Abstract
Exposure to environmental toxicants that affect the immune system and overall health of many mammals is mostly unavoidable. One of the more common substances is the mycotoxins, especially carcinogenic aflatoxin (AF)B1 which also causes immune suppression/dysregulation in exposed hosts. The present study analyzed the effects of naturally occurring levels of AFB1 on apoptosis of healthy bovine and camelid neonatal neutrophils (PMN) that were isolated both before and after host consumption of colostrum. Cells from bovine and camel neonates (n = 12 sets of PMN/mammal/timepoint) were exposed for 24 h to a low level of AFB1 (i.e. 10 ng AFB1/ml) and then intracellular ATP content and caspase-3, -7, and -9 activities (determined by bioluminescence) were assessed. The results indicated a significant lessening of intracellular ATP content and equivalents of luminescence intensity in AFB1-treated PMN in all studied samples, i.e. isolated pre-and post-colostrum consumption. In contrast, caspase-3, -7, and -9 activities in both pre- and post-colostrum consumption bovine and camelid PMN were noticeably increased (∼>2-fold). The damaging effects of AFB1 were more pronounced in bovine neonate PMN than in camelid ones. These results showed that camelid or bovine neonatal PMN collected pre- and post-colostrum are sensitive (moreso after consumption) to naturally occurring levels of AFB1. While merits of colostrum are well known, its failure to mitigate toxic effects of AFB1 in what would translate into a critical period in the development of immune competence (i.e. during the first few days of life in bovine and camelid calves) is surprising. The observed in vitro toxicities can help clarify underlying mechanisms of immune disorders caused by AFs in animals/humans.
Introduction
There are many environmental substances that can affect the functions of immune cells in mammals, (in)directly leading to increases in infectious and noninfectious diseases (Mehrzad et al. Citation2011, Citation2017; Viegas et al. Citation2013, Citation2015; Mehrzad, Devriendt, et al. Citation2015; Mehrzad, Shajari, et al. Citation2015; Taheri et al. Citation2016). One class of those is mycotoxins, especially highly broadly non-selective toxic and carcinogenic aflatoxin (AF)B1. Although advances in agrifood technology have greatly reduced the potential for human/live-stock AFB1 exposures (Cervino et al. Citation2008; Hernandez Hierro et al. Citation2008; Li et al. Citation2009), anthropogenic activities/climate change still impact on exposure risks worldwide (Fink-Gremmels Citation2008; Marroquín-Cardona et al. Citation2014; Mehrzad, Bahari, et al. Citation2018; Mehrzad, Hosseinkhani, et al. Citation2018), especially in developing nations (Mehrzad et al. Citation2011, Citation2013; Mozafari et al. Citation2017). Indeed, the current and future concerns about AFB1 exposure are not just focused on detectable levels in the food chain or the human body; a constant serious issue is chronic naturally occurring exposures to low- to very low levels of AFB1 (Fink-Gremmels Citation2008; Mehrzad et al. Citation2011, Citation2013; Jeannot et al. Citation2012; Viegas et al. Citation2013, Citation2015).
In developing nations (and even European/Nordic nations), animals/humans are routinely exposed to very low levels of AFB1 (Martins et al. Citation2007; Gallo et al. Citation2008; Hernandez Hierro et al. Citation2008; Viegas et al. Citation2013, Citation2015). This has been shown to result in damage to innate immune cells and their associated molecules (Mehrzad et al. Citation2013; Mehrzad, Devriendt, et al. Citation2014; Mehrzad, Maleki, et al. Citation2014) and increases in the incidence of a variety of chronic infectious/noninfectious diseases that impact on animal and human health. Thus, beyond its known carcinogenic potential, it has become increasingly clear this mycotoxin also presents an immunotoxic risk (Mehrzad et al. Citation2011; Jeannot et al. Citation2012; Mehrzad, Bahari, et al. Citation2018; Mehrzad, Hosseinkhani, et al. Citation2018). Accordingly, better analytical techniques designed for even more-precise detection of AFB1 in agri-products (and the bodies of/product from feed animals) are required to achieve successful strategies to mitigate overall AFB1 contamination from the start and its spread through the food chain and into humans. Nevertheless, even with the caveat that although AFB1 is an important environmental carcinogen/immunotoxin, toxifications of feed/food products do not occur in isolation. It is important to note that human consumption of feeds/animal meat-products contaminated with a combination of AFB1 and other potent toxicants like deoxynivalenol or fumonisin (particularly in developed countries) occurs often. This results in a far more complex (compared to that induced by AFB1 alone) series of immunomodulatory events in these exposed hosts (Chaytor et al. Citation2011; Gruber-Dorninger et al. Citation2019; Van der Fels-Klerx et al. Citation2019).
