Commun Adhes 2003; 10: 193–199
- Milks L C, Kumar N M, Houghten R, Unwin N, Gilula N B. Topology of the 32-kd liver gap junction protein determined by site-directed antibody localizations. EMBO J 1988; 7: 2967–2975
- Minor D L, Jr, Masseling S J, Jan Y N, Jan L Y. Transmembrane structure of an inwardly rectifying potassium channel. Cell 1999; 96: 879–891
- Oh S, Ri Y, Bennett M V, Trexler E B, Verselis V K, Bargiello T A. Changes in permeability caused by connexin 32 mutations underlie X-linked Charcot-Marie-Tooth disease. Neuron 1997; 4: 927–38
- Oshima A, Tani K, Hiroaki Y, Fujiyoshi Y, Sosinsky G E. Three-dimensional structure of a human connexin26 gap junction channel reveals a plug in the vestibule. Proc Natl Acad Sci USA 2007; 104: 10034–10039
- Ri Y, Ballesteros J A, Abrams C K, Oh S, Verselis V K, Weinstein H, Bargiello T A. The role of a conserved proline residue in mediating conformational changes associated with voltage gating of Cx32 gap junctions. Biophys J 1999; 76: 2887–2898
- Sharp L L, Zhou J, Blair D F. Tryptophan-scanning mutagenesis of MotB, an integral membrane protein essential for flagellar rotation in Escherichia coli. Biochemistry 1995; 34: 9166–9171
- Skerrett I M, Smith J F, Nicholson B J. Mechanistic differences between chemical and electrical gating of gap junctions. Current Topics in Membranes, vol. 49, C Peracchia. Academic Press, San Diego, CA 1999; 249–269
- Skerrett I M, Merritt M, Zhou L, Zhu H, Cao F-L, Smith J F, Nicholson B J. Applying the Xenopus oocyte expression system to the analysis of gap junction proteins. Methods in Molecular Biology Connexin Methods and Protocols, R Bruzzone, C Giaume. Humana Press, NJ 2000; 225–249
- Skerrett I M, Aronowitz J, Shin J H, Cymes G, Kasperek E, Cao F-L, Nicholson B J. Identification of amino acid residues lining the pore of a gap junction channel. J Cell Biol 2002; 159: 349–359
- Subbiah R N, Kondo M, Campbell T J, Vandenberg J I. Tryptophan scanning mutagenesis of the HERG K+ channel: The S4 domain is loosely packed and likely to be lipid exposed. J Physiol 2005; 569: 367–379
- Suchyna T M, Xu L X, Gao F, Fourtner C R, Nicholson B J. Identification of a proline residue as a transduction element involved n voltage gating of gap junctions. Nature 1993; 365: 847–849
- Toloue. Selectivity of Gap Junctions for Natural Metabolites and its Structural Basis. PhD thesis, University of Buffalo, State University of New York, Buffalo, NY 2007
- Unger V M, Kumar N M, Gilula N B, Yeager M. Three-dimensional structure of a recombinant gap junction membrane channel. Science 1999; 283: 1176–1180
- White T W, Bruzzone R, Goodenough D A, Paul D L. Mouse Cx50, a functional member of the connexin family of gap junction proteins, is the lens fiber protein MP70. Mol Biol Cell 1992; 3: 711–720
- Zhou X W, Pfahnl A, Werner R, Hudder A, Llanes A, Luebke A, Dahl G. Identification of a pore lining segment in gap junction hemichannels. Biophys J 1997; 72: 1946–1953