ABSTRACT
Introduction
Respiratory diseases represent a worldwide health issue. The recent Sars-CoV-2 pandemic, the burden of lung cancer, and inflammatory respiratory diseases urged the development of innovative therapeutic solutions. In this context, therapeutic antibodies (Abs) offer a tremendous opportunity to benefit patients with respiratory diseases. Delivering Ab through the airways has been demonstrated to be relevant to improve their therapeutic index. However, few inhaled Abs are on the market.
Areas covered
This review describes the different barriers that may alter the fate of inhaled therapeutic Abs in the lungs at steady state. It addresses both physical and biological barriers and discusses the importance of taking into consideration the pathological changes occurring during respiratory disease, which may reinforce these barriers.
Expert opinion
The pulmonary route remains rare for delivering therapeutic Abs, with few approved inhaled molecules, despite promising evidence. Efforts must focus on the intertwined barriers associated with lung diseases to develop appropriate Ab-formulation-device combo, ensuring optimal Ab deposition in the respiratory tract. Finally, randomized controlled clinical trials should be carried out to establish inhaled Ab therapy as prominent against respiratory diseases.
1. Introduction
Therapeutic antibodies (Ab) have become a major class of therapeutics with nearly 1200 Abs in clinical studies and ~175 in late-stage clinical development or regulatory review [Citation1]. Many Abs are used as first-line standard of care for cancer, inflammatory, metabolic, and infectious diseases [Citation2] thanks to their multifaceted properties and good safety profile (). For the readers who like a broader view on Ab structure and function, we invite them to read recent reviews [Citation3–5]. Abs is known to have a remarkable expansion due to the global response to the SARS-CoV2 pandemic. However, non-Covid-19 late-stage clinical pipeline grew by ~20% in the past year, making Abs the predominant molecules in the arena of biotherapeutics.
Due to their size and polarity, Abs (IgG) displayed limited membrane permeability. This will influence their absorption and biodistribution after administration, giving Ab a unique pharmacokinetic profile, which will mainly depend on the route of administration [Citation6,Citation7]. Historically, Abs were commonly delivered by intravenous (i.v.) infusion, followed by subcutaneous (s.c.) and intramuscular (i.m.) injection. Although these parenteral routes result in a high systemic bioavailability, they provide limited local distribution in the disabled peripheral organs, resulting in low Ab amount in the vicinity of the targeted antigen. This may explain the injection (i.v.) of high dose of Ab to achieve a therapeutic response, which may expose the host to potential toxicity while increasing treatment cost. Consequently, there has been an increasing trend toward the consideration and development of alternative delivery modalities to maximize Ab’s therapeutic index.
Respiratory diseases are one of the leading causes of mortality worldwide, which is mainly attributable to lung cancer, inflammatory diseases, and respiratory tract infections [Citation8]. The recent SARS-CoV-2 pandemic, the overall development of antimicrobial resistance, seasonal virus outbreaks, and the increase in atmospheric pollutants have severely aggravated the prognosis of airway diseases urging for the development of alternative therapeutic strategies. In this context, Abs emerged as valuable therapeutics to tackle multiple respiratory diseases, as exemplified by the clinical successes of Obiltoxaximab/Raxibacumab against pulmonary anthrax, Omalizumab in severe asthma and Pembrolizumab in lung cancer [Citation9–11]. The route of administration of Ab depends on the disease context: for lung cancer and respiratory infections, the conventional route is i.v., whereas the s.c. route is preferred in chronic respiratory diseases, such as asthma. Oral inhalation is often the gold standard for small molecules used for the treatment of inflammatory respiratory diseases, like corticosteroids and bronchodilators in asthma and chronic obstructive pulmonary disease (COPD). Inhalation provides direct access to both upper and lower respiratory tract accelerating the onset of action and improving therapeutic index for pathological targets located there [Citation12,Citation13]. Although many approved Abs for respiratory disease are delivered through systemic routes, inhalation has been extensively considered for therapeutic Abs undergoing both preclinical studies and clinical evaluation [Citation14–17]. Despite the obvious theoretical advantages of topical administration for Ab by inhalation, few of these benefits have materialized in the clinics yet. Indeed, after the heyday of nasal and pulmonary biopharmaceuticals in the late 1990s, there have been few successes with only one therapeutic protein currently approved by inhalation – Pulmozyme™, a recombinant human DNase I – for cystic fibrosis (CF) patients. Presently, there are five inhaled Abs in clinical trial (). This paucity underlines the complexity of developing inhaled Abs and points out the persisting hurdles to overcome. A detailed understanding of the biology and pharmacology of inhaled Ab is required to ensure the successful transition from pre-clinical to clinical assessment. The present review describes the physical, biochemical, and cellular barriers faced by Ab during and after inhalation, and shows that it may be worth considering developing successful inhaled Abs.
Table 1. Clinical development of therapeutic antibodies for respiratory diseases.
2. Antibody deposition in the airways after inhalation
2.1. Impact of lung anatomy and ventilation
Lung's branched architecture represents the first physical barrier encountered by inhaled molecules. Lungs consist of multiple tubular bifurcation starting from the trachea, bronchi, and bronchioles and ultimately reaching the alveoli where gas exchanges occur. Inhaled molecules, propelled by air flow generated through breathing or external ventilation (involving breathing pattern, tidal volume, flow rates), will be forced to deposit on respiratory surface, which progressively subdivide and reduce their diameter (from 1.5 cm in trachea to less than 200 µm in the airways). Conducting airways (trachea + bronchi) represent a volume of ~150 mL, while the respiratory zone, which encompasses alveoli, aggregates 2.5–3 L. The whole respiratory surface is estimated to be up to 150 m2, facing the external environment especially in the terminal alveolar region. Besides anatomy, physiology of the airways will influence inhaled particle deposition. Its complex geometry, humidity, and breathing pattern will impact the deposition of inhaled particles, while the mucociliary clearance mechanism will eliminate particles trapped in the mucus layer toward the gastrointestinal tract.
These anatomic considerations influence the general principles for inhaled particle deposition on the respiratory surface governed by impaction, sedimentation, and diffusion. These forces will also depend on the aerodynamics, shape, density, and hydrophilic properties of the particle [Citation18]. The mass median aerodynamic diameter (MMAD) of a particle is of particular importance. MMAD is defined as the diameter of a sphere, with a density of 1 g cm−3, which settles in still air with the same velocity as the particle in question [Citation19]. In the large conducting airways, where high speed and turbulent air flow is found, deposition of inhaled particles with MMAD > 5–10 µm is governed by impaction. In the conductive airways, where the air velocity is slower, gravitational sedimentation is the main deposition mechanism for particles with a MMAD ~ 0.5–5 µm [Citation20]. Finally, Brownian diffusion and electrostatic attraction will dictate the diffusion of particle with MMAD <1 µm, especially in the small airways and alveoli where the air velocity is close to zero [Citation21]. These physical considerations for particle deposition are also appropriate for inhaled Ab, contained in dry or liquid aerosol particles.
2.2. Impact of the inhalation device and antibody formulation
Numerous studies have concluded on the development of rationally designed formulations and inhaler devices to enable suitable aerodynamic properties and promote deposition of inhaled drugs to the appropriate target site [Citation22,Citation23].
2.2.1. Importance of the device
2.2.1.1. Devices for antibody inhalation
Three main types of inhalers are used for medical applications including dry powder inhalers (DPI), pressurized metered-dose inhalers (pMDI) and nebulizers.
DPI deliver solid aerosols and the technology contains three major components – a solid/powder formulation, the metering system, and the aerosol dispersion part. The main challenge consists in de-agglomerating the dry powder containing the active ingredients in micronized particles to be deposited in the lungs. For Ab, another challenge is the possibility of delivering relatively high dose and thus obtaining powders with high concentration of Ab. In comparison to the extensive amount of literature on the development of formulation of protein therapeutics (including Ab) in a dried, solid state, investigations on inhalable dried powders of Ab via DPI remain negligible, but still relevant. It is noteworthy that some Ab-based therapeutics were successfully developed to be delivered by a DPI [Citation24–26]. One reason may be that DPI provides faster administration time and better portability, as compared to nebulizers, thereby being more patient-friendly and possibly improving compliance/adherence of patients with chronic respiratory diseases.
Metered dose inhaler technology contains solutions or suspensions and uses propellants during the atomization process, which are usually not compatible with protein therapeutics. Thus, pulmonary delivery of Ab with a pressurized metered-dose inhaler (pMDI) is poorly explored [Citation14,Citation15].
Nebulizers (jet, ultrasonic, and mesh) are often used as a first step in the development process of inhalation-based administration of protein therapeutics. Accommodating liquid solution or suspension, they allow inhalation of larger volumes with often greater pulmonary deposition than DPI and nebulization is applicable in all clinical situations. Moreover, nebulized formulations avoid drying steps; thus, their development is less time consuming and expensive. Weak points of nebulization rely on longer administration time and reduced liquid formulation stability.
To the best of our knowledge, Ab delivery by soft-mist inhalers, a new class of devices for large-dose medication, generating a liquid spray, has not been reported yet. New technologies, such as surface acoustic waves (SAW) nebulizers, have been reported for aerosolization of Abs, with some success and may be considered for pulmonary delivery of Ab-based therapeutics in the future [Citation27,Citation28].
2.2.1.2. Antibody for inhalation
Abs are mainly formulated as a solution for nebulization or dry powder for DPIs for pulmonary delivery.
Ab may encounter stressing mechanisms during both drying (heating, air–liquid interface, etc.) and aerosolization (freezing, shearing forces, etc.) processes, which may necessitate special inputs to avoid Ab denaturation or degradation.
Freeze drying, spray drying, and spray-freeze-drying are different drying techniques for proteins, the latter being less common in industry [Citation23,Citation24]. Thin-film freeze-drying, a cryogenic technique, has also been applied to several Ab and resulted in stable powders with aerosol performances compatible for pulmonary delivery [Citation25]. Drying techniques expose Ab to thermal stress, shear force and interface stress. Recently, Brunaugh et al. showed that IgG1 is particularly sensitive to air–ice interfaces during spray freeze drying, reducing storage stability of Ab [Citation26]. Despite the multiple stress associated with the development of inhalable dry-powder Ab, two spray-dried Ab powders have been developed and tested in the clinic [Citation15]. VR942 is an anti-IL-13 Fab fragment blocking the pro-inflammatory action of IL-13 was tested in a phase I trial in asthma patients and reported to be safe. CSJ117 is an anti-thymic stromal lymphopoietin (TSLP) Fab fragment, stopped after clinical phase 2 in asthma (). In the phase I trial, it was demonstrated to be safe and well tolerated by patients with mild asthma. Although DPI often suffer from lower deposition in the lungs as compared to nebulizers, the dried-powder formulation of CSJ117 was able to achieve a total lung dose of app. 95% of the delivered dose.