As noted above, tiny amounts of AFB1 are routinely found in agricultural commodities (Martins et al. Citation2007; Hernandez Hierro et al. Citation2008; Pukkala et al. Citation2009; Viegas et al. Citation2013; Mozafari et al. Citation2017). Accordingly, food-producing animals (and the humans that consume these meats and their associated products like milk) are at risk for potential immunosuppression from repeated low-levels of AFB1 exposures (Malvandi et al. Citation2013; Mohammadi et al. Citation2014; Mehrzad, Devriendt, et al. Citation2015; Mehrzad, Bahari, et al. Citation2018; Mehrzad, Hosseinkhani, et al. Citation2018). As the adult livestock are being exposed to this mycotoxin, it is inevitable their offspring are also likely impacted. The occurrence/intake of AFB1 (and its metabolite(s)) by neonatal bovines, camels, and humans has been documented (Cervino et al. Citation2008; Hernandez Hierro et al. Citation2008; Li et al. Citation2009; Mehrzad et al. Citation2011; Viegas et al. Citation2015). Given that ∼50% of AFB1 in contaminated feeds eventually enters into the bloodstream and ultimately various secreta/milk, it was unsurprising that in a 700 kg lactating cow that ingested 20 kg of feed (dry matter-based) contaminated with AFB1 at the European Union (EU) upper limit of acceptability (Gallo et al. Citation2008; Mehrzad et al. Citation2011), blood/secreta/milk levels of AFB1 (and associated metabolites) were seen to reach at as high as 10 ng/ml. Thus, a presence of detectable amounts of AFB1 in the environment and subsequently in the food chain (Martins et al. Citation2007; Pukkala et al. Citation2009; Viegas et al. Citation2013; Mozafari et al. Citation2017) poses a serious problem worldwide for potential chronic harm to important livestock and to humans.
Neutrophils (PMN) are an abundant pivotal circulating innate immune cell important for the induction of immune responses against invading pathogens (Hampton et al. Citation1998; Reeves et al. Citation2002; Asadollahi et al. Citation2015; Taheri et al. Citation2016). Fresh non-apoptotic PMN are rapidly recruited to sites of injury/infection whereupon they engulf and kill invading microorganisms. It is thus no surprise then that, critically, induction of neutrophil apoptosis (especially against these freshly mobilized highly active cells) is one hallmark of immunotoxicity induced by exogenous toxicants (Kennedy et al. Citation2004; Fialkow et al. Citation2006; Sudo et al. Citation2007; Koedel et al. Citation2009), including AFB1 (Mehrzad et al. Citation2011; Mehrzad, Devriendt et al. Citation2014).
While the function of PMN in many mammalian species has been well-studied, little is known about the PMN of two important mammals, e.g. cows and camels. Even less-investigated has been the impact of bovine/camelid age on functionality of these cells. It is known that like in most mammals, the immune system of bovine or camelid neonates is functionally immature and weak (Mehrzad et al. Citation2001, Citation2009; Mehrzad, Maleki, et al. Citation2014). On the other hand, it is not clear what (if any) impact ingestion of colostrum might have on the immune system of these animals. This is especially so with regard to their PMN. Unequivocally, prompt consumption of colostrum imparts huge benefits for neonates and helps with the maturation of their immune system (Weaver et al. Citation2000; Kamber et al. Citation2001; Gulliksen et al. Citation2008; Jrad et al. Citation2015). Nevertheless, even with this clear benefit, there have been no studies to date that have examined if colostrum is able to mitigate the potential adverse effects of toxicants, including AFB1, on mammalian immune cells.
Accordingly, the study described here was undertaken to help narrow the existing gap in our understanding of the functions of mammalian neonatal PMN (as potential model systems for broader use in immunotoxicology), to describe potential immunomodulatory effects from exposures of these cell types to naturally occurring levels of AFB1, and to determine if colostrum ingestion was able to halt/reverse any potential harm to these cells that might have been caused by AFB1.
Materials and methods
Animal selection, experimental design and blood sampling of bovine and camelid neonates
The neonates who provided the samples from which cells were isolated were from two commercial herds (each contained ∼1000 Holstein dairy cows (>370 lactating) and ∼300 one-humped camelids (Camelus dromedaries) (>70 lactating)) located on farms in Iran. Animal performance, management conditions, and colostrum intake on the farms were monitored to assure both mother and neonate health. The mean (minimum, maximum) relative temperature and absolute humidity in the study area during the study period were 9.6 °C (3.9, 15.4) and 64% (40, 87), respectively. One month before parturition, the healthy pregnant mothers were selected and separated from the rest of the herd. Immediately after birth, mother and neonate health status were thoroughly checked. Within 12 h of birth, a total of 5 and 4 l of their mother’s colostrum was twice given to the bovine and camel neonates, respectively. This was continued until Day 4 post-partum. Information on the experimental design and exact timing of the blood sampling of the neonates (before and after colostrum feeding) is found in . In all cases, neonate blood (n = 12/species; six male, six female) was collected into heparinized vacutainer tubes (Becton Dickinson, Franklin Lakes, NJ) from the external jugular vein. Blood samples were collected and analyzed/processed immediately after birth (before first colostrum consumption) and 3–4 days later (after final colostrum consumption).
Figure 1. Experimental design. Bovine and camelid neonatal PMN (isolated before vs. after colostrum consumption) were exposed to AFB1 (10 ng/ml) for 24 h. Comparative (para)clinical and hematological evaluations. Eosin/Giemsa staining of isolated PMN and whole blood smears. Magnification 1000×.

Hematological assessments, levels of total serum protein and fibrinogen, and the total numbers of circulating WBC, RBC, platelets, PMN, etc. were determined in all samples using a Coulter counter (Nihon Kohdem, Tokyo, Japan) and a Celltac X MEK-6450 auto-analyzer (Nihon Kohdem, Tokyo, Japan). Differentiation of nucleated blood cells was performed on eosin-/Giemsa-stained whole blood smears using light microscopy. From each blood sample, neonatal bovine and camelid PMN were isolated after hypotonic lysis of erythrocytes (described in Mehrzad, Maleki, et al. Citation2014). This procedure routinely yields populations of >95% PMN with >98% (trypan blue exclusion) viability. After washing with RPMI 1640 (R&D Systems, Minneapolis, MN), PMN suspensions were counted and adjusted to ∼5 × 106 viable PMN/ml in complete RPMI 1640 medium (containing 10% fetal calf serum, 2 mM L-glutamine, sodium pyruvate, non-essential amino acids, 100 U penicillin/ml, 100 μg streptomycin/ml, phenol red, and β-mercaptoethanol) (all from R&D Systems, Minneapolis, MN).
