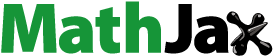
ABSTRACT
This study examined the protective effect of Zn on salt-stressed Brassica juncea plants using some key morphological and biochemical attributes at different developmental stages (30, 60, and 90 days after treatment [DAT]). Salt stress (200 mM) caused suppression in plant height, root length, and dry weight by 58.35%, 41.15%, and 53.33%, respectively, at 90 DAT, but Zn application improved these variables by 15.52%, 16.59%, and 11.45%, respectively. Furthermore, 200 mM NaCl decreased total chlorophyll by 45.32% and relative water content by 27.62% at 90 DAT, whereas Zn application compensated the decrease in the levels of both variables. NaCl (200 mM) increased H2O2, malondialdehyde, and electrolyte leakage by 70.48%, 35.25%, and 68.39%, respectively, at 90 DAT, but Zn supplementation appreciably reduced these variables. Except for catalase, enzymatic antioxidant activity increased under NaCl stress. Zn application with salt further increased the activities of superoxide dismutase, catalase, ascorbate peroxidase, glutathione reductase, and glutathione-S-transferase by 33.51%, 9.21%, 10.98%, 17.46%, and 12.87%, respectively, at 90 DAT. At 90 DAT, salt stress increased flavonoids by 24.88%, and Zn supply by a further 7.68%. Overall, Zn mitigated the adverse effects of salt stress through osmotic adjustment, as well as by modulating the oxidative defense system and flavonoid contents.
1. Introduction
Salinity is a major environmental cue, which considerably suppresses crop production worldwide through its injurious effects on plant growth and development (Ashraf and McNeilly Citation2004; Ahanger and Agarwal Citation2017). Globally, high salinity affects over 20% of arable land; it exacerbates industrialization and urbanization in most parts of the world. However, poor irrigation practices are further aggravating the problem (Gupta and Huang Citation2014). Salinity stress hampers plant growth via diminishing cell division and expansion, reducing photosynthetic efficiency, modifying metabolic processes, as well as causing ion toxicity, osmotic stress, oxidative stress, genotoxicity, and other physiological disorders (Ibrahim et al. Citation2012; Wani et al. Citation2013; Yan et al. Citation2013; Ahmad et al. Citation2016b; Anjum et al. Citation2015; Hussein et al. Citation2017). Cumulatively, these adverse effects perturb growth and alter normal metabolism in plants (Wani et al. Citation2013; Rasool et al. Citation2013; Ahmad et al. Citation2014; Iqbal et al. Citation2015; Tang et al. Citation2015; Hussein et al. Citation2017).
Salinity stress induces the excessive generation of reactive oxygen species (ROS), which can cause cell death through causing damages to proteins, lipids, and DNA (Ahmad et al. Citation2010; Gill and Tuteja Citation2010; Anjum et al. Citation2015). Plants possess endogenous oxidative defense mechanisms (enzymatic and non-enzymatic antioxidant) to counteract salinity-induced ROS. ROS scavengers include superoxide dismutase (SOD), catalase (CAT), ascorbate peroxidase (APX), glutathione reductase (GR), and glutathione-S-transferase (GST), whereas non-enzymatic components primarily include ascorbic acid (ASA) and glutathione (GSH), both low-molecular-weight metabolites (Ahmad et al. Citation2010; Gill and Tuteja Citation2010). Plant flavonoid content is also believed to confer stress tolerance (Ahmad et al. Citation2016a). Moreover, up-regulated osmolytes’ (e.g. proline, glycine betaine, and soluble sugars) synthesis and accumulation protect proteins, enzymes, and other biologically important molecules (Hayat et al. Citation2012; Ahanger and Agarwal Citation2017). These osmotically active solutes act as antioxidants and contribute to water content maintenance for optimal cellular functioning (Ahanger et al. Citation2014, Citation2015; Anjum et al. Citation2014).