Nebulization exposes Abs to several stresses: a huge air–liquid interface (24 m2-1500 m2) where proteins have a tendency to adsorb, denature, and aggregate. For jet and ultrasonic nebulizers, additional stresses may include temperature rise and protein recirculation. Among the three main type of nebulizers (jet, ultrasonic, and mesh-nebulizers), the latter have been shown to be the least deleterious for Ab regarding the generation of aggregates [Citation28]. Besides aggregation, chemical insults may also occur during nebulization, in particular, oxidation, which has been observed for other protein therapeutics [Citation29]. Several Abs have been developed for mesh-nebulization and reached clinical trials, including anti-SARS-CoV-2 Ab which are currently under evaluation [Citation28,Citation30]. It is noteworthy that the vulnerability extent of Abs to these stresses is highly dependent on the molecule and the device. Therefore, the choice of the Ab itself (scaffold etc.) and the device technology must be considered carefully and very early in the development.
2.2.2. Importance of the formulation
Abs are susceptible to multiple stresses from bioproduction up to storage and administration to patients. Thus, Ab stability should be monitored efficiently along its development process, and efforts must be made on developing an adequate formulation to ensure quality and safety of the final Ab product. In fact, the simple repurposing of the Ab formulation developed for i.v. is not appropriate as stresses (shaking, temperature, etc.) applied during the pharmaceutical development of parenteral Ab are not like the ones of nebulization [Citation31]. Formulation design for inhaled Ab must prevent protein aggregation, degradation, and/or produce amorphous powders that are easily dispersible to minimize lung toxicity. As previously demonstrated for Ab delivered parenterally, this could be achieved by the rational selection of appropriate excipients and buffering system [Citation29,Citation32–34]. Ab can be stabilized with a variety of molecules including salts, surfactants, sugars, polyols, and amino acids. However, the choice of excipients generally recognized as safe for the pulmonary delivery is very limited due to the lack of studies assessing their toxicity by the oral inhalation route.
For dried proteins, stabilizers encompass a variety of molecules including salts, surfactants, sugars and polyols, and amino acids, but only a few of them are approved for pulmonary delivery. For example, among sugars, only lactose, mannitol, and glucose are accepted for pulmonary delivery. The right type of buffering agents, their concentration, and pH should be chosen appropriately since they may influence the propensity of Ab to aggregate. For example, buffering agent and pH were shown to limit aggregation and deamidation during the drying of an anti-IL-13 fragment. Sugars (sucrose, trehalose, raffinose, and lactose) and polyols (mannitol) protect proteins against physical and chemical degradation, in particular, during the dehydration step. However, lactose, which is often used as a drug carrier in DPI, may undergo the Maillard reaction, interacting with amines, and may not be less appropriate for proteins. They may also contribute to produce fine powders suitable for pulmonary deposition, as exemplified for an anti-IgE Ab. In dried powder formulations, amino acids act through various mechanisms, protecting proteins from thermal and interfacial stress, functioning as a buffering agent, and improving powder dispersion. For example, the addition of trileucine to the dry powder formulation of CSJ117 Fab fragment improved drug deposition [Citation35,Citation36].
In liquid formulation, stabilizers include the buffering system, salts, and surfactants. The buffering agent and pH adjusting agents have been shown to greatly influence Ab aggregation, as they maintain pH and protein stability [Citation29], [Citation37]. In fact, citrate buffer was found to be less protective for IgG1, during mesh-nebulization [Citation28]. Salts are used to adjust osmolarity, generating formulation adequate for airway delivery [Citation34]. Surfactant, like polysorbate, is used to prevent adsorption at interfaces (air-liquid, liquid-solid, etc.), by displacing protein from interfaces, and have proven successful in stabilizing Ab during nebulization [Citation31,Citation38,Citation39].
2.3. 1. Consequences of aggregation during Ab aerosolization
As mentioned above, due to their labile structure, Abs are sensitive to multiple chemical and physical stresses during aerosolization. This may ultimately lead to physical degradation including denaturation and, at the end, promoting aggregation [Citation40]. Disruption of disulfide bond disruption or peptide bond cleavage leads to Ab fragmentation. Besides physical modifications, Abs are also susceptible to chemical degradations including amino-acid oxidation, or deamidation which may occur due to exposure to moisture or through interaction with certain excipients. Physical and chemical aggressions are intertwined, and both will ultimately induce aggregation [Citation40,Citation41]. Aggregates are assembled with native and/or unfolded Ab linked together by weak interactions or covalent bonds in a soluble or insoluble form.
Ab sensitivity to aggregation is dependent on intrinsic features like their primary and tertiary structure, their subclass, or isoelectric point of their CDR, but also extrinsic conditions such Ab concentration, formulation, etc. It must be noted that analyzing aggregation of Ab following nebulization requires collecting/condensating the aerosol back into a solution to implement analytical methods (dynamic light scattering, SEC-HPLC, etc.). Collection devices will interfere with Ab stability and may be taken into consideration for aggregation monitoring [Citation42].
The impact of chemical and physical degradations on Ab may be highly dependent on their location and the nature of the aggregates produced [Citation40]. For instance, chemical degradation in the Fc fragment may interfere with Fc-effector functions and interactions with FcRn, thereby modifying Ab pharmacodynamics and pharmacokinetics. Chemical degradation occurring in the CDR may impair Ab binding to its target antigen and loss of potency. Aggregation may modify inhaled Ab potency, increase or loss, as recently reported [Citation42]. Aggregation is of concern regarding toxicity of therapeutic Ab. In fact, aggregation is known to be a major factor promoting immunogenicity and the generation of antidrug antibody (ADA) [Citation43]. Immunogenicity is defined as a dysregulated activation of the host immune system leading to various deleterious reactions ranging from hypersensitivity to anaphylaxis or cytokine release syndrome. ADA causes the alteration of Ab pharmacokinetic profile, abrogation of its pharmacological activity, and through the formation of an immune-complex with the drug, activation of immunotoxicity [Citation44]. The pathological mechanisms underlying immunotoxicity are diverse and may be related to the route of administration of the drug. In fact, it was recently shown that Ab aggregates generated during mesh-nebulization induced a profound and sustained local and systemic depletion of immune cells after delivery through the pulmonary route rather than after systemic administration [Citation45]. It appears critical to control physical and chemical degradations of inhaled Ab to minimize risks for patients and evaluate the immunogenicity of Ab aggregates as a precautionary approach based on these results and the correlation of aggregation and immunogenicity of parenteral Ab products.
3. Impact of biological barriers on Ab lung delivery
The different biological barriers/changes that a full-length Ab may face after deposition into the respiratory tract are illustrated in . Their impact may be slightly different depending on the format/structure of Ab-based therapeutics.
Figure 2. Biological barriers/changes that inhaled Ab-based therapeutics may face after deposition into the respiratory tract. Illustrated for a full-length Ab (burgundy). AM: alveolar macrophage (created with Biorender.com).
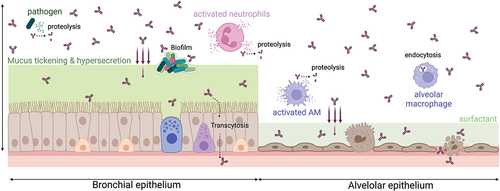
3.1. Mucociliary barrier
Mucus is a viscoelastic hydrogel coating the epithelial surface of the upper and central lung. Continuously produced and secreted by mucous cells including Goblet cells, club cells, and other submucosal glands, it acts as a lubricant maintaining moisture above the epithelium [Citation46]. It also provides a barrier to billions of pathogens and environmental particles that are inhaled daily [Citation47]. Mucus is mainly composed of water (>95%), protein – whose main representant are mucins – DNA, lipids, electrolytes, and cellular debris [Citation46,Citation48]. Mucins are glycoproteins with periodic carboxy- and amino-terminal domains conferring to the mucus a three-dimensional mesh network structure stabilized by hydrophobic, electrostatic, hydrogen bonds, or other specific binding interactions [Citation49–51]. Mucin homeostasis dictates rheological properties of the mucus characterized by viscoelasticity, pH, ionic strength, and charge [Citation46]. These properties will give mucus its main features, with pore size ranging from 10 to 100 nm and thickness around several micrometers (thickness being higher in the trachea than in the bronchi) [Citation52].
Several studies have investigated the behavior of biotherapeutics in the respiratory, GI, and cervico-vaginal physiological mucus with conflicting results. While some studies have reported that Ab diffusion was barely altered in mucus [Citation53], other showed a reduced diffusion coefficient [49]. Determining the fate of Abs in the respiratory mucus is of importance as entrapped particles will be expelled tower the pharynx/larynx before being swallowed, thanks to the coordinated beating of cilia lining the upper airways, a mechanism known as mucociliary clearance (MCC). For instance, it has been shown that a substantial fraction of anti-IL17 fragment was removed by MCC after intratracheal delivery [Citation54]. Besides impaired diffusion through the mucus due to molecular hindrance, Abs, as they are hydrophilic molecules, will tightly and polyvalently interact with the negatively charged glycan domains of mucin, limiting drug diffusion throughout the mucus [Citation55]. Interestingly, it has been shown that the Fc fragment of Abs is the moiety exhibiting the highest electrostatic interactions with mucin fibers, impairing significantly the diffusion of multimeric (IgA, IgM) Ab [Citation53].
Lining the epithelial surface, the peri-ciliary layer (PCL) is cooperating with ciliated cells and the mucus and is required for effective mucociliary clearance. PCL is considered as a nearly Newtonian fluid with low viscosity that allows cilia to freely beat, propelling the overlying mucus layer [Citation56]. PCL is composed of membrane-spanning mucins and mucopolysaccharides that are tethered to cilia, microvilli, and epithelial surface, controlling moisture between the epithelium and the mucus. PCL structure exhibited pore size with lower (~20–40 nm) size than in the mucus layer. It acts as a permeable barrier preventing the penetration of 40 nm beads or even smaller infectious agents [Citation57]. No direct evidence has been provided so far on the impact of the diffusion of inhaled Abs.