To ensure there was no AFB1 in the feed for and the colostrum of the cows and camels, an indirect ELISA were performed (Mozafari et al. Citation2017) using test kits specific for feedstuffs (EuroProxima, Beijerinckweg, The Netherlands) and bulk milk/colostrum (Ridascreen-AFM1, RBiopharm, Pfungstadt, Germany) and an ELx 800 plate reader (BioTek Instruments, Winooski, VT).
All aspects of this study design, including the blood sampling to derive PMN for use in the in vitro cell culture assays, were in accordance with the local animal welfare regulations and were approved by the animal ethics committee of Ferdowsi University of Mashhad.
AFB1-neonatal PMN co-culture
AFB1 powder was purchased from Sigma (St. Louis, MO); the AFB1 was derived from Aspergillus flavus (>98% pure) and certified to be free of lipopolysaccharide and other potential contaminating chemicals. The AFB1 powder was dissolved in 96% ethanol (to 0.1 mg/ml) as described previously (Mehrzad, Devriendt, et al. Citation2014). To assess the in vitro effects of AFB1 on neonatal blood PMN, AFB1 was then separately added, at a final concentration of 0 (control) or 10 ng/ml to PMN that were plated in 24-well plates in complete RPMI 1640 (PMN in each well were at ∼106 viable PMN/ml; 2 ml/well). The plates were then incubated for 24 h at 37 °C in a 5% CO2 incubator (Memmert, Schwabach, Germany). After incubation, the contents of each well were transferred to 1.5 ml microtubes and centrifuged (500×g, 4 °C, 5 min) to generate pellets. Each PMN pellet was immediately frozen at –20 °C, then stored at –80 °C for later use in the BL assays (ATP and caspases assays).
The inevitable occurrence of low-level AFB1-contamination in the food chain was one of the main concerns taken into account during the designing of the study here. The selection of the dose of 10 ng AFB1/ml here was done to reflect circulating levels of the mycotoxin (along with its metabolites) that could reach a neonate via its mother’s milk (Martins et al. Citation2007; Fink-Gremmels Citation2008; Gallo et al. Citation2008; Gruber-Dorninger et al. Citation2019; van der Fels-Klerx et al. Citation2019). This level was also based on the metabolism and toxico-dynamics of AFB1 using data acquired from many other studies of AFB1-exposed mammals (Gallo et al. Citation2008; Wu et al. Citation2009; Viegas et al. Citation2013, Citation2015; Mehrzad, Devriendt, et al. Citation2015; Mehrzad, Bahari, et al. Citation2018; Mehrzad, Hosseinkhani, et al. Citation2018; Mehrzad et al. Citation2020).
To confirm this specific dose used was below the expected LC50 for AFB1 in these PMN cytotoxicity of the AFB1 at the level used was evaluated in an MTT 3-(4,5-dimethylthiazol-2-yl)-2,5-diphenyltetrazolium bromide) tetrazolium reduction assay was employed. Detailed methods for the MTT assay are available in Präbst et al. (Citation2017). Data (see Supplemental Figure S1) indicated that this particular AFB1 level was in fact ≈3000-times lower than the predicted LC50 in these PMN.
Bioluminescence (BL) measures of AFB1-exposed bovine/camelid neonate PMN ATP
Levels of intracellular ATP in the PMN were measured using a luciferin-luciferase reaction-based BL assay. In brief, PMN pellets (containing ∼106 viable PMN/ml, in 1 ml total volume) were first gently lysed on ice by the addition of 150 µl cell culture lysis reagent (CCLR, details available on request) and repeated pipetting. The samples were then placed on a 4 °C shaker for 30 min and then centrifuged (2500×g, 4 °C, 5 min). Each cleared lysate was then used for measures of ATP levels. For this, an ATP complex was generated by mixing 100 µl MgSO4 (100 mM), 100 µl ATP (40 mM), and 400 µl luciferin (5 mM after dissolving in 50 mM Tris buffer (pH 7.8)), all brought to 1 ml final volume with Tris buffer. In the assay, 5 µl PMN lysate and 5 µl ATP complex with luciferase were combined and luminescence read in an FB21 Berthold tube luminometer (Berthold, Bad Wildbad, Germany). Data were recorded every 30 s for 1 min and then expressed in terms of cumulative relative light units (RLU/s) (Mehrzad, Bahari, et al. Citation2018; Vahidi-Ferdowsi et al. Citation2018). A six-point standard curve generated with ATP (0.001–0.5 mM) was generated for each assay, and ATP content in each test sample was extrapolated. The detailed methods and data for the ATP standard curve are provided in Supplemental Figure S2.
BL measures of AFB1-exposed bovine/camelid neonate PMN caspase-3, -7, and -9
Neonatal PMN caspase-3, -7, and -9 activities were assessed using luciferin-luciferase-based assays with Caspase-Glo®-3/7 and -9 assay kits (Promega, Madison, WI). For this, the above lysates (from ∼106 viable PMN/ml) were used. In brief, 2 µl luciferase (kit) were added to 3 µl cell lysate, and after gentle pipetting luminescence was read. In this case, data were recorded every 5 min for 25 min for caspases-3/-7 and every 30 s for 10 min for caspase-9. All data were then expressed as cumulative RLU/s; maximal caspases activity (counts or cumulative RLU/s) was separately reported and analyzed.