Several chemical, biological, and physical strategies are being adopted to obtain a maximum economic yield from crops. For example, supplementation of mineral macro- and micro-nutrients at optimal concentrations allays adverse effects of stressors, leading to growth maintenance and yield increases (Ahanger et al. Citation2015; Ahmad et al. Citation2015b; Siddiqui et al. Citation2015; Ahanger and Agarwal Citation2017). Among microelements, Zn is vital for regulating enzyme activity, the protein and membrane stabilization; it is a component of Zn finger, a major structural motif critical for DNA binding (Tavallali et al. Citation2009). Zinc is a cofactor of over 300 enzymes and associated proteins involved in carbohydrate metabolism, nucleic acid and protein synthesis, as well as cell division (Osredkar and Sustar Citation2011; Singh et al. Citation2015). Moreover, Zn can impart stress tolerance through the formation of Cu/Zn-SOD, a major antioxidant (Ahmad et al. Citation2010).
Brassica juncea (L.) Czern & Coss (family Brassicaceae) is an important oil-seed crop that often experiences salinity stress because of its vast cultivation in arid and semi-arid regions (Wani et al. Citation2013). Addition of Zn salts directly to soil solution has been demonstrated to improve crop growth and ameliorate stress in B. juncea (Prasad and Saradhi Citation1995). However, only a few reports exist in the literature on how foliar application of Zn may prevent stress-induced oxidative damage through regulating antioxidative and osmoregulatory components. Thus, in this study, we have tried to explore if an exogenous application of Zn to the foliage of salt-stressed B. juncea plants could effectively regulate the oxidative defense system and the levels of osmotically active metabolites.
2. Materials and methods
2.1. Plant materials and growth conditions
B. juncea (L.) Czern. & Coss. seeds of varuna cultivar were sown in Petri dishes and placed in a growth chamber. Five germinated seedlings were shifted to each pot (5 kg capacity with 23-cm diameter) containing peat, perlite, and sand (1:1:1, v/v/v) and the pots were supplied with Hoagland nutrient solution (200 mL per pot) for 2 weeks under average day/night temperatures of 26°C/16°C. After this thinning was done manually and 3 seedlings were allowed to grow in each pot. After 18 days, varying NaCl concentrations (0, 100, and 200 mM) dissolved in Hoagland nutrient solution were applied on alternate days to all pots except the control till 90 days after treatment (DAT). The composition of Hoagland solution is given below: (mg L−1): 270 N (KNO3), 31 P (KH2PO4), 234 K (KNO3), 200 Ca (Ca(NO3)2·4H2O), 64 S (MgSO4·7H2O), 48 Mg (MgSO4·7H2O), 2.8 Fe (Fe-EDTA), 0.5 Mn (MnCl2·4H2O), 0.5 B (H3BO3), 0.02 Cu (CuSO4), 0.05 Zn (ZnSO4·7H2O), and 0.01 Mo (H3MoO4·H2O) (Hoagland and Arnon Citation1940). The pH of nutrient solution was adjusted to 6.5 with 0.1 mM KOH.
Zinc (ZnSO4·7H2O, 1 mM) dissolved in distilled water was sprayed on plant foliage after every 2 days with a manual sprayer (10 mL/plant), from day 7 (25-day-old plant) to day 90 (115-day-old plant) of NaCl treatment. The experimental design was completely randomized with five replicates. Leaf samples (secondary leaves) were collected for analysis after salt treatment for 30 (55-day-old plant), 60 (85-day-old plant), and 90 (115-day-old plant) days.
2.2. Growth and biomass
The length of shoot and root was measured manually with a scale. The samples were dried at 65°C for 72 h for the assessment of dry biomass expressed in grams per plant.
2.3. Estimation of chlorophyll content
Chlorophyll pigments were estimated following Hiscox and Israelstam (Citation1979). The tissue samples were extracted from fresh leaves in dimethyl sulfoxide (DMSO); the absorbance of the resultant supernatant was measured spectrophotometrically at 645 and 663 nm (Beckman 640 D, USA), using DMSO as a blank.