The complex physical and chemical features of airway surface liquids, comprising the mucus layer and PCL, are interdependent and in constant equilibrium to guarantee a homeostatic rheology [Citation46]. In fact, in many lung diseases, some parameters are severely altered. For example, asthma, COPD, and CF are characterized by overproduction of mucus, resulting in a thicker mucus layer reaching >250 µm [Citation58]. This is accompanied by chronic inflammation that will impact mucus architecture through dehydration and increased cross-linking between mucins. This ultimately leads to increased viscosity, rigidity, and shrinkage of the pore size to be smaller than 100 nm [Citation59]. The reduced osmotic modulus of the mucus layer will also constrict the PCL, eventually stopping the mucociliary clearance [Citation56]. All these ASL abnormalities may impair Ab penetration and diffusion toward the lung epithelium, as previously described for other therapeutics [Citation60–62].
The development of strategies promoting Ab diffusion through the mucus requires an exhaustive knowledge of its structure and physicochemical properties in both physiological and pathological contexts. In fact, depending on the location of the Ab target antigen and the modifications of the mucus properties associated to the disease, it may be necessary to develop formulations to pass the mucus barrier or explore combined treatments to facilitate Ab interaction with its antigen [Citation29]. For example, the addition of anti-adhesive molecules, such as low molecular weight polyethylene glycol (PEG), may be relevant to cross the mucus barrier, as shown in vitro [Citation63] and in vivo [Citation64] for other applications.
3.2. Alveolar barrier
Deep within the lungs, the alveoli represent the largest part of the airways in contact with the external environment. It has been estimated to be ~100 m2, constantly exposed to billions of particles, whose main objective is to ensure gas exchange. To do so, the anatomical structure of the alveoli is substantially different from the central lung. Alveoli epithelium is composed of type I and II pneumocytes, covered with a thin layer of pulmonary surfactant (PS). PS is mainly composed of phospholipids (90%) in which hydrophobic acyl chains are exposed toward the air and polar headgroups are facing the aqueous phase adsorbed on pneumocytes. Thus, PS lowers the surface tension of this water layer. This will minimize the effort of breathing and avoid alveolar collapse during exhalation enabling the process of breathing. The high lipid content of PS offers a hydrophobic environment favoring solubilization of poorly water-soluble molecules. Conversely, PS cannot efficiently retain hydrophilic substances [Citation65]. Apart from lipids, PS contains proteins (~10%) including hydrophobic proteins SP-B and SP-C which are essential for the surface activity of PS and hydrophilic proteins SP-A and SP-D which are involved in innate immunity [Citation66–68]. SP-D promotes agglutination of particles in larger corpses that will be removed via the mucociliary clearance mechanism. Alveolar clearance process is dependent on the particle shape, size, and chemical composition. It involves alveolar macrophages (AM) and non-phagocytic cells (through clathrin or caveolae-mediated uptake). It plays a significant role in pulmonary clearance processes by internalizing and degrading inhaled particles with size ranging from 0.5 to 5 µm with an optimized size of 1–3 µm and MW >40 kDa [Citation69]. Smaller particles can escape AM scavenging, but they can ultimately be pinocytosed by type I pneumocyte. Large proteins like Abs may also interact with different PS components, resulting in the formation of a corona around the inhaled particle [Citation70] and promoting aggregation. SP-A and SP-D, by interacting with inhaled proteins, will lower their absorption rate in the alveolar space, promoting their phagocytosis by AM [Citation71,Citation72] mediated through binding of the Ab’s Fc domain to cell-surface Fcγ-receptor (FcγR) [Citation73]. This has been confirmed by confocal imaging and cell depletion studies, showing that AM plays a significant role in the clearance of inhaled biotherapeutics [Citation74,Citation75], including Ab [Citation16]. In addition, Abs are supposed to slowly get across the alveolar-capillary barrier (see II.5), prolonging their residency in the airways, thus giving more time to AM to phagocytose them.
The biophysical properties of PS allow it to quickly (within seconds) adsorb onto the lung air–liquid interface. Consequently, PS has been exploited for quite a long time to favor lung delivery of various drugs, especially nanomedicines. PS may represent a double-edged sword for delivering hydrophobic and hydrophilic inhaled compounds into the lung [Citation76].
Similarly, to the ASL, homeostatic PS function may be disturbed in various lung diseases by directly interacting with PS component, impairing PS production by type II cells, or by altering the alveolar-capillary membrane [Citation65]. Infections and chronic inflammation occurring during COPD and CF are, for example, associated with the alteration of type II cell function leading to the alterations of PS composition and synthesis. Endothelial and epithelial cell damage can lead to high permeability edema with leakage of plasma protein which will inactivate the PS layer [Citation77]. Immune cell homeostasis is also impaired by a decreased number of macrophages harboring altered phagocytic capacities and a recruitment of neutrophils and monocytes that will profoundly alter alveolar microenvironment [Citation78]. This will have a critical impact on the fate of inhaled biotherapeutic both directly by increasing barrier effect or indirectly by the reduction of ventilation due to alveolar collapse.
Strategies to increase lung retention, by limiting mucociliary clearance mechanisms and evading AM uptake include the addition of anti-adhesive molecules, such as PEGs. For example, PEGylation of Ab fragments (anti-IL-17 and -IL-13) markedly increased their lung residence time [Citation16]. The size-discriminating property of AM – which favors phagocytosis of particles with size ranging 0.5–5 µm [Citation79] – may be used for the development of innovative approaches for inhaled Ab. For example, carrier entrapped-particles of larger size are used to escape AM and provide longer inhaled drug release [Citation80].
3.3. Impact of proteases
Proteases are enzymes catalyzing maturation or catabolism of proteins, a process critically involved in the control of multiple biological processes. Proteases are classified into six different groups based on the specificity of their catalytic site. At steady state, the most abundant proteases found in human broncho-alveolar lavage are dipeptidyl peptidase 4, dipeptidyl carboxypeptidase I, pro-cathepsin H, and cathepsin D [Citation81]. In addition, PS is enriched in metalloproteinase (MMP) 2 and dipeptidyl carboxypeptidase I, which can alter the fate of inhaled proteins and peptides [Citation82,Citation83]. The irrevocability of their modes of action and the pleiotropic functions necessitates proteases to be tightly regulated. This is notably done thanks to anti-proteases controlling protease production, maturation, secretion, and activation [Citation84]. The neutrality of protease/anti-protease balance is a marker of the healthy lung.
It has been demonstrated that like other proteins, Ab is sensitive to proteolysis. Neutrophil-associated proteases (elastase, PR3), MMPs, or bacteria secreted proteases have the ability to cleave endogenous immunoglobulins [Citation85–88]. The proteolysis of therapeutic Abs is still under-appreciated and may represent a critical contributor to impair the therapeutic response [Citation89–94] and pharmacokinetic (PK) [Citation95] profile of inhaled Abs. This may be particularly significant in the context of pulmonary diseases, which are frequently associated with an increased expression and activity of lung proteases due to a dysregulated protease/anti-protease balance [Citation96,Citation97]. In COPD, CF, or acute respiratory distress syndrome (ARDS), the activation of resident immune cells or the massive influx of leukocytes leads to an increased activity of proteases (notably elastase and proteinase 3) [Citation98–102], which is even enhanced during infectious exacerbations, contributing to the decline of respiratory function [Citation103].
To the best of our knowledge, the inactivation of Ab by proteolysis is under-appreciated and may account as a major contributor to therapeutic response evasion. Identification of the major cleavage sites may help engineer Ab protected from proteolysis and maintain effector functions [Citation91]. The use of rescuing Ab, binding to neo-epitope generated after proteolytic cleavage, may also be considered to preserve Ab functionality [Citation104]. Combination of Ab therapy with protease inhibitors may also represent an interesting way to maintain Ab functionality in inflammatory/infectious diseases [Citation89,Citation90,Citation105–107].
3.4. Impact of bacterial biofilm
In the context of respiratory infections, the site of deposition of the inhaled drug depends on the type of microbes and its infective capacity. In the context of respiratory diseases such CF, COPD, and bronchiectasis, bacteria associated with chronic infection are usually found embedded in biofilm. A biofilm is a three-dimensional structured community of bacteria encased in self-produced extracellular polymeric substance mainly composed of exopolysaccharides, proteins, and DNA [Citation108,Citation109] proteins [Citation110,Citation111]. This mucoid phenotype confers to the bacteria the ability to adhere to both biotic and inert surfaces – including endotracheal tubes used for ventilation – and to reduce its exposition to antibiotics and immune cells [Citation112,Citation113]. The clinical importance of biofilm complexity as a pathological obstacle to antimicrobial therapeutics is now well recognized and is a direct consequence of its structural properties. Biofilm exhibits positive electrostatic charges, which will either repulse or trap negatively charged antimicrobial peptides or proteins [Citation114]. The physical hindrance formed by exopolysaccharides severely limits the engulfment by phagocytes of pathogens after their recognition by an Ab – a phenomenon referred as opsonophagocytosis –, thereby reducing bacterial clearance [Citation115]. Finally, bacteria inside a biofilm display profound metabolic modification as compared to planktonic ones. This is associated with the reduction of active cellular processes, including cell wall formation or the expression of virulence factors, which have may represent potent therapeutic targets for Ab promoting lung microbial resilience [Citation116].
Strategies improving drug penetration into the biofilm are already evaluated in early clinical stages [Citation117] and mainly focus on retained medical devices [Citation118]. Challenges associated with residual biofilms, triggering chronic infections, should consider the biofilm life cycle. Promoting mature biofilm dispersion using surfactant, DNase, or D-amino-acids have been considered with some success [Citation119–121] and may be worth testing in combination with inhaled Ab. Another strategy might rely on the use of Ab fragments, such as scFv, with smaller molecular weight, which might better penetrate into the biofilm, like they do in solid tumors [Citation122].
3.5. Interactions with the epithelium surface
Historically, inhalation has been evaluated for the local or systemic delivery of biological drugs [Citation123]. The large size and complex structure of Abs significantly decrease their ability to cross the airway epithelium to reach the systemic circulation. This probably explains the clinical failure of inhaled omalizumab as neutralizing serum IgE is critical to achieve a therapeutic response.
For locally-acting inhaled Abs, their target antigen may be located on the epithelium surface or in the parenchyma [Citation22].