Statistical analyses
All data are presented as means ± SEM. Pre- and post-colostrum bovine/camelid neonatal PMN data were analyzed using SPSS software (v.21, IBM, Armonk, NY). Normality tests were first conducted to confirm that the responses in each group (AFB1-treated vs. non-treated control) were normally distributed. For comparative analyses, a Wilcoxon signed ranks test was used. Data were considered significantly different (AFB1-treated vs. non-treated control) at p < 0.05.
Results
AFB1 modifies cellular ATP levels in neonate PMN isolated pre-/post-colostrum
After 24 h of AFB1 exposure, changes in PMN intracellular ATP content were apparent. In cells isolated before the first colostrum consumption, ATP contents in both the bovine and camelid neonate PMN were increased 1.8-fold and 2.0-fold, respectively () vs. levels in species-specific control PMN by the AFB1 treatment. In cells harvested after colostrum consumption, 24-h AFB1-induced decreases in ATP levels were only by 33% and 25% in the bovine and camelid PMN, respectively () vs. in species-control PMN levels. In this study, BL intensity/ATP levels were not compared between the bovine and camelid PMN.
Figure 2. ATP levels in neonatal PMN. Exposure to AFB1 (10 ng/ml) for 24 h induced changes in ATP quantity in bovine (a, b) and camelid (c, d) neonatal PMN that were isolated before (a, c) vs. after (b, d) colostrum consumption. Differing superscripts indicate significant difference (p < 0.05). Values shown are mean ± SEM of 12 per species.
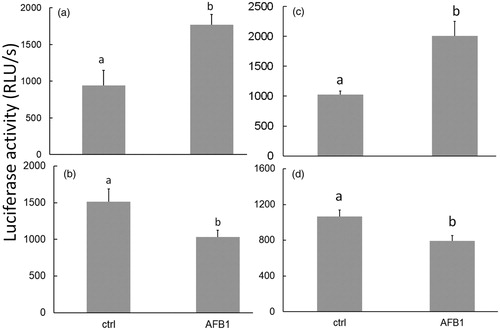
AFB1 induces caspase-3/-7 and -9 activation in neonate PMN isolated pre-/post-colostrum
After 24 h of AFB1 exposure, the BL-based apoptosis assays revealed there were higher caspase-3, -7, and -9 activities in all AFB1-treated PMN. Caspase-3 and -7 activity was increased (vs. levels in species-specific control PMN) 8.5- and 4.0-fold in bovine, and 4.0- and 2.5-fold in camelid, neonate PMN isolated before and after colostrum consumption, respectively (). Similarly, caspase-9 activity was increased 2.2- and 2.5-fold in bovine and 2.0- and 0.3-fold in camelid cells () vs. levels in species-specific control PMN. In this study, BL intensity/caspase activities were not compared between the bovine and camelid PMN.
Figure 3. Caspases-3, -7, and -9 activities in neonatal PMN. AFB1 (10 ng/ml for 24 h)-induced changes in caspase 3/7 (a–d) and 9 (e–h) activities in bovine (a, b, e, f) and camelid (c, d, g, h) neonatal PMN isolated before (a, c, e, g) vs. after (b, d, f, h) colostrum consumption. Differing superscripts indicate significant difference (p < 0.05). Values shown are mean ± SEM of 12 per species. Doxorubicin ((Dox) (insets)) was only used as positive control for apoptosis/caspases activity; these data were not compared to any of the other groups.
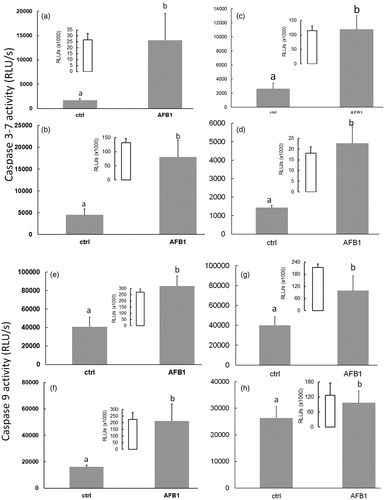
Discussion
The present study analyzed for the first time the damaging effects of AFB1 on bovine and camelid neonatal PMN, using cells isolated from neonates before and after their first colostrum consumption. The results indicated that a biologically relevant level of AFB1 (i.e. 10 ng/ml) induced apoptosis in these neonatal PMN through ATP depletion and caspase activation. Although the general merits of colostrum are well-known, colostrum consumption nonetheless failed to mitigate the AFB1-mediated bovine/camelid neonatal PMN injury ().
Figure 4. AFB1 can induce apoptosis via caspases 3/7 and 9 activation, and ATP depletion in bovine/camelid neonatal PMN, potentially through death-receptor-mediated, free radical, and mitochondrial pathways of cell apoptosis. In the intrinsic pathways, various apoptotic stimuli (e.g. ROS, other microenvironmental stresses) mediate permeabilization of the mitochondrial outer membrane and trigger signaling pathways. Within cytosol, cytochrome c together with Apaf-1 and dATP form apoptosome complexed to which the initiator of procaspase-9 is recruited and activated. Caspase-9-catalyzed activation of caspase-3 executes final steps of apoptosis. This schematic highlights what was detected in this study.