2.4. Estimation of leaf relative water content
Leaf discs were punched from the samples of each treatment and their fresh weight (FW) determined. The discs were floated on water for up to 4 h to record turgid weight. Subsequently, the discs were oven-dried at 85°C to estimate dry weight (DW) (Smart and Bingham Citation1974). Leaf relative water content (LRWC) was calculated as follows:(1)
(1)
2.5. Determination of electrolyte leakage
Electrolyte leakage in leaf tissues was determined following Dionisio-Sese and Tobita (Citation1998). The leaf discs were kept in 10 mL deionized water and electrical conductivity observed at time point 0 (EC0). The leaf discs were heated in a water bath at 60°C for 25 min and then measured EC1. Thereafter, the samples were boiled at 100°C for 10 min for EC2 measurement. The formula used to calculate the electrolyte leakage was:
2.6. Determination of leaf free proline content
Proline content was determined following Bates et al. (Citation1973). The absorbance of the sample mixture treated appropriately with specific reagents was recorded spectrophotometrically at 520 nm (Beckman 640 D, USA), using toluene as a blank.
2.7. Estimation of H2O2 and malondialdehyde
Hydrogen peroxide content was determined following Velikova et al. (Citation2000). Fresh leaves (500 mg) were homogenized in 5 mL of 0.1% (w/v) trichloroacetic acid (TCA). The homogenate was centrifuged at 12,000g for 15 min; thereafter, 0.5 mL of the supernatant was mixed with 0.5 mL of 10 mM potassium phosphate buffer (pH 7.0) and 1.0 mL of 1.0 M potassium iodide solution. The supernatant absorbance was read at 390 nm.
To determine lipid peroxidation (a measure of malondialdehyde [MDA] formation), fresh leaf tissue was first macerated in 1% TCA and then centrifuged at 10,000g for 5 min. The supernatant (1.0 mL) was reacted with 4.0 mL of 0.5% thiobarbituric acid for 30 min in a water bath at 95°C, cooled in an ice-bath, and centrifuged again at 10,000 rpm for 5 min. The optical density of the samples was recorded at A532. Unspecific turbidity was corrected via subtracting the optical density at 600 nm from the A532 reading. An extinction coefficient of 155 mM−1 cm−1 (Heath and Packer Citation1968) was used to calculate MDA concentration.
2.8. Antioxidant enzyme assay
Fresh leaves (10 g each) were homogenized in a buffer of 100 mM Tris-HCl (pH 7.5) containing 5.0 mM DTT, 10 mM MgCl2, 1.0 mM EDTA, 5.0 mM magnesium acetate, 1.5% PVP, 1.0 mM PMSF, and 1 μg/mL aproptinin. The extract was centrifuged for 15 min at 10,000 rpm to obtain the supernatant for enzyme assays. Two millimolar ascorbate was added to the buffer for extracting APX. Soluble protein content was determined following Bradford (Citation1976), using bovine serum albumin as the standard.
SOD (EC 1.15.1.1) activity was determined following van Rossum et al. (Citation1997), after an initial photoreduction of nitroblue tetrazolium (NBT) at 560 nm. One SOD unit was defined as the amount of protein that caused a 50% decrease in SOD-inhibitable NBT reduction and was expressed as Unit mg−1 protein.
Catalase (EC 1.11.1.6) activity was assayed by monitoring the decrease in absorbance at 240 nm for 3 min (Luck Citation1971) and was expressed as EU mg−1 protein.
APX (EC 1.11.1.11) activity was spectrophotometrically assayed following an absorbance decrease at 265 nm (Nakano and Asada Citation1981) and was expressed as Unit mg−1 protein.
After initial NADPH oxidation, GR (EC 1.6.4.2) activity was determined by monitoring an absorbance decrease at 340 nm for 2 min (Foyer and Halliwell Citation1976) and was expressed as μmol NADPH oxidized min−1 (Units mg−1 protein).
Fresh leaves (0.5 g each) were homogenized in 3.0 mL meta-phosphoric acid (5%) containing 1.0 mM EDTA; the homogenate was centrifuged at 11,500g and 4°C for 15 min. The resultant supernatant was used for ASA and GSH determination, following Huang et al. (Citation2005) and Anderson (Citation1985), respectively. Calculations were performed with ASA and GSH standards.
GST (EC 2.5.1.18) activity was estimated by the method of Hasanuzzaman and Fujita (Citation2013). The absorbance was read at 340 nm for 1 min using a spectrophotometer (Beckman 640 D, USA) and was expressed as EU mg−1 protein.