3.5.1. Lung absorption
The successful landing of inhaled Abs on the airway surface is not necessarily associated with systemic biodistribution, as it involves complex absorption mechanisms through the epithelium and the endothelium [Citation124]. Inhaled Abs can be absorbed by three distinct mechanisms: paracellular diffusion via tight junctions, transcellular diffusion via vesicular endocytosis or pinocytosis, and receptor-dependent transcytosis [Citation125,Citation126]. The molecule size is one of the most important parameters dictating the rate of absorption across the epithelium [Citation127–129]. In fact, the systemic bioavailability of inhaled biologics decreased proportionally with their molecular mass [Citation130]. Inhaled proteins with MW < 40 kDa cross quickly the alveolar-capillary barrier [Citation18,Citation131,Citation132], while full-length Ab [Citation15,Citation133] or Ab fragments [Citation25,Citation54] exhibited limited bioavailability. Paracellular permeability allows high-capacity transport of molecule with molecular mass <600 Da and size in the range of 8–9 Å in diameter [Citation134]. Due to their high MW, inhaled full-length Abs – and even smaller fragments – transportation by paracellular diffusion is estimated negligible [Citation54]. They preferentially seem to exploit transcellular passage [Citation135] to reach the systemic compartment. Transcellular transport of inhaled full-length, e.g. containing a Fc-domain, occurs using the neonatal Fc receptor (FcRn) (see section II.5.2). Other molecular features, including pH, electrical charge, surface activity, and solubility/stability of the inhaled antibody, will influence its bioavailability [Citation6]. Eventually, in pathological conditions, alterations of the alveolar-capillary barrier may induce transient leakage allowing less restrictive and size-independent transportation [Citation136,Citation137].
3.5.2. The role of FcRn
Described in 1964 by Brambell et al., FcRn is a histocompatibility complex (MHC) class I-related intracellular receptor expressed in the cellular early endosomes [Citation138,Citation139], and transiently on the cell surface during endosome fusion to the plasma membrane. In the respiratory tract, FcRn is expressed by bronchial cells and alveolar epithelial cells (in some species) [Citation140–143] and in the nasal epithelium [Citation144]. Originally described as a receptor mediating IgG transcytosis from mother to fetus, FcRn is also recognized as one of the main parameters dictating PK of therapeutic Ab, prolonging their half-life in blood and tissues. In fact, pinocytosed Ab by lung epithelial cells will bind to FcRn, in acidic (pH ~6.0–6.5) early endosomes, via its interaction with amino-acid residues located in the CH2-CH3 hinge region of the Ab [Citation145–147]. Endosomes are then shuttled toward the plasma membrane where Ab-FcRn complexes dissociate at physiological pH, liberating the Ab in the environment [Citation138]. This mechanism confers protection to Ab from degradation in the lysosome. In the lungs, it will maintain homeostatic Ab levels [Citation148], allow Ab transcytosis across the mucosal surface [Citation140] and contribute to Ab biodistribution via recycling [Citation15,Citation149]. The efficiency of FcRn-mediated Ab salvation pathway is somehow limited, it will concern approximately two-third of the Ab uptake in the endosome [Citation150,Citation151] with saturating recycling capacity especially in the presence of a high exogenous concentration of Abs [Citation152].
Interestingly, pathological modulation of FcRn expression will impact Ab’s PK/PD. For example, polymorphism-increasing levels of FcRn transcripts is associated with improved bioavailability of therapeutic Abs [Citation153]. In the lung, FcRn expression is restricted to the bronchial epithelium as well as alveolar macrophages in humans and non-human primates [Citation15,Citation140]. Thus, FcRn expression or the quantity of cells expressing FcRn may be particularly sensitive to lung inflammation and infection. For instance, FcRn expression has been shown to be decreased in the tumor tissue [Citation154], but the impact of this reduction on the intra-tumoral distribution of Abs has not been investigated yet. A study reported that the lung residence time of airway-delivered Cetuximab (chimeric IgG1) was 10 times shorter in FcRn-knockout (KO) animals, as compared to naive mice [Citation15], in agreement with the role of FcRn in Ab half-life. However, the (systemic) bioavailability of cetuximab was higher in FcRn-KO animals, suggesting that other mechanisms may account for the passage of inhaled Ab from the airways into the systemic circulation.
3.5.3. The role of FcγRs
The binding of inhaled Abs to FcγRs, expressed at the surface of many immune cells – including AM – can promote Ab clearance through receptor-mediated endocytosis. Ab-FcγR complex will be internalized, promoting its intracellular catabolism. However, this process does not seem to be a significant barrier to Ab absorption as experiments using FcγR knockout animals showed a marginal impact on Ab catabolism [Citation155]. In specific circumstances, Ab binding to FcγR may limit its bioavailability, when considering (i) phagocyte infiltration due to inflammatory or infectious triggers which will increase the net amount of FcγR molecule, (ii) larger Ab scaffold, like antibody-drug-conjugate, or Fc-mutant Ab with improved affinity to FcγR [Citation156].
4. Conclusion
The recent Sars-CoV-2 pandemic has highlighted our lack of preparedness with limited pipeline of drugs in development, inappropriate mode of action or route of administration. This urged the development of innovative therapeutic solutions. Among them, inhaled Abs were particularly attractive. Treating respiratory diseases by inhaled Ab seems obvious when considering the importance of matching the delivery of Ab in the vicinity of their target. In fact, multiple preclinical and early clinical evidence have shown the relevance of inhalation to improve the therapeutic index of Abs to treat respiratory diseases. However, there are many parameters to integrate during inhaled Ab drug development, such as barriers, to achieve clinical success and pave the way for inhaled Abs.
5. Expert opinion
For the treatment of respiratory diseases, locoregional delivery of Ab appears to be the most relevant, and accumulating evidence has shown a better therapeutic index of Abs delivered locally into the lungs. However, multiple questions remain to be addressed to understand better the pharmacology of inhaled Abs, at the basic research levels, and considered for further clinical investigations to support the development of successful inhaled Ab therapy.
Additional lung pharmacokinetic/pharmacodynamic (PK/PD) studies are necessary to better characterize the fate of pulmonary delivered Ab. PK studies are mandatory during drug development to transpose preclinical results to humans, helping to determine the best schedule and regimen for Ab, while predicting the clinical outcome. Very few studies have assessed Ab fate after local administration [Citation152], and modeling the Ab behavior in the lungs from data obtained systemically may not be the most appropriate considering the insufficient understanding of the molecular processes involved in Ab lung absorption. Moreover, the important biological/physiological and anatomical differences of animal species with humans make difficult the translation of PK results. Selecting the most appropriate animal species and computing relevant mathematical models would be valuable to properly evaluate inhaled Ab PK parameters. While rodents are commonly used for preclinical PK studies, they did not provide predictive results for aerosol deposition in humans, due to strong anatomical/physiological differences. PK studies in larger animals may be helpful for clinical transposition. Finally, it is noteworthy that PK studies are usually done in healthy animals, but pathological conditions modifying lung homeostasis (mucus hypersecretion, protease-inhibitor imbalance, etc.) may dramatically change PK parameters of inhaled Abs.
Abs have witnessed notable advances, revolutionizing the treatment of various lung diseases. However, as reported in this review, several challenges remain to ensure their successful delivery directly into the lungs. In fact, maintaining the stability of inhaled Ab is of paramount importance. In this review, we have highlighted the challenges related to denaturation and aggregation which may arise during the process of aerosolization and cause a loss of pharmacological activity and/or immunogenicity. From a regulatory point-of-view, a high-quality Ab product should be free from visible aggregates and contain low levels of particles above 10 µm and 25 µm [Citation157]. These recommendations from the European pharmacopoeia apply to parenteral Ab products, but there are no guidelines for inhaled Ab, yet. So far, only a few studies have investigated the local effects of Ab aggregates on lung physiology. We have recently shown that Ab aggregates generated by aerosolization may be of particular concern as they induce lung cytotoxicity and inflammation [Citation45]. Additional experiments will be required to analyze the impact of the type of nebulizer and Ab on the induction of local and systemic immunogenicity. Besides, immunogenicity ultimately leads to the generation of ADA that may accelerate Ab clearance and hinder its efficacy. Regulatory agencies recommend monitoring Ab immunogenicity during product development, in animals, if it is relevant, and in humans. On several occasions, Ab immunogenicity has been conducted to terminate the development of efficacious Ab [Citation158]. Further development of delivery devices specialized for Ab-based biopharmaceuticals is needed to address these challenges.
Mitigation strategies to limit immunogenicity include optimization of Ab’s formulation. In fact, pharmaceutical development of a high-quality product must guarantee an efficacious and safe treatment along all life of the product [Citation159]. Classically, parenteral Ab formulations are designed to provide protection against temperature changes, light exposure, or shearing/shaking associated to transport and injection device [Citation159]. Such formulations have been shown to be suitable for mesh nebulization of therapeutic proteins in the lungs while limiting Ab aggregation [Citation17,Citation160]. However, recent findings demonstrated that these repurposed formulations cannot be invariably transposed to inhaled Ab [Citation31]. Further studies are mandatory to select appropriate dose and excipients to stabilize Ab during nebulization. This must be done in the context of a limited range of FDA-approved excipients for lung administration and the potential impact of excipients on formulation properties (viscosity, charge, etc.), and eventually device performances [Citation161]. Thus, the identification of lung compatible excipients is important for the safe development of inhaled Abs.
On the one hand, many works have focused, over the past decade, on the impact of device technology, formulation, and Ab engineering to minimize Ab degradation and allow reproducible deposition in the targeted area of the lung. Although broad principles have been demonstrated (e.g. protection by surfactants, etc.), each Ab acts differently during aerosolization. On the other hand, few studies have considered the biological barriers encountered by the Ab after deposition into the respiratory tract, which may lead to Ab degradation and limit their access to biological targets. Ab-based biotherapeutics, which are in development of inhalation, will likely build upon the learnings from evidence described in this review. Considering the type of molecule, its target, and the disease context, each barrier may need to be considered from the early beginning in the development of new inhaled Ab to avoid failure during clinical development. Improving our understanding of the barrier significance as impediments is paramount to achieve the desired treatment paradigm. In the future, various mitigation strategies should be investigated to overcome biological barriers. This includes surface modifications to improve Ab diffusion and deposition in pathological mucus or surfactant, advanced formulations ensuring Ab protection from degradation mediated by endogenous protease and co-treatment (mucolytics, biofilm disrupting agents) to facilitate Ab access to its target antigen.