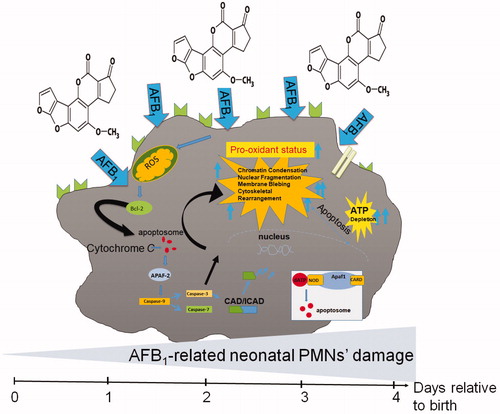
One key indicator of cells undergoing apoptosis (cell damage/injury) is intracellular levels of ATP – mainly due to its role in the regulation of caspase activity and apoptosome formation. Apoptosis (programmed cell death) is a highly regulated phenomenon that consists of several ATP-related physio-pathological pathways (Reed Citation2002; Zamaraeva et al. Citation2005). AFB1 is known to induce apoptosis in liver, kidney, thymus, spleen, cardiomyocytes, and the gastro-intestinal system, as well as in cells of immune system (Lewis et al. Citation2005; Bahari et al. Citation2014, Citation2015; Mehrzad, Devriendt, et al. Citation2014; Mohammadi et al. Citation2014; Mughal et al. Citation2017; Wang et al. Citation2017; Mehrzad, Bahari, et al. Citation2018). For example, it has been shown that AFB1 could impact on the functionalities of dendritic cells (DCs) (Mohammadi et al. Citation2014; Mehrzad, Hosseinkhani, et al. Citation2018), PMN (Mehrzad et al. Citation2011) and other leukocytes (Malvandi et al. Citation2013; Mehrzad et al. Citation2013). Research has also confirmed that AFB1 at very low doses can induce apoptosis and potential ATP depletion in various cell types (Wang et al. Citation2017; Mughal et al. Citation2017; Vahidi-Ferdowsi et al. Citation2018; Mehrzad, Bahari, et al. Citation2018); based on the changes in ATP, these outcomes might arise in part through caspase 3/7 activation.
In spite of all the research that has been performed on effects of AFB1 in relation to cancer (Mughal et al. Citation2017), immunity, necrosis, and apoptosis (Ubagai et al. Citation2008; Mehrzad et al. Citation2011, Citation2018, Citation2020; Mary et al. Citation2012; Bahari et al. Citation2014, Citation2015), mechanisms of action for AFB1 still remain to be clarified. While effects of AFB1 on a variety of immune cells have long been of intense interest (Mary et al. Citation2012; Bahari et al. Citation2014, Citation2015; Mohammadi et al. Citation2014), there is very little information on the potential impact of this toxicant on cells of two mammals of critical import around the world, i.e. camels and cows. This is all the more critical in that problems of fungal contamination of livestock feeds are always present in many places whose populations rely upon these hosts for livelihood/sustenance (Viegas et al. Citation2013; Mozafari et al. Citation2017). Though the LC50 of this toxin in the camel and bovine neonate PMN was assessed (see Supplemental Figure S1), further dose-dependency studies are still needed with these cells to more fully assess any/all toxic events that could be induced by the AFB1.
Another not-well studied factor in the (immuno)toxicity of AFB1 is related to host age. These data are clear that among mammals, newborns/young hosts are more susceptible to toxicities from this mycotoxin metabolite (Dimitri and Gabal Citation1996; Bailey et al. Citation1998). In the context of immune function, as in most mammals, the immune system of bovine or camelid neonates is functionally immature and weak (Mehrzad et al. Citation2001, Citation2009; Mehrzad, Devriendt, et al. Citation2014). For mammalian neonates, prompt consumption of colostrum imparts huge benefits and helps with the maturation of their immune system (Weaver et al. Citation2000; Kamber et al. Citation2001; Gulliksen et al. Citation2008; Jrad et al. Citation2015; Godden et al. Citation2019). It is still not clear what (if any) impact colostrum ingestion might have on the immune system of bovine or camelid neonates. Further, with any particular immune cell type, it is not certain colostrum ingestion might have any beneficial effect in general or against a given toxicant in particular (Gulliksen et al. Citation2008; Godden et al. Citation2019).
The data here showed that AFB1-PMN interactions could impact on neonatal bovine or camelid PMN differently before and after colostrum consumption. That levels of ATP and activities of caspases-9, -3, and -7 were still lowered by AFB1 (vs. control cell) regardless of colostrum consumption showed that the milk failed to impart “protection” in these neonates’ PMN against potential immunotoxic effects from AFB1. It is not yet clear how AFB1-metabolizing enzymes work in bovine or camelid neonatal PMN (regardless of before or after colostrum consumption). However, because the magnitude of the effects, it seems likely that post-colostrum consumed neonatal PMN are more matured and AFB1-induced effects seemed to now differ. It is plausible to assume that some protective enzymes (detoxifying) in the neonates became more functional as a result of the colostrum. Clearly, further investigation is warranted.
In the end, it is likely that many extra-/intracellular enzymes involved in AFB1 metabolism vary across different animal hosts as they due across (immune) cell types (Ubagai et al. Citation2008; Mehrzad, Bahari, et al. Citation2018; Mehrzad, Hosseinkhani, et al. Citation2018; Mehrzad et al. Citation2020). For example, in comparing bovine and camel cells, our laboratories have reported that levels of AFB1-metabolizing enzymes in monocytes are higher than in lymphocytes (Bahari et al. Citation2014, Citation2015). Nevertheless, it would seem from the data here that bovine and camelid neonatal PMN are more sensitive to AFB1 after than before colostrum consumption. Normally, colostrum activates PMN (Jrad et al. Citation2015; Godden et al. Citation2019) and activated PMN are more susceptible to AFB1 toxicity (Bahari et al. Citation2014, Citation2015). One might surmise that the bovine/camelid PMN cellular enzymes involved in AFB1 metabolism might have changed (in availability) from pre- to post-colostrum consumption, with levels/functions of AFB1-metabolizing enzymes increasing.