2.9. Estimation of total flavonoid content
Flavonoid content was determined using the colorimetric method (Zhishen et al. Citation1999). Absorbance was read at 510 nm and flavonoid content expressed as mg catechin equivalents g−1 of extract (mg g−1).
2.10. Statistical analysis
Data were presented as mean ± SE of five replicates and subjected to one-way analysis of variance (ANOVA), followed by Duncan's multiple range test for examining the differences among the values. Significance was set at P ≤ 0.05.
3. Results
3.1. Plant growth and biomass yield
depicts the combined effects of NaCl and Zn on plant growth and biomass yield. Compared with control, heights of plants treated with 100 mM NaCl decreased by 13.18%, 17.85%, and 21.75% at 30, 60, and 90 DAT, respectively. The treatment with 200 mM NaCl decreased plant height by 25.75%, 39.68%, and 58.35% compared with the control heights at 30, 60, and 90 DAT, respectively. Compared to plants subjected to 200 mM NaCl, Zn foliar supplementation (200 mM NaCl + Zn) enhanced plant height by 11.71%, 14.38%, and 15.52% at 30, 60, and 90 DAT, respectively.
Table 1. Effect of Zn (1 mM) on growth and biomass yield in mustard plants under NaCl stress.
Root length decreased in salt-stressed (200 mM NaCl) plants compared with the control plants maximally by 31.71%, 39.85%, and 41.15% at 30, 50, and 90 DAT, respectively (). However, Zn supplementation (200 mM NaCl + Zn) enhanced root lengths by 18.73% (30 DAT), 18.01% (60 DAT), and 16.59% (90 DAT) over with the plants treated with NaCl alone.
Shoot DW decreased under NaCl concentrations. Under 200 mM of NaCl, the maximum decrease of 47.18%, 52.82%, and 53.33% (from control were observed) at 30, 60, and 90 DAT, respectively. Compared with the salt-stressed plants, Zn supplementation increased shoot DWs by 13.02%, 12.56%, and 11.45% at 30, 60, and 90 DAT, respectively ().
3.2. Total chlorophyll content and LRWC
At 90 DAT, total chlorophyll content decreased with respect to the control by 38.06% and 45.32% in plants treated with 100 and 200 mM NaCl, respectively (). Compared with these plants, Zn supplementation increased total chlorophyll content by 10.94% (100 mM NaCl + Zn) and 8.13% (200 mM NaCl + Zn) at 90 DAT.
Table 2. Effect of Zn (1 mM) on chlorophyll, relative water content of mustard plants under NaCl stress.
At 30, 60, and 90 DAT, relative water content (RWC) of plants under 200-mM-NaCl stress increased by 40.40%, 36.06%, and 27.62%, respectively, over the control (). Under 200 mM NaCl + Zn, RWC increased by 16.61%, 14.39%, and 11.83% at 30, 60, and 90 DAT, respectively, with respect to the salt-stressed plants.
3.3. Electrolyte leakage and proline content
presents data on how electrolyte leakage and proline content were affected by NaCl and Zn application. Under 100 mM NaCl, electrolyte leakage decreased by 49.78% (30 DAT), 51.21% (60 DAT), and 53.11% (90 DAT). Under 200 mM NaCl, maximum increases of 65.08% (30 DAT), 66.61% (60 DAT), and 68.39% (90 DAT) were recorded with respect to the control. Compared with the 200 mM NaCl group, Zn application (200 mM NaCl + Zn) decreased electrolyte leakage by 29.31%, 27.43%, and 22.97% at 30, 60, and 90 DAT, respectively.
Table 3. Effect of Zn (1 mM) on electrolyte leakage and proline content of mustard plants under NaCl stress.
Under 100 mM NaCl, proline content increased over the control by 41.06%, 50.43%, and 57.82% at 30, 60, and 90 DAT, respectively (). Under 200 mM NaCl, the increase went up to 52.08%, 59.61%, and 65.42% at 30, 60, and 90 DAT, respectively. A further increase of 7.75%, 16.14%, and again 16.14% was recorded at 30, 60, and 90 DAT, respectively, due to Zn supplementation ().