Moreover, further clinical evaluation is essential to establish meaningful evidence for the therapeutic superiority and safety profile of inhalation of biopharmaceuticals. In fact, recent clinical trial failures also point out the necessity to define better the disease/population, the target, and the drug and the device that may all together really benefit patients. Indeed, ALX-0171 was recently evaluated in a Phase 2 trial in children with Respiratory Syncytial Virus (RSV) infections in Japan by inhalation through a mesh-nebulizer connected to a face mask. Although, it has proven safe and has reached the primary endpoint, i.e. a rapid drop of nasal viral load below the limit of detection, it failed to affect disease trajectory in severe patients [Citation30]. Improvement in clinical evaluation of inhaled Ab may require a better definition of the primary endpoints, using composite mortality and clinical parameters, and the inclusion of a larger number of patients.
Overall, inhaled Ab has a tremendous opportunity to benefit patients with respiratory diseases on their own or in combination with/support to existing therapeutics to enhance their efficacy. Although many hurdles remain to overcome, as described in this review, the growing interest of both the scientific and clinical communities might accelerate the progress of the scientific knowledge on therapeutic Ab inhalation.
Article highlights
Inhaled Ab therapy has been shown to be success in the treatment of respiratory disease in several preclinical models and early clinical trials.
Stresses associated with formulation and aerosolization processes may impair inhaled Ab activity.
The lungs itself comprise several biological barriers hindering Ab deposition and efficacy.
Some lung diseases (CF etc.) are associated with biological barrier changes that should be considered for the use of inhaled Ab.
It is important to integrate the challenges of Ab inhalation early during drug development for program success.
Declaration of interest
N Heuze-Vourc’h was co-founder and scientific expert for Cynbiose Respiratory. In the past 3 years, she received personal consultancy/expertise fees from the European agency/commission, Novartis, Immune Biosolutions, the National Research Agency (ANR), and Aleva/Telis Bioscience. The laboratory receives research support from Sanofi, CSL Behring, and Oxyvie and receipt of equipment from Aerogen Ltd. The authors have no other relevant affiliations or financial involvement with any organization or entity with a financial interest in or financial conflict with the subject matter or materials discussed in the manuscript apart from those disclosed.
Reviewer disclosures
Peer reviewers on this manuscript have no relevant financial or other relationships to disclose.
Acknowledgments
The figures were created with Biorender.com
Additional information
Funding
References
- Kaplon H, Crescioli S, Chenoweth A, et al. Antibodies to watch in 2023. MAbs. 2023 Jan;15(1):2153410. doi: 10.1080/19420862.2022.2153410
- The Antibody Society. 2023 May 01. Available from: https://www.antibodysociety.org/resources/approved-antibodies/
- Vidarsson G, Dekkers G, Rispens T. IgG subclasses and allotypes: from structure to effector functions. Front Immunol. 2014;5:520. doi: 10.3389/fimmu.2014.00520
- Beck A, Haeuw JF, Wurch T, et al. The next generation of antibody-drug conjugates comes of age. Discov Med. 2010 Oct;10(53):329–339.
- Elshiaty M, Schindler H, Christopoulos P. Principles and Current clinical Landscape of Multispecific antibodies against cancer. Int J Mol Sci. 2021 May 26;22(11):5632. doi: 10.3390/ijms22115632
- Ryman JT, Meibohm B. Pharmacokinetics of monoclonal antibodies. CPT Pharmacometrics Syst Pharmacol. 2017 Sep;6(9):576–588. doi: 10.1002/psp4.12224
- Alagga AA, Gupta V. Drug absorption. StatPearls. 2023. Treasure Island (FL). https://www.ncbi.nlm.nih.gov/books/NBK557405/
- Agusti A, Vogelmeier CF, Halpin DMG. Tackling the global burden of lung disease through prevention and early diagnosis. Lancet Respir Med. 2022 Nov;10(11):1013–1015. doi: 10.1016/S2213-2600(22)00302-2
- Tsai CW, Morris S. Approval of Raxibacumab for the treatment of inhalation anthrax under the US Food and drug administration “animal Rule”. Front Microbiol. 2015;6:1320. doi: 10.3389/fmicb.2015.01320
- Chiang DT, Clark J, Casale TB. Omalizumab in asthma: approval and postapproval experience. Clin Rev Allergy Immunol. 2005 Aug;29(1):3–16. doi: 10.1385/CRIAI:29:1:003
- Pai-Scherf L, Blumenthal GM, Li H, et al. FDA approval summary: pembrolizumab for treatment of metastatic non-small cell lung cancer: first-line therapy and beyond. Oncology. 2017 Nov;22(11):1392–1399. doi: 10.1634/theoncologist.2017-0078
- Borghardt JM, Kloft C, Sharma A. Inhaled therapy in respiratory disease: The complex Interplay of pulmonary Kinetic processes. Can Respir J. 2018;2018:2732017. doi: 10.1155/2018/2732017
- Rau JL. The inhalation of drugs: advantages and problems. Respir Care. 2005 Mar;50(3):367–382.
- Herve V, Rabbe N, Guilleminault L, et al. VEGF neutralizing aerosol therapy in primary pulmonary adenocarcinoma with K-ras activating-mutations. MAbs. 2014;6(6):1638–1648. doi: 10.4161/mabs.34454
- Guilleminault L, Azzopardi N, Arnoult C, et al. Fate of inhaled monoclonal antibodies after the deposition of aerosolized particles in the respiratory system. J Control Release. 2014 Dec 28;196:344–354. doi: 10.1016/j.jconrel.2014.10.003
- Koussoroplis SJ, Paulissen G, Tyteca D, et al. Pegylation of antibody fragments greatly increases their local residence time following delivery to the respiratory tract. J Control Release. 2014 Aug 10;187:91–100. doi: 10.1016/j.jconrel.2014.05.021
- Respaud R, Vecellio L, Diot P, et al. Nebulization as a delivery method for mAbs in respiratory diseases. Expert Opin Drug Deliv. 2015 Jun;12(6):1027–1039. doi: 10.1517/17425247.2015.999039
- Patton JS, Fishburn CS, Weers JG. The lungs as a portal of entry for systemic drug delivery. Proc Am Thorac Soc. 2004;1(4):338–344. doi: 10.1513/pats.200409-049TA
- de Boer AH, Gjaltema D, Hagedoorn P, et al. Characterization of inhalation aerosols: a critical evaluation of cascade impactor analysis and laser diffraction technique. Int J Pharm. 2002 Dec 5;249(1–2):219–231. doi: 10.1016/S0378-5173(02)00526-4
- Heyder J. Deposition of inhaled particles in the human respiratory tract and consequences for regional targeting in respiratory drug delivery. Proc Am Thorac Soc. 2004;1(4):315–320. doi: 10.1513/pats.200409-046TA
- Loira-Pastoriza C, Todoroff J, Vanbever R. Delivery strategies for sustained drug release in the lungs. Adv Drug Deliv Rev. 2014 Aug;75:81–91. doi: 10.1016/j.addr.2014.05.017
- Ferrati S, Wu T, Kanapuram SR, et al. Dosing considerations for inhaled biologics. Int J Pharm. 2018 Oct 5;549(1–2):58–66. doi: 10.1016/j.ijpharm.2018.07.054
- Rohrer J, Lupo N, Bernkop-Schnurch A. Advanced formulations for intranasal delivery of biologics. Int J Pharm. 2018 Dec 20;553(1–2):8–20. doi: 10.1016/j.ijpharm.2018.10.029
- Shepard KB, Vodak DT, Kuehl PJ, et al. Local treatment of non-small cell lung cancer with a spray-dried Bevacizumab formulation. AAPS Pharm Sci Tech. 2021 Aug 31;22(7):230. doi: 10.1208/s12249-021-02095-7
- Burgess G, Boyce M, Jones M, et al. Randomized study of the safety and pharmacodynamics of inhaled interleukin-13 monoclonal antibody fragment VR942. EBioMedicine. 2018 Sep;35:67–75.
- Pan HW, Seow HC, JCK L, et al. Spray-dried and spray-freeze-dried powder formulations of an anti-interleukin-4Ralpha antibody for pulmonary delivery. Pharm Res. 2022 Sep;39(9):2291–2304. doi: 10.1007/s11095-022-03331-w
- Rajapaksa A, Qi A, Yeo LY, et al. Enabling practical surface acoustic wave nebulizer drug delivery via amplitude modulation. Lab Chip. 2014 Jun 7;14(11):1858–1865. doi: 10.1039/C4LC00232F
- Van Heeke G, Allosery K, De Brabandere V, et al. Nanobodies(r) as inhaled biotherapeutics for lung diseases. Pharmacol Ther. 2017 Jan;169:47–56.
- Matthews AA, Ee PLR, Ge R. Developing inhaled protein therapeutics for lung diseases. Mol Biomed. 2020;1(1):11. doi: 10.1186/s43556-020-00014-z
- Cunningham S, Piedra PA, Martinon-Torres F, et al. Nebulised ALX-0171 for respiratory syncytial virus lower respiratory tract infection in hospitalised children: a double-blind, randomised, placebo-controlled, phase 2b trial. Lancet Respir Med. 2021 Jan 01;9(1):21–32.
- Mayor A, Thibert B, Huille S, et al. Inhaled antibodies: formulations require specific development to overcome instability due to nebulization. Drug Deliv Transl Res. 2021 Aug;11(4):1625–1633. doi: 10.1007/s13346-021-00967-w
- Depreter F, Pilcer G, Amighi K. Inhaled proteins: challenges and perspectives. Int J Pharm. 2013 Apr 15;447(1–2):251–280. doi: 10.1016/j.ijpharm.2013.02.031
- Pilcer G, Amighi K. Formulation strategy and use of excipients in pulmonary drug delivery. Int J Pharm. 2010 Jun 15;392(1–2):1–19. doi: 10.1016/j.ijpharm.2010.03.017
- Hickey AJ, Stewart IE. Inhaled antibodies: Quality and performance considerations. Human Vaccines Immunother. 2022 Apr 29;18(2):1940650. doi: 10.1080/21645515.2021.1940650
- Chen L, Okuda T, Lu XY, et al. Amorphous powders for inhalation drug delivery. Adv Drug Deliv Rev. 2016 May 1;100:102–115. doi: 10.1016/j.addr.2016.01.002
- Son YJ, Miller DP, Weers JG. Optimizing spray-dried Porous particles for high dose delivery with a Portable dry powder inhaler. Pharmaceutics. 2021 Sep 21;13(9):1528. doi: 10.3390/pharmaceutics13091528
- Mayor A, Thibert B, Huille S, et al. Inhaled IgG1 antibodies: The buffering system is an important driver of stability during mesh-nebulization. Eur J Pharm Biopharm. 2022 Dec;181:173–182.