Neutrophil apoptosis is one critical means by which chemical agents induce immunotoxicity, immunoincompetence, and increases in disease severity in exposed hosts (Mehrzad et al. Citation2001; Kennedy et al. Citation2004; Mehrzad et al. Citation2001, Citation2009; Fialkow et al. Citation2006; Sudo et al. Citation2007; Koedel et al. Citation2009). Though the study here did not test the impact of changes in neonate PMN apoptosis on overall immune system functionality in the neonates themselves (which calls for additional studies), in previous work, it was seen that increases in PMN apoptosis due to AFB1 exposure resulted in diminished phagocytic/bactericidal activity of the cells (Mehrzad et al. Citation2011), as well as among lymphocytes and monocytes (Mehrzad et al. Citation2020). Thus, if the effects seen in vitro translate to the in situ scenario, a likely consequence of the increases in apoptosis in PMN seen here could be an increase in incidence/severity of inflammation, infection, and bacterial/viral diseases in the camel and cow offspring (Kennedy et al. Citation2004; Mehrzad et al. Citation2001, Citation2009; Fialkow et al. Citation2006; Sudo et al. Citation2007; Koedel et al. Citation2009).
To ascertain if the current study results reflect in vivo immunotoxicities – specifically in regard to host PMN, studies will be performed that involve direct exposures of the neonates (both pre- and post-colostrum consumption) to the mycotoxin. Precisely because the cow/camel neonates were not directly challenged with AFB1, it is difficult to conclude if colostrum would actually provide benefits to the immune system of any AFB1-exposed neonate. All that can be discerned from the current data is that colostrum consumption did/did not affect the PMN themselves such that they now responded differently to AFB1 as compared to cells from hosts with no colostrum consumption. Additional in vivo studies are needed to provide this clarification.
A final limitation of the study here was that although changes in PMN caspase activity and apoptosis (hallmarks of cell toxicity/damage) were noted, no other validation endpoints such as TUNEL/Annexin V staining, measures of cytochrome c release from mitochondria, and/or morphologic analyses of apoptosis, were undertaken. Ongoing studies in our laboratories are performing these various measurements to confirm the outcomes reported here.
It was previously observed in earlier studies that maternal transfer of immunity to neonates (i.e. through colostrum consumption) can be disrupted/weakened by AFB1. This means that even if all health/performance management conditions for the ruminants’ and their neonates are optimal, a presence of even tiny amounts of AFB1 in the feed and/or colostrum/milk could adversely interfere with both mother and neonate immunity and overall health. Thus, stringent monitoring of AFB1 in colostrum/milk and in both maternal and neonatal feed is absolutely required.
Supplemental Material
Download MS Word (100 KB)Disclosure statement
No potential conflict of interest was reported by the author(s).
Additional information
Funding
References
- Asadollahi F, Mehrzad J, Chaichi M, Taghavi-Razavizadeh A. 2015. Photoimmunological properties of borage in bovine neutrophil in vitro model. J Photochem Photobiol. 151:270–275.
- Bahari A, Mehrzad J, Mahmoudi M, Bassami M, Dehghani H. 2014. Cytochrome P450 isoforms are differently up-regulated in AFB1-exposed human lymphocytes and monocytes. Immunopharmacol Immunotoxicol. 36(1):1–10.
- Bahari A, Mehrzad J, Mahmoudi M, Bassami M, Dehghani H. 2015. GST-M1 is transcribed moreso than AKR7A2 in AFB1-exposed human monocytes and lymphocytes. J Immunotoxicol. 12(2):194–198.
- Bailey G, Dashwood R, Loveland P, Pereira C, Hendricks J. 1998. Molecular dosimetry in fish: Quantitative target organ DNA adduction and hepato-carcinogenicity for four aflatoxins by two exposure routes in rainbow trout. Mutat Res. 399(2):233–244.
- Cervino C, Asam S, Knopp D, Rychlik M, Niessner R. 2008. Use of isotope-labeled aflatoxins for LC–MS/MS stable isotope dilution analysis of food. J Agric Food Chem. 56(6):1873–1879.
- Chaytor A, See M, Hansen J, de Souza A, Middleton T, Kim S. 2011. Effects of chronic exposure to diets with reduced concentrations of aflatoxin and deoxynivalenol on growth and immune status of pigs. J Anim Sci. 89(1):124–135.
- Dimitri R, Gabal M. 1996. Immunosuppressant activity of aflatoxin ingestion in rabbits measured by response to Mycobacterium bovis antigen. I. Cell mediated immune response measured by skin test reaction. Vet Hum Toxicol. 38:333–336.
- Fialkow L, Fochesatto Filho L, Bozzetti M, Milani A, Rodrigues Filho E, Ladniuk R, Pierozan P, de Moura R, Prolla J, Vachon E, et al. 2006. Neutrophil apoptosis: Marker of disease severity in sepsis and sepsis-induced acute respiratory distress syndrome. Crit Care. 10(6):R155.
- Fink-Gremmels J. 2008. Mycotoxins in cattle feeds and carry-over to dairy milk: A review. Food Addit Contam. 25(2):172–180.
- Gallo A, Moschini M, Masoero F. 2008. Aflatoxins absorption in the gastrointestinal tract and in the vaginal mucosa in lactating dairy cows. Ital J Anim Sci. 7(1):53–63.