3.4. H2O2 accumulation and MDA content
Under 200 mM NaCl, H2O2 accumulation increased over controls by 69.57% (30 DAT), 69.29% (60 DAT), and 70.48% (90 DAT). However, with Zn supplementation, H2O2 concentrations significantly decreased compared with those in salt-stressed plants ((A)). Specifically, 47.18% (30 DAT), 52.06% (60 DAT), and 53.00% (90 DAT) decrease was observed with 100 mM NaCl + Zn treatments, whereas 41.14% (30 DAT), 51.29% (60 DAT), and 48.41% (90 DAT) decrease with 200 mM NaCl + Zn ((A)).
Figure 1. Effect of different concentrations of NaCl in the presence and absence of Zn (1 mM) on H2O2 (A) and malondialdehyde (MDA) content (B) in Brassica juncea plants. Different letters indicate significant difference (P < 0.05) among the treatments within a developmental stage. Symbols $, £ and ¥ denote significant effects of different developmental stages within a same treatment.
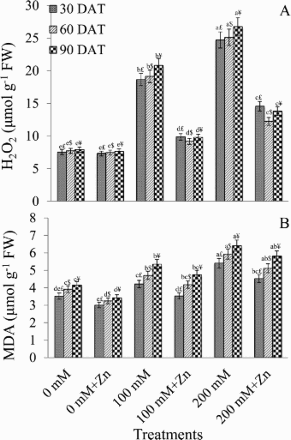
MDA content reflects the degree of lipid peroxidation and is widely accepted as an indicator of oxidative damage. Leaf MDA was higher in salt-stressed seedlings than in the control plants ((B)). Under 100 mM NaCl, MDA content increased by 16.38% (30 DAT), 16.77% (60 DAT), and 22.42% (90 DAT), whereas under 200 mM NaCl, the increase was 34.93% (30 DAT), 33.89% (60 DAT), and 35.25% (90 DAT). In general, Zn application decreased MDA content in salt-treated plants compared with that in salt-treated plants ((B)).
3.5. Antioxidant in NaCl-treated plants
presents enzymatic antioxidant activity under NaCl and Zn treatments. Both NaCl treatments increased SOD activity over the control with a maximal increase of 22.53%, 23.52%, and 28.97% at 30, 60, and 90 DAT, respectively, under 200 mM NaCl ((A)). Zinc application further increased SOD activity in salt-stressed plants by 19.87% (100 mM NaCl + Zn) and 33.51% (200 mM NaCl + Zn).
Figure 2. Effect of different concentrations of NaCl in the presence and absence of Zn (1 mM) on activities of superoxide dismutase (SOD) (A), catalase (CAT) (B), ascorbate peroxidase (APX) (C), and glutathione reductase (GR) (D) in Brassica juncea plants. Different letters indicate significant difference (P < 0.05) among the treatments within a developmental stage. Symbols $, £ and ¥ denote significant effects of different developmental stages within a same treatment.
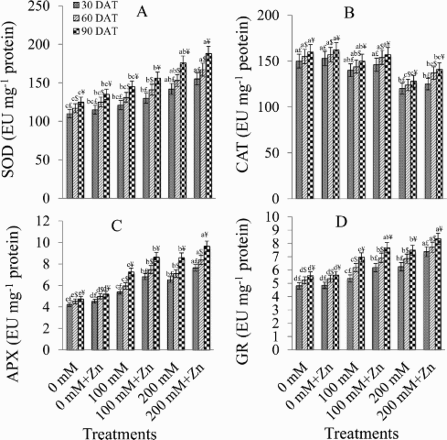
Increasing NaCl concentrations consistently decreased CAT activity. A 20% decrease with reference to the control was observed in all the three samples ((B)). However, Zn supplementation enhanced the CAT activity over that in salt-stressed plants, by 4% at 30 DAT, 9.48% at 60 DAT, and 9.21% at 90 DAT.