- Respaud R, Marchand D, Pelat T, et al. Development of a drug delivery system for efficient alveolar delivery of a neutralizing monoclonal antibody to treat pulmonary intoxication to ricin. J Control Release. 2016 Jul 28;234:21–32. doi: 10.1016/j.jconrel.2016.05.018
- Respaud R, Marchand D, Parent C, et al. Effect of formulation on the stability and aerosol performance of a nebulized antibody. MAbs. 2014;6(5):1347–1355. doi: 10.4161/mabs.29938
- Le Basle Y, Chennell P, Tokhadze N, et al. Physicochemical stability of monoclonal antibodies: A review. J Pharm Sci. 2020 Jan;109(1):169–190. doi: 10.1016/j.xphs.2019.08.009
- Burkitt W, Domann P, O’Connor G. Conformational changes in oxidatively stressed monoclonal antibodies studied by hydrogen exchange mass spectrometry. Protein Sci. 2010 Apr;19(4):826–835. doi: 10.1002/pro.362
- Bodier-Montagutelli E, Respaud R, Perret G, et al. Protein stability during nebulization: Mind the collection step! Eur J Pharm Biopharm. 2020 Jul;152:23–34.
- Ponce R, Abad L, Amaravadi L, et al. Immunogenicity of biologically-derived therapeutics: assessment and interpretation of nonclinical safety studies. Regul Toxicol Pharmacol. 2009 Jul;54(2):164–182. doi: 10.1016/j.yrtph.2009.03.012
- Krishna M, Nadler SG. Immunogenicity to biotherapeutics - the role of anti-drug immune Complexes. Front Immunol. 2016;7:21. doi: 10.3389/fimmu.2016.00021
- Secher T, Bodier-Montagutelli E, Parent C, et al. Aggregates associated with instability of antibodies during aerosolization induce adverse immunological effects. Pharmaceutics. 2022 Mar 18;14(3):671. doi: 10.3390/pharmaceutics14030671
- Leal J, Smyth HDC, Ghosh D. Physicochemical properties of mucus and their impact on transmucosal drug delivery. Int J Pharm. 2017 Oct 30;532(1):555–572. doi: 10.1016/j.ijpharm.2017.09.018
- Lai SK, Wang YY, Wirtz D, et al. Micro- and macrorheology of mucus. Adv Drug Deliv Rev. 2009 Feb 27;61(2):86–100. doi: 10.1016/j.addr.2008.09.012
- Thornton DJ, Rousseau K, McGuckin MA. Structure and function of the polymeric mucins in airways mucus. Annu Rev Physiol. 2008;70(1):459–486. doi: 10.1146/annurev.physiol.70.113006.100702
- Creeth JM. Constituents of mucus and their separation. Br Med Bull. 1978 Jan;34(1):17–24. doi: 10.1093/oxfordjournals.bmb.a071454
- Lieleg O, Ribbeck K. Biological hydrogels as selective diffusion barriers. Trends Cell Biol. 2011 Sep;21(9):543–551. doi: 10.1016/j.tcb.2011.06.002
- Rose MC, Voynow JA. Respiratory tract mucin genes and mucin glycoproteins in health and disease. Physiol Rev. 2006 Jan;86(1):245–278. doi: 10.1152/physrev.00010.2005
- Sanders N, Rudolph C, Braeckmans K, et al. Extracellular barriers in respiratory gene therapy. Adv Drug Deliv Rev. 2009 Feb 27;61(2):115–127. doi: 10.1016/j.addr.2008.09.011
- Olmsted SS, Padgett JL, Yudin AI, et al. Diffusion of macromolecules and virus-like particles in human cervical mucus. Biophys J. 2001 Oct;81(4):1930–1937. doi: 10.1016/S0006-3495(01)75844-4
- Patil HP, Freches D, Karmani L, et al. Fate of PEGylated antibody fragments following delivery to the lungs: Influence of delivery site, PEG size and lung inflammation. J Control Release. 2018 Feb 28;272:62–71. doi: 10.1016/j.jconrel.2017.12.009
- Cone RA. Barrier properties of mucus. Adv Drug Deliv Rev. 2009 Feb 27;61(2):75–85. doi: 10.1016/j.addr.2008.09.008
- Boucher RC. Airway surface dehydration in cystic fibrosis: pathogenesis and therapy. Annu Rev Med. 2007;58:157–170. doi: 10.1146/annurev.med.58.071905.105316
- Button B, Cai LH, Ehre C, et al. A periciliary brush promotes the lung health by separating the mucus layer from airway epithelia. Science. 2012 Aug 24;337(6097):937–941. doi: 10.1126/science.1223012
- Roy I, Vij N. Nanodelivery in airway diseases: challenges and therapeutic applications. Nanomedicine. 2010 Apr;6(2):237–244. doi: 10.1016/j.nano.2009.07.001
- Duncan GA, Jung J, Joseph A, et al. Microstructural alterations of sputum in cystic fibrosis lung disease. JCI Insight. 2016 Nov 3;1(18):e88198. doi: 10.1172/jci.insight.88198
- Braeckmans K, Peeters L, Sanders NN, et al. Three-dimensional fluorescence recovery after photobleaching with the confocal scanning laser microscope. Biophys J. 2003 Oct;85(4):2240–2252. doi: 10.1016/S0006-3495(03)74649-9
- Ensign LM, Tang BC, Wang YY, et al. Mucus-penetrating nanoparticles for vaginal drug delivery protect against herpes simplex virus. Sci Transl Med. 2012 Jun 13;4(138):138ra79. doi: 10.1126/scitranslmed.3003453
- Maisel K, Reddy M, Xu Q, et al. Nanoparticles coated with high molecular weight PEG penetrate mucus and provide uniform vaginal and colorectal distribution in vivo. Nanomedicine (Lond). 2016 Jun;11(11):1337–1343. doi: 10.2217/nnm-2016-0047
- Lai SK, O’Hanlon DE, Harrold S, et al. Rapid transport of large polymeric nanoparticles in fresh undiluted human mucus. Proc Natl Acad Sci U S A. 2007 Jan 30;104(5):1482–1487. doi: 10.1073/pnas.0608611104
- Muralidharan P, Mallory E, Malapit M, et al. Inhalable PEGylated phospholipid nanocarriers and PEGylated therapeutics for respiratory delivery as aerosolized colloidal dispersions and dry powder inhalers. Pharmaceutics. 2014 Jun 20;6(2):333–353. doi: 10.3390/pharmaceutics6020333
- Haitsma JJ, Lachmann U, Lachmann B. Exogenous surfactant as a drug delivery agent. Adv Drug Deliv Rev. 2001 Apr 25;47(2–3):197–207. doi: 10.1016/S0169-409X(01)00106-5
- Lopez-Rodriguez E, Perez-Gil J. Structure-function relationships in pulmonary surfactant membranes: from biophysics to therapy. Biochim Biophys Acta. 2014 Jun;1838(6):1568–1585. doi: 10.1016/j.bbamem.2014.01.028
- Guagliardo R, Perez-Gil J, De Smedt S, et al. Pulmonary surfactant and drug delivery: focusing on the role of surfactant proteins. J Control Release. 2018 Dec 10;291:116–126. doi: 10.1016/j.jconrel.2018.10.012
- Castillo-Sanchez JC, Cruz A, Perez-Gil J. Structural hallmarks of lung surfactant: Lipid-protein interactions, membrane structure and future challenges. Arch Biochem Biophys. 2021 May 30;703:108850. doi: 10.1016/j.abb.2021.108850
- Fernandes CA, Vanbever R. Preclinical models for pulmonary drug delivery. Expert Opin Drug Deliv. 2009 Nov;6(11):1231–1245. doi: 10.1517/17425240903241788
- Mandal T, Konduru NV, Ramazani A, et al. Effect of surface charge and hydrophobicity on phospholipid-nanoparticle corona formation: a molecular dynamics simulation study. Coll Inter Sci Commun. 2018 Jun 01;25:7–11.
- Ruge CA, Schaefer UF, Herrmann J, et al. The interplay of lung surfactant proteins and lipids assimilates the macrophage clearance of nanoparticles. PLoS One. 2012;7(7):e40775. doi: 10.1371/journal.pone.0040775
- Todoroff J, Vanbever R. Fate of nanomedicines in the lungs. Curr Opin Colloid Interface Sci. 2011 Jun 01;16(3):246–254.
- Wang W, Wang EQ, Balthasar JP. Monoclonal antibody pharmacokinetics and pharmacodynamics. Clin Pharmacol Ther. 2008 Nov;84(5):548–558. doi: 10.1038/clpt.2008.170
- Ducreux J, Vanbever R. Crucial biopharmaceutical issues facing macromolecular candidates for inhalation: the role of macrophages in pulmonary protein clearance. Respir Drug Deliv Eur. 2007;2007:31–41.
- Lombry C, Edwards DA, Preat V, et al. Alveolar macrophages are a primary barrier to pulmonary absorption of macromolecules. Am J Physiol Lung Cell Mol Physiol. 2004 May;286(5):L1002–8. doi: 10.1152/ajplung.00260.2003
- Hidalgo A, Cruz A, Perez-Gil J. Barrier or carrier? Pulmonary surfactant and drug delivery. Eur J Pharm Biopharm. 2015 Sep;95(Pt A):117–127. doi: 10.1016/j.ejpb.2015.02.014
- Lachmann B, Eijking EP, So KL, et al. In vivo evaluation of the inhibitory capacity of human plasma on exogenous surfactant function. Intensive care Med. 1994;20(1):6–11. doi: 10.1007/BF02425047
- Michelson P, Faro A, Ferkol T, et al. 51 - pulmonary disease in cystic fibrosis. In: Wilmott R, Deterding R Li A, editors. Kendig’s Disorders of the Respiratory Tract in Children. Ninth. Philadelphia: Elsevier; 2019. p. 777–787.e4. 10.1016/B978-0-323-44887-1.00051-1
- Geiser M. Update on macrophage clearance of inhaled micro- and nanoparticles. J Aerosol Med Pulm Drug Deliv. 2010 Aug;23(4):207–217. doi: 10.1089/jamp.2009.0797
- Tsapis N, Bennett D, Jackson B, et al. Trojan particles: large porous carriers of nanoparticles for drug delivery. Proc Natl Acad Sci U S A. 2002 Sep 17;99(19):12001–12005. doi: 10.1073/pnas.182233999
- Woods A, Andrian T, Sharp G, et al. Development of new in vitro models of lung protease activity for investigating stability of inhaled biological therapies and drug delivery systems. Eur J Pharm Biopharm. 2020 Jan;146:64–72.