- Godden S, Lombard L, Woolums A. 2019. Colostrum management for dairy calves. Vet Clin North Am Food Anim Pract. 35(3):535–556.
- Gruber-Dorninger C, Jenkins T, Schatzmayr G. 2019. Global mycotoxin occurrence in feed: A 10-year survey. Toxins (Basel). 11(7):375.
- Gulliksen S, Lie K, Solverod L, Osteras O. 2008. Risk factors associated with colostrum quality in Norwegian dairy cows. J Dairy Sci. 91(2):704–712.
- Hampton M, Kettle A, Winterbourn C. 1998. Inside the neutrophil phagosome: Oxidants, myeloperoxidase, and bacterial killing. Blood. 92(9):3007–3017.
- Hernandez Hierro J, Garcia-Villanova R, Rodriguez Torrero P, Toruno Fonseca I. 2008. Aflatoxins and ochratoxin A in red paprika for retail sale in Spain: Occurrence and evaluation of a simultaneous analytical method. J Agric Food Chem. 56:751–756.
- Jeannot E, Boorman G, Kosyk O, Bradford B, Shymoniak S, Tumurbaatar B, Weinman S, Melnyk S, Tryndyak V, Pogribny I, et al. 2012. Increased incidence of AFB1-induced liver tumors in hepatitis virus C transgenic mice. Int J Cancer. 130(6):1347–1356.
- Jrad Z, Oulahal N, Adt I, Khorchani T, Degraeve P, El-Hatmi H. 2015. Camel colostrum: Nutritional composition and improvement of anti-microbial activity after enzymatic hydrolysis. Emir J Food Agric. 27:384–389.
- Kamber R, Farah Z, Rusch P, Hassig M. 2001. Studies on the supply of immunoglobulin G to newborn camel calves (Camelus dromedarius). J Dairy Res. 68(1):1–7.
- Kennedy G, Spence V, Underwood C, Belch J. 2004. Increased neutrophil apoptosis in chronic fatigue syndrome. J Clin Pathol. 57(8):891–893.
- Koedel U, Frankenberg T, Kirschnek S, Obermaier B, Häcker H, Paul R, Häcker G. 2009. Apoptosis is essential for neutrophil functional shutdown and determines tissue damage in experimental pneumococcal meningitis. PLOS Pathog. 5(5):e1000461.
- Lewis L, Onsongo M, Njapau H, Schurz-Rogers H, Luber G, Kieszak S, Nyamongo J, Backer L, Dahiye AM, Misore A, 2005. Aflatoxin contamination of commercial maize products during an outbreak of acute aflatoxicosis in Eastern and Central Kenya. Environ Health Perspect. 113(12):1763–1768.
- Li F, Li Y, Wang Y, Luo X. 2009. Natural occurrence of aflatoxins in Chinese peanut butter and sesame paste. J Agric Food Chem. 57(9):3519–3524.
- Malvandi A, Mehrzad J, Saleh-Moghaddam M. 2013. Biologically-relevant doses of mixed aflatoxins B and G up-regulate MYD88, TLR2, and CD14 transcripts in human PBMC. Immunopharmacol Immunotoxicol. 35(4):528–532.
- Marroquín-Cardona A, Johnson N, Phillips T, Hayes A. 2014. Mycotoxins in a changing global environment – A review. Food Chem Toxicol. 69:220–230.
- Martins H, Mendes Guerra M, d’Almeida Bernardo F. 2007. Occurrence of AFB1 in dairy cow feed over 10 years in Portugal (1995–2004). Rev Iberoam Micol. 24(1):69–71.
- Mary V, Theumer M, Arias S, Rubinstein H. 2012. Reactive oxygen species sources and biomolecular oxidative damage induced by AFB1 and fumonisin B1 in rat spleen mononuclear cells. Toxicology. 302(2–3):299–307.
- Mehrzad J, Bahari A, Bassami M, Mahmoudi M, Dehghani H. 2018. Immunobiologically-relevant level of AFB1 alters transcription of key functional immune genes, phagocytosis, and survival of human dendritic cells. Immunol Lett. 197:44–52.
- Mehrzad J, Devriendt B, Baert K, Cox E. 2015. Aflatoxins of type B and G affect porcine dendritic cell maturation in vitro. J Immunotoxicol. 12(2):174–180.
- Mehrzad J, Devriendt B, Baert K, Cox K. 2014. AFB1 interferes with the antigen-presenting capacity of porcine dendritic cells. Toxicol In Vitro. 28(4):531–537.
- Mehrzad J, Dosogne H, Vangroenweghe F, Burvenich C. 2001. A comparative study of bovine blood and milk neutrophil functions with luminol-dependent chemiluminescence. Luminescence. 16:343–356.
- Mehrzad J, Duchateau L, Burvenich C. 2009. Phagocytic and bactericidal activity of blood and milk-resident neutrophils against Staphylococcus aureus in primiparous and multiparous cows during early lactation. Vet Microbiol. 134(1–2):106–112.
- Mehrzad J, Fazel F, Pouyamehr N, Hosseinkhani S, Dehghani H. 2020. Naturally-occurring level of AFB1 injures human, canine and bovine leukocytes through ATP depletion and caspase activation. Int J Toxicol.
- Mehrzad J, Hosseinkhani S, Malvandi A. 2018. Human microglial cells undergo proapoptotic induction and inflammatory activation upon in vitro exposure to a naturally-occurring level of AFB1. Neuroimmunomodulation. 25(3):176–183.