Salt stress (100 mM) enhanced the APX activity relative to control by 22.18% (30 DAT), 24.03% (60 DAT), and 34.48% (90 DAT) ((C)). The higher salt stress (200 mM) had a stronger effect, elevating the APX activity by 35.72% (30 DAT), 36.33% (60 DAT), and 44.70% (90 DAT). Zinc application further enhanced the APX activity by 15.99% (with 100 mM NaCl + Zn) and 10.98% (with 200 mM NaCl + Zn) in relation to salt-stressed plants, as observed at 90 DAT.
At 90 DAT, the GR activity under 100 and 200 mM NaCl increased significantly over the control by plants maximally by 19.71% and 25.50%, respectively ((D)). Application of Zn further increased the GR activity by 15.31% (30 DAT), 11.02% (60 DAT), and 10.29% (90 DAT) over that in the 200 mM NaCl-treated plants.
Similarly, under 200 mM NaCl, the GST activity increased with respect to the control by 47.07%, 46.87%, and 46.65% at 30, 60, and 90 DAT, respectively ((E)). Compared with these plants, Zn supplementation (200 mM NaCl + Zn) further enhanced the GST activity by 14.76% (30 DAT), 12.33% (60 DAT), and 12.87% (90 DAT).
Zinc application increased the ASA content by 13.79% and end with relative to the salt-stressed plants. depicts the impact of NaCl and Zn on ASA and GSH levels in B. juncea seedlings. Under 200 mM NaCl stress, ASA declined relative to the control levels by 47.91%, 47.85%, and 46.93% at 30, 60, and 90 DAT, respectively. Under 200 mM NaCl + Zn, ASA content increased by 13.79% (30 DAT), 14.71% (60 DAT), and 17.46% (90 DAT) relative to that in plants treated with salt only.
Figure 3. Effect of different concentrations of NaCl in the presence and absence of Zn (1 mM) on ascorbic acid (AsA) (A), glutathione (GSH) (B) and glutathione S-transferase (GST) (C) in Brassica juncea plants. Different letters indicate significant difference (P < 0.05) among the treatments within a developmental stage. Symbols $, £ and ¥ denote significant effects of different developmental stages within a same treatment.
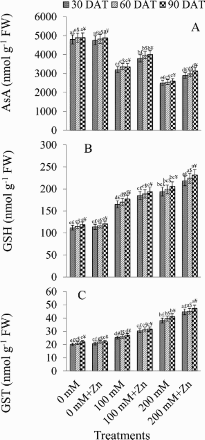
An elevation of 32.12% (30 DAT), 32.35% (60 DAT), and 32.76% (90 DAT) relative to the control was observed in the GSH content under 100 mM NaCl stress ((B)). The increase was greater, i.e. 42.24% (30 DAT), 42.21% (60 DAT), and 42.23% (90 DAT) under 200 mM NaCl. Zn supplementation (200 mM NaCl + Zn) caused a further increase of 11.00% (30 DAT), 11.16% (60 DAT), and 11.20% (90 DAT) over the salt-stressed plants.
3.6. Flavonoid content in NaCl-treated plants supplemented with Zn
The maximum decrease in flavonoid content by 38.32% (30 DAT), 31.20% (60 DAT), and 24.88% (90 DAT) were observed under 200 mM NaCl (). With 100 mM NaCl, the decrease was 23.90% (30 DAT), 20.78% (60 DAT), and 18.06% (90 DAT). Zinc foliar application (200 mM NaCl + Zn) enhanced the flavonoid content by 6.46% (30 DAT), 9.08% (60 DAT), and 7.68% (90 DAT), compared with plants treated with 200 mM NaCl only.
Figure 4. Effect of different concentrations of NaCl in presence and absence of Zn (1 mM) on total flavonoid content in Brassica juncea plants. Different letters indicate significant difference (P < 0.05) among the treatments within a developmental stage. Symbols $, £ and ¥ denote significant effects of different developmental stages within a same treatment.