- Candiano G, Bruschi M, Pedemonte N, et al. Proteomic analysis of the airway surface liquid: modulation by proinflammatory cytokines. Am J Physiol Lung Cell Mol Physiol. 2007 Jan;292(1):L185–98. doi: 10.1152/ajplung.00085.2006
- Nagae A, Abe M, Becker RP, et al. High concentration of carboxypeptidase M in lungs: presence of the enzyme in alveolar type I cells. Am J Respir Cell Mol Biol. 1993 Aug;9(2):221–229. doi: 10.1165/ajrcmb/9.2.221
- Vasiljeva O, Menendez E, Nguyen M, et al. Monitoring protease activity in biological tissues using antibody prodrugs as sensing probes. Sci Rep. 2020 Apr 3;10(1):5894. doi: 10.1038/s41598-020-62339-7
- Dunnhaupt S, Kammona O, Waldner C, et al. Nano-carrier systems: Strategies to overcome the mucus gel barrier. Eur J Pharm Biopharm. 2015 Oct;96:447–453.
- von Pawel-Rammingen U, Johansson BP, Bjorck L. IdeS, a novel streptococcal cysteine proteinase with unique specificity for immunoglobulin G. EMBO J. 2002 Apr 2;21(7):1607–1615. doi: 10.1093/emboj/21.7.1607
- Brezski RJ, Jordan RE. Cleavage of IgGs by proteases associated with invasive diseases: an evasion tactic against host immunity? MAbs. 2010 May;2(3):212–220. doi: 10.4161/mabs.2.3.11780
- Deveuve Q, Lajoie L, Barrault B, et al. The proteolytic cleavage of therapeutic monoclonal antibody hinge region: more than a matter of subclass. Front Immunol. 2020;11:168. doi: 10.3389/fimmu.2020.00168
- Biancheri P, Brezski RJ, Di Sabatino A, et al. Proteolytic cleavage and loss of function of biologic agents that neutralize tumor necrosis factor in the mucosa of patients with inflammatory bowel disease. Gastroenterology. 2015 Nov;149(6):1564–1574 e3. doi: 10.1053/j.gastro.2015.07.002
- Curciarello R, Sobande T, Jones S, et al. Human Neutrophil elastase proteolytic activity in ulcerative colitis favors the loss of function of therapeutic monoclonal antibodies. J Inflamm Res. 2020;13:233–243. doi: 10.2147/JIR.S234710
- Kinder M, Greenplate AR, Grugan KD, et al. Engineered protease-resistant antibodies with selectable cell-killing functions. J Biol Chem. 2013 Oct 25;288(43):30843–30854. doi: 10.1074/jbc.M113.486142
- Hsiao HC, Fan X, Jordan RE, et al. Proteolytic single hinge cleavage of pertuzumab impairs its Fc effector function and antitumor activity in vitro and in vivo. Breast Cancer Res. 2018 Jun 1;20(1):43. doi: 10.1186/s13058-018-0972-4
- Fan X, Brezski RJ, Fa M, et al. A single proteolytic cleavage within the lower hinge of trastuzumab reduces immune effector function and in vivo efficacy. Breast Cancer Res. 2012 Aug 8;14(4):R116. doi: 10.1186/bcr3240
- Zhang N, Deng H, Fan X, et al. Dysfunctional antibodies in the tumor microenvironment associate with impaired anticancer immunity. Clin Cancer Res. 2015 Dec 1;21(23):5380–5390. doi: 10.1158/1078-0432.CCR-15-1057
- Agu RU, Ugwoke MI, Armand M, et al. The lung as a route for systemic delivery of therapeutic proteins and peptides. Respir Res. 2001;2(4):198–209. doi: 10.1186/rr58
- Youn YS, Kwon MJ, Na DH, et al. Improved intrapulmonary delivery of site-specific PEGylated salmon calcitonin: optimization by PEG size selection. J Control Release. 2008 Jan 4;125(1):68–75. doi: 10.1016/j.jconrel.2007.10.008
- Al Ojaimi Y, Blin T, Lamamy J, et al. Therapeutic antibodies – natural and pathological barriers and strategies to overcome them. Pharmacol Ther. 2021 Oct 20;233:108022. doi: 10.1016/j.pharmthera.2021.108022
- Hashimoto S, Okayama Y, Shime N, et al. Neutrophil elastase activity in acute lung injury and respiratory distress syndrome. Respirology. 2008 Jun;13(4):581–584. doi: 10.1111/j.1440-1843.2008.01283.x
- Pandey KC, De S, Mishra PK. Role of proteases in chronic obstructive pulmonary disease. Front Pharmacol. 2017;8:512. doi: 10.3389/fphar.2017.00512
- Thulborn SJ, Mistry V, Brightling CE, et al. Neutrophil elastase as a biomarker for bacterial infection in COPD. Respir Res. 2019 Jul 30;20(1):170. doi: 10.1186/s12931-019-1145-4
- Witko-Sarsat V, Halbwachs-Mecarelli L, Schuster A, et al. Proteinase 3, a potent secretagogue in airways, is present in cystic fibrosis sputum. Am J Respir Cell Mol Biol. 1999 Apr;20(4):729–736. doi: 10.1165/ajrcmb.20.4.3371
- Oriano M, Terranova L, Sotgiu G, et al. Evaluation of active neutrophil elastase in sputum of bronchiectasis and cystic fibrosis patients: A comparison among different techniques. Pulm Pharmacol Ther. 2019 Dec;59:101856.
- Chalmers JD, Moffitt KL, Suarez-Cuartin G, et al. Neutrophil elastase activity is associated with exacerbations and lung function decline in bronchiectasis. Am J Respir Crit Care Med. 2017 May 15;195(10):1384–1393. doi: 10.1164/rccm.201605-1027OC
- Fan X, Brezski RJ, Deng H, et al. A novel therapeutic strategy to rescue the immune effector function of proteolytically inactivated cancer therapeutic antibodies. Mol Cancer Ther. 2015 Mar;14(3):681–691. doi: 10.1158/1535-7163.MCT-14-0715
- Amancha KP, Hussain A. Effect of protease inhibitors on pulmonary bioavailability of therapeutic proteins and peptides in the rat. Eur J Pharm Sci. 2015 Feb 20;68:1–10. doi: 10.1016/j.ejps.2014.11.008
- Park SH, Kwon JH, Lim SH, et al. Characterization of human insulin microcrystals and their absorption enhancement by protease inhibitors in rat lungs. Int J Pharm. 2007 Jul 18;339(1–2):205–212. doi: 10.1016/j.ijpharm.2007.03.003
- Hussain A, Arnold JJ, Khan MA, et al. Absorption enhancers in pulmonary protein delivery. J Control Release. 2004 Jan 8;94(1):15–24. doi: 10.1016/j.jconrel.2003.10.001
- Montanaro L, Poggi A, Visai L, et al. Extracellular DNA in biofilms. Int J Artif Organs. 2011 Sep;34(9):824–831. doi: 10.5301/ijao.5000051
- Ciornei CD, Novikov A, Beloin C, et al. Biofilm-forming Pseudomonas aeruginosa bacteria undergo lipopolysaccharide structural modifications and induce enhanced inflammatory cytokine response in human monocytes. Innate Immun. 2010 Oct;16(5):288–301. doi: 10.1177/1753425909341807
- Fong JC, Karplus K, Schoolnik GK, et al. Identification and characterization of RbmA, a novel protein required for the development of rugose colony morphology and biofilm structure in Vibrio cholerae. J Bacteriol. 2006 Feb;188(3):1049–1059. doi: 10.1128/JB.188.3.1049-1059.2006
- Tielker D, Hacker S, Loris R, et al. Pseudomonas aeruginosa lectin LecB is located in the outer membrane and is involved in biofilm formation. Microbiology (Reading). 2005 May;151(Pt 5):1313–1323. doi: 10.1099/mic.0.27701-0
- Meluleni GJ, Grout M, Evans DJ, et al. Mucoid Pseudomonas aeruginosa growing in a biofilm in vitro are killed by opsonic antibodies to the mucoid exopolysaccharide capsule but not by antibodies produced during chronic lung infection in cystic fibrosis patients. J Immunol. 1995 Aug 15;155(4):2029–2038. doi: 10.4049/jimmunol.155.4.2029
- Costerton JW, Stewart PS, Greenberg EP. Bacterial biofilms: a common cause of persistent infections. Science. 1999 May 21;284(5418):1318–1322. doi: 10.1126/science.284.5418.1318
- Anaya-Lopez JL, Lopez-Meza JE, Ochoa-Zarzosa A. Bacterial resistance to cationic antimicrobial peptides. Crit Rev Microbiol. 2013 May;39(2):180–195. doi: 10.3109/1040841X.2012.699025
- Campoccia D, Mirzaei R, Montanaro L, et al. Hijacking of immune defences by biofilms: a multifront strategy. Biofouling. 2019 Nov;35(10):1055–1074. doi: 10.1080/08927014.2019.1689964
- Koo H, Allan RN, Howlin RP, et al. Targeting microbial biofilms: current and prospective therapeutic strategies. Nat Rev Microbiol. 2017 Dec;15(12):740–755. doi: 10.1038/nrmicro.2017.99
- Bjarnsholt T, Ciofu O, Molin S, et al. Applying insights from biofilm biology to drug development - can a new approach be developed? Nat Rev Drug Discov. 2013 Oct;12(10):791–808. doi: 10.1038/nrd4000
- Zander ZK, Becker ML. Antimicrobial and Antifouling strategies for polymeric medical devices. ACS Macro Lett. 2018 Jan 16;7(1):16–25. doi: 10.1021/acsmacrolett.7b00879
- Kolodkin-Gal I, Romero D, Cao S, et al. D-amino acids trigger biofilm disassembly. Science. 2010 Apr 30;328(5978):627–629. doi: 10.1126/science.1188628
- Chang RYK, Li M, Chow MYT, et al. A dual action of D-amino acids on anti-biofilm activity and moisture-protection of inhalable ciprofloxacin powders. Eur J Pharm Biopharm. 2022 Apr;173:132–140.