- Mehrzad J, Klein G, Kamphues J, Wolf P, Grabowski N, Schuberth H. 2011. In vitro effects of very low levels of AFB1 on free radical production and bactericidal activity of bovine blood neutrophils. Vet Immunol Immunopathol. 141(1–2):16–25.
- Mehrzad J, Mahmudy Gharaie M, Taheri M. 2017. Effects of arsenic on porcine dendritic cells in vitro. J Immunotoxicol. 14(1):1–8.
- Mehrzad J, Maleki M, Raji A, Razmi G. 2014. An ultrastructural investigation of the blood neutrophils in camel (Camelus dromedarius). Comp Clin Pathol. 23(4):885–892.
- Mehrzad J, Milani M, Mahmoudi M. 2013. Naturally occurring level of mixed aflatoxins B and G stimulate toll-like receptor-4 in bovine mononuclear cells. Vet Quart. 33(4):186–190.
- Mehrzad J, Shajari M, Saleh-Moghaddam M, Sarmad-Nabavi M. 2015. Stressed (acute) mice display neuro-immuno-dysregulation and defective innate immune response against coliform infection. Int Immunopharmacol. 28(1):168–174.
- Mohammadi A, Mehrzad J, Mahmoudi M, Schneider M. 2014. Environmentally-relevant level of AFB1 dysregulates human dendritic cells through signaling on key Toll-like receptors. Int J Toxicol. 33(3):175–186.
- Mozafari S, Mohsenzadeh M, Mehrzad J. 2017. Seasonally feed-related aflatoxins B1 and M1 spread in semiarid industrial dairy herd and its deteriorating impacts on food and immunity. J Food Qual. 2017:1–8.
- Mughal MJ, Peng X, Zhou Y, Fang J. 2017. AFB1 invokes apoptosis via death receptor pathway in hepatocytes. Oncotarget. 8(5):8239–8249.
- Präbst K, Engelhardt H, Ringgeler S, Hübner H. 2017. Basic colorimetric proliferation assays: MTT, WST, and resazurin cell viability assays. Methods Mol Biol. 1601:1–17.
- Pukkala E, Martinsen J, Lynge E, Gunnarsdottir H, Sparén P, Tryggvadottir L, Weiderpass E, Kjaerheim K. 2009. Occupation and cancer: Follow-up of 15 million people in five Nordic countries. Acta Oncol. 48(5):646–790.
- Reed J. 2002. Apoptosis-based therapies. Nat Rev Drug Discov. 1(2):111–121.
- Reeves E, Lu H, Jacobs H, Messina C, Bolsover S, Gabella G, Potma E, Warley A, Roes J, Segal A. 2002. Killing activity of neutrophils is mediated through activation of proteases by K+ flux. Nature. 416(6878):291–297.
- Sudo C, Ogawara H, Saleh A, Nishimoto N, Utsugi T, Ooyama Y, Fukumura Y, Murakami M, Handa H, Tomono S, et al. 2007. Clinical significance of neutrophil apoptosis in peripheral blood of patients with Type 2 diabetes mellitus. Lab Hematol. 13:1008–1012.
- Taheri M, Mehrzad J, Afshari R, Saleh-Moghaddam M, Mahmudy Gharaie M. 2016. Inorganic arsenic can be potent granulotoxin in mammalian neutrophils in vitro. J Immunotoxicol. 13(5):686–693.
- Ubagai T, Tansho S, Ito T, Ono Y. 2008. Influences of AFB1 on reactive oxygen species generation and chemotaxis of human polymorphonuclear leukocytes. Toxicol In Vitro. 22(4):1115–1120.
- Vahidi-Ferdowsi P, Mehrzad J, Malvandi A, Hosseinkhani S. 2018. Bioluminescence-based detection of astrocytes apoptosis and ATP depletion induced by biologically-relevant level AFB1. World Mycotoxin J. 11(4):589–598.
- Van der Fels-Klerx H, Vermeulen L, Gavai A, Liu C. 2019. Climate change impacts on AFB1 in maize and AFM1 in milk: A case study of maize grown in Eastern Europe and imported to the Netherlands. PLOS One. 14(6):e0218956.
- Viegas S, Veiga L, Figueiredo P, Almeida A, Carolino E, Viegas C. 2015. Assessment of workers' exposure to aflatoxin B1 in a Portuguese waste industry. Ann Occup Hyg. 59(2):173–181.
- Viegas S, Veiga L, Figueredo P, Almeida A, Carolino E, Sabino R, Veríssimo C, Viegas C. 2013. Occupational exposure to AFB1 in swine production and possible contamination sources. J Toxicol Environ Health. 76(15):944–951.
- Wang W, Xu Z, Yu C, Xu X. 2017. Effects of AFB1 on mitochondrial respiration, ROS generation, and apoptosis in broiler cardiomyocytes. Anim Sci J. 88(10):1561–1568.
- Weaver D, Tyler J, van Metre D, Hostetler D, Barrington G. 2000. Passive transfer of colostral immunoglobulins in calves. J Vet Intern Med. 14(6):569–577.
- Wu Q, Jezkova A, Yuan Z, Pavlikova L, Dohnal V, Kuca K. 2009. Biological degradation of aflatoxins. Drug Metab Rev. 41(1):1–7.
- Zamaraeva MV, Sabirov RZ, Maeno E, Ando-Akatsuka Y, Bessonova SV, Okada Y. 2005. Cells die with increased cytosolic ATP during apoptosis: A bioluminescence study with intracellular luciferase. Cell Death Differ. 12(11):1390–1397.