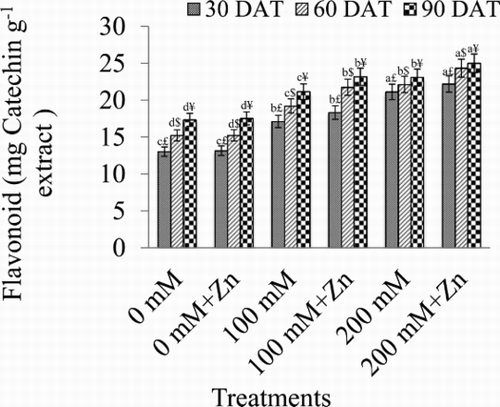
4. Discussion
In the present study, salt stress caused a decline in plant growth through reducing plant height, root length, and shoot DW, which substantiates some earlier reports on different plant species (Husen et al. Citation2016). The negative effects of NaCl on growth and biomass yield might have been due to osmotic stress and low uptake of essential elements (Hashem et al. Citation2014). In the present investigation, it is amply clear that Zn foliar sprays allayed NaCl stress effects in B. juncea. Weisany et al. (Citation2014) reported that Zn application reduces salt-stress damage considerably because of positive effects on the uptake and partitioning of important mineral elements. Our study shows that foliar spray of Zn allayed NaCl-caused effects in B. juncea. Zinc application considerably reduces the salinity-caused damage because of its positive effects on the uptake and partitioning of important mineral elements (Weisany et al. Citation2011, Citation2014). It improves plant growth by increasing the natural auxin (indole acetic acid) production and, consequently, activating the cell division and enlargement (Ali and Mahmoud Citation2013), maintaining membrane structural integrity (Weisany et al. Citation2014), accumulating phospholipids (Jiang et al. Citation2014), improving protein synthesis (Ebrahimian and Bybordi Citation2011), scavenging free oxygen radicals (Jiang et al. Citation2014), mediating nutrient translocation from developing cells (Jiang et al. Citation2014), and restricting excessive Na+ and Cl− uptake (Weisany et al. Citation2011; Siddiqui et al. Citation2015; Yousuf et al. Citation2016a, Citation2016b).
Salt stress clearly reduced total chlorophyll content of B. juncea leaves, which is in agreement with earlier findings of Weisany et al. (Citation2011), Hayat et al. (Citation2012), and Iqbal et al. (Citation2015) in different plant species. Salt stress is known to hamper the synthesis of enzymes involved in the generation and protection of photosynthetic pigments (El-Tayeb Citation2005). Destruction of these enzymes via salt stress triggers changes in the structure and stability of the pigment-protein complex, leading to chlorophyllase up-regulation and enhanced chlorophyll degradation; this process may be the cause for reduced chlorophyll content in salt-stressed plants (Fang et al. Citation1998). Additionally, Zn application may increase photosynthetic pigment synthesis through direct positive effects on the uptake of magnesium, a central chlorophyll component (Weisany et al. Citation2011, Citation2014). Our data showing chlorophyll increase with Zn application are in agreement with the findings of Samreen et al. (Citation2017) for Vigna radiata and Novo et al. (Citation2014) for B. juncea. Zinc-induced protection of sulfhydryl group may be another reason for amelioration of the drastic chlorophyll decline by salt stress (Weisany et al. Citation2011).
High concentrations of toxic ions such as Na+ in the soil solution cause osmotic stress, resulting in a reduced water uptake. In the salt-stressed plants, this lowered water uptake decreases the stomatal conductance (Tavallali et al. Citation2009). Zinc application proved beneficial in maintaining the tissue water content of B. juncea even under saline conditions. Tavallali et al. (Citation2009) demonstrated that a reduced water potential in the salt-stressed Pistacia vera led to cellular dysfunction. Our results regarding the effect of Zn application on RWC in salt-stressed plants find support from Weisany et al. (Citation2011).
In our study, application of NaCl as well as Zn increased proline accumulation. Increased proline accumulation is believed to maintain tissue water potential under environmental stresses, thus offering an important tolerance strategy (Ahanger et al. Citation2014, Citation2015; Ahmad et al. Citation2015a, Citation2015b). Salt-stressed plants maintain higher proline content through enhancing the activity of key enzymes involved in proline synthesis (Ahmad et al. Citation2016b). Proline thus protects enzymatic function and helps in free radical scavenging (Rajendrakumar et al. Citation1997). Zinc application may improve proline accumulation via regulating solute potential, and therefore water uptake from the soil.