- Louis M, Clamens T, Tahrioui A, et al. Pseudomonas aeruginosa biofilm dispersion by the human atrial natriuretic peptide. Adv Sci. 2022 Mar;9(7):e2103262. doi: 10.1002/advs.202103262
- Thurber GM, Schmidt MM, Wittrup KD. Antibody tumor penetration: transport opposed by systemic and antigen-mediated clearance. Adv Drug Deliv Rev. 2008 Sep;60(12):1421–1434. doi: 10.1016/j.addr.2008.04.012
- Liang W, Pan HW, Vllasaliu D, et al. Pulmonary delivery of biological drugs. Pharmaceutics. 2020 Oct 26;12(11):1025. doi: 10.3390/pharmaceutics12111025
- Glassman PM, Abuqayyas L, Balthasar JP. Assessments of antibody biodistribution. J Clin Pharmacol. 2015 Mar;55(3):S29–38. doi: 10.1002/jcph.365
- Niven RW. Delivery of biotherapeutics by inhalation aerosol. Crit Rev Ther Drug Carrier Syst. 1995;12(2–3):151–231. doi: 10.1615/CritRevTherDrugCarrierSyst.v12.i2-3.20
- Wolff RK. Safety of inhaled proteins for therapeutic use. J Aerosol Med. 1998 Winter;11(4):197–219. doi: 10.1089/jam.1998.11.197
- Folkesson HG, Westrom BR, Karlsson BW. Permeability of the respiratory tract to different-sized macromolecules after intratracheal instillation in young and adult rats. Acta Physiol Scand. 1990 Jun;139(2):347–354. doi: 10.1111/j.1748-1716.1990.tb08933.x
- Conhaim RL, Watson KE, Lai-Fook SJ, et al. Transport properties of alveolar epithelium measured by molecular hetastarch absorption in isolated rat lungs. J Appl Physiol. 2001 Oct;91(4):1730–1740. doi: 10.1152/jappl.2001.91.4.1730
- Holter JF, Weiland JE, Pacht ER, et al. Protein permeability in the adult respiratory distress syndrome. Loss of size selectivity of the alveolar epithelium. J Clin Invest. 1986 Dec;78(6):1513–1522. doi: 10.1172/JCI112743
- Patton JS, Trinchero P, Platz RM. Bioavailability of pulmonary delivered peptides and proteins: α-interferon, calcitonins and parathyroid hormones. JControlled Release. 1994 Jan 01;28(1):79–85.
- Patton JS, Byron PR. Inhaling medicines: delivering drugs to the body through the lungs. Nat Rev Drug Discov. 2007 Jan;6(1):67–74. doi: 10.1038/nrd2153
- Labiris NR, Dolovich MB. Pulmonary drug delivery. Part I: physiological factors affecting therapeutic effectiveness of aerosolized medications. Br J Clin Pharmacol. 2003 Dec;56(6):588–599. doi: 10.1046/j.1365-2125.2003.01892.x
- Maillet A, Guilleminault L, Lemarie E, et al. The airways, a novel route for delivering monoclonal antibodies to treat lung tumors. Pharm Res. 2011 Sep;28(9):2147–2156. doi: 10.1007/s11095-011-0442-5
- Watson CJ, Rowland M, Warhurst G. Functional modeling of tight junctions in intestinal cell monolayers using polyethylene glycol oligomers. Am J Physiol Cell Physiol. 2001 Aug;281(2):C388–97. doi: 10.1152/ajpcell.2001.281.2.C388
- Kim KJ, Malik AB. Protein transport across the lung epithelial barrier. Am J Physiol Lung Cell Mol Physiol. 2003 Feb;284(2):L247–59. doi: 10.1152/ajplung.00235.2002
- Turner JR. Intestinal mucosal barrier function in health and disease. Nat Rev Immunol. 2009 Nov;9(11):799–809. doi: 10.1038/nri2653
- Buschmann MM, Shen L, Rajapakse H, et al. Occludin OCEL-domain interactions are required for maintenance and regulation of the tight junction barrier to macromolecular flux. Mol Biol Cell. 2013 Oct;24(19):3056–3068. doi: 10.1091/mbc.e12-09-0688
- Ober RJ, Martinez C, Vaccaro C, et al. Visualizing the site and dynamics of IgG salvage by the MHC class I-related receptor, FcRn. J Immunol. 2004 Feb 15;172(4):2021–2029. doi: 10.4049/jimmunol.172.4.2021
- Antohe F, Radulescu L, Gafencu A, et al. Expression of functionally active FcRn and the differentiated bidirectional transport of IgG in human placental endothelial cells. Hum Immunol. 2001 Feb;62(2):93–105. doi: 10.1016/S0198-8859(00)00244-5
- Spiekermann GM, Finn PW, Ward ES, et al. Receptor-mediated immunoglobulin G transport across mucosal barriers in adult life: functional expression of FcRn in the mammalian lung. J Exp Med. 2002 Aug 5;196(3):303–310. doi: 10.1084/jem.20020400
- Sakagami M, Omidi Y, Campbell L, et al. Expression and transport functionality of FcRn within rat alveolar epithelium: a study in primary cell culture and in the isolated perfused lung. Pharm Res. 2006 Feb;23(2):270–279. doi: 10.1007/s11095-005-9226-0
- Akilesh S, Christianson GJ, Roopenian DC, et al. Neonatal FcR expression in bone marrow-derived cells functions to protect serum IgG from catabolism. J Immunol. 2007 Oct 1;179(7):4580–4588. doi: 10.4049/jimmunol.179.7.4580
- Latvala S, Jacobsen B, Otteneder MB, et al. Distribution of FcRn across species and tissues. J Histochem Cytochem. 2017 Jun;65(6):321–333. doi: 10.1369/0022155417705095
- Campanari ML, Bourefis AR, Kabashi E. Diagnostic challenge and Neuromuscular Junction Contribution to ALS Pathogenesis. Front Neurol. 2019;10:68. doi: 10.3389/fneur.2019.00068
- Raghavan M, Bonagura VR, Morrison SL, et al. Analysis of the pH dependence of the neonatal Fc receptor/immunoglobulin G interaction using antibody and receptor variants. Biochemistry. 1995 Nov 14;34(45):14649–14657. doi: 10.1021/bi00045a005
- Kim JK, Firan M, Radu CG, et al. Mapping the site on human IgG for binding of the MHC class I-related receptor, FcRn. Eur J Immunol. 1999 Sep;29(9):2819–2825. doi: 10.1002/(SICI)1521-4141(199909)29:09<2819:AID-IMMU2819>3.0.CO;2-6
- Oganesyan V, Damschroder MM, Cook KE, et al. Structural insights into neonatal Fc receptor-based recycling mechanisms. J Biol Chem. 2014 Mar 14;289(11):7812–7824. doi: 10.1074/jbc.M113.537563
- Vogelzang A, Lozza L, Reece ST, et al. Neonatal Fc receptor Regulation of lung immunoglobulin and CD103+ Dendritic cells confers Transient Susceptibility to Tuberculosis. Infect Immun. 2016 Oct;84(10):2914–2921. doi: 10.1128/IAI.00533-16
- Bequignon E, Dhommee C, Angely C, et al. FcRn-dependent transcytosis of monoclonal antibody in human nasal epithelial cells in vitro: A Prerequisite for a New delivery route for therapy? Int J Mol Sci. 2019 Mar 19;20(6):1379. doi: 10.3390/ijms20061379
- Kim J, Hayton WL, Robinson JM, et al. Kinetics of FcRn-mediated recycling of IgG and albumin in human: pathophysiology and therapeutic implications using a simplified mechanism-based model. Clin Immunol. 2007 Feb;122(2):146–155. doi: 10.1016/j.clim.2006.09.001
- Junghans RP, Anderson CL. The protection receptor for IgG catabolism is the beta2-microglobulin-containing neonatal intestinal transport receptor. Proc Natl Acad Sci U S A. 1996 May 28;93(11):5512–5516. doi: 10.1073/pnas.93.11.5512
- Guillon A, Pardessus J, Lhommet P, et al. Exploring the fate of inhaled monoclonal antibody in the lung parenchyma by microdialysis. MAbs. 2019 Feb;11(2):297–304. doi: 10.1080/19420862.2018.1556081
- Passot C, Azzopardi N, Renault S, et al. Influence of FCGRT gene polymorphisms on pharmacokinetics of therapeutic antibodies. MAbs. 2013 Jul;5(4):614–619. doi: 10.4161/mabs.24815
- Dalloneau E, Baroukh N, Mavridis K, et al. Downregulation of the neonatal Fc receptor expression in non-small cell lung cancer tissue is associated with a poor prognosis. Oncotarget. 2016 Aug 23;7(34):54415–54429. doi: 10.18632/oncotarget.10074
- Abuqayyas L, Balthasar JP. Application of knockout mouse models to investigate the influence of FcgammaR on the tissue distribution and elimination of 8C2, a murine IgG1 monoclonal antibody. Int J Pharm. 2012 Dec 15;439(1–2):8–16. doi: 10.1016/j.ijpharm.2012.09.042
- Lux A, Yu X, Scanlan CN, et al. Impact of immune complex size and glycosylation on IgG binding to human FcgammaRs. J Immunol. 2013 Apr 15;190(8):4315–4323. doi: 10.4049/jimmunol.1200501
- Council of Europe. Particulate contamination: sub-visible particles – PDG revision of general chapter released for public consultation in: healthcare EDftQoMa, editor. https://www.edqm.eu/en/-/2.9.19.-particulate-contamination-sub-visible-particles-pdg-revision-of-general-chapter-released-for-public-consultation-22019
- FDA. Immunogenicity assessment for therapeutic protein products 2014. Available from: https://www.fda.gov/regulatory-information/search-fda-guidance-documents/immunogenicity-assessment-therapeutic-protein-products
- Falconer RJ. Advances in liquid formulations of parenteral therapeutic proteins. Biotechnol Adv. 2019 Nov 15;37(7):107412. doi: 10.1016/j.biotechadv.2019.06.011
- Bodier-Montagutelli E, Mayor A, Vecellio L, et al. Designing inhaled protein therapeutics for topical lung delivery: what are the next steps? Expert Opin Drug Deliv. 2018 Aug;15(8):729–736. doi: 10.1080/17425247.2018.1503251
- Yildiz-Pekoz A, Ehrhardt C. Advances in pulmonary drug delivery. Pharmaceutics. 2020 Sep 23;12(10):911. doi: 10.3390/pharmaceutics12100911