In our study, salt stress induced H2O2 over-accumulation in B. juncea plants, but Zn application inhibited its production. Under certain threshold levels, H2O2 has a protective effect due to its role in stress-induced signaling processes. However, H2O2 overproduction causes excessive peroxidation of membrane fatty acids, protein denaturation, and negative effects on DNA/RNA integrity (Tuteja et al. Citation2009; Habibi Citation2014). The accumulation of H2O2 in salt-stressed plants may be due to reduced RWC, which limits H2O2 diffusion from its generation site (Weisany et al. Citation2014). Stress-mediated H2O2 production, subsequent membrane leakage, and MDA formation in combination determine the intensity of oxidative stress (Tavallali et al. Citation2009; Ahmad et al. Citation2014, Citation2015a). Loss of membrane integrity from increased ROS production causes leakage of important mineral ions from cellular organelles (Tuna et al. Citation2007). Specifically, membrane integrity is compromised through the generation of lipid hydroperoxides via ROS–membrane interactions (Hashem et al. Citation2014). Zinc-treated mustard seedlings exhibited reduced MDA content and increased membrane stability, confirming the positive role of Zn in avoiding ROS-induced oxidative damage under salt stress.
Salt stress enhances ROS generation, thereby increasing antioxidant enzyme activity (Tavallali et al. Citation2009; Ahmad et al. Citation2016b; Weisany et al. Citation2014). In the present study, salt stress up-regulated the activities of SOD, APX, GR, and GST. Furthermore, zinc application caused rapid ROS elimination, thereby further enhancing antioxidant enzyme activity coupled with better plant growth. Previous research supports our findings that supplementation of optimal mineral concentrations keeps ROS at nontoxic levels (Ahanger et al. Citation2015; Iqbal et al. Citation2015). SOD acts on superoxide radicals, which are further scavenged by either CAT or APX through the ascorbate–glutathione pathway; as a result, superoxide radicals are removed and prevented from forming more toxic radicals (Ahmad et al. Citation2010; Bose et al. Citation2014). Additionally, SOD prevents metal-mediated formation of toxic OH−. In the ascorbate–glutathione pathway, APX, GR, GSH, and ASA are key members that mediate removal of H2O2 through the transfer of electrons from NADPH to H2O2, with GSH and ASA acting as mobile redox buffers. Our findings suggest that Zn application elevated GSH and GR concentrations, contributing to efficient H2O2 scavenging and lower lipid peroxidation. Increased APX activity can overcome salt-stress-induced decreases in CAT activity; both molecules are active H2O2 scavengers (Ahmad et al. Citation2010; Gill and Tuteja Citation2010). Here, we demonstrated that CAT activity was decreased by salt stress, but increased by Zn supplementation, suggesting that Zn can optimize CAT activity to improve the processes of H2O2 scavenging under stress. GST mediates detoxification via conjugating xenobiotics with the non-enzymatic antioxidant, tripeptide glutathione (Ahmad et al. Citation2010; Gill and Tuteja Citation2010). Zn-induced enhancement in the GST activity can mediate quick detoxification of radicals, in addition to its role in hormone homeostasis, vacuolar sequestration of anthocyanins, cell apoptosis, and stress responses (Dixon et al. Citation2010; Gill and Tuteja Citation2010). Our results showing increased GST activity in salt-stressed B. juncea plants concur with the findings of Gapińska et al. (Citation2008) in Lycopersicon esculentum.
Flavonoids are polyphenols with low molecular weight and are vital to the protection of photosynthesizing cells. Plants maintaining higher flavonoid content show improved photosynthetic efficiency due to rapid, flavonoid-mediated scavenging of superoxide radicals (Majer et al. Citation2014). It is not clear if the Zn-induced enhancement of NaCl tolerance is related to the metal's involvement in flavonoid synthesis. However, in salt-stressed plants, flavonoids are thought to act as chelators (Winkel-Shirley Citation2002). Mineral supplementation has been shown to enhance polyphenol accumulation in Avena sativa, leading to growth maintenance (Ahanger et al. Citation2015). Besides their role as antioxidants, flavonoids function in plant metabolism and are likely involved in various signaling mechanisms, as suggested by their interaction with protein kinases involved in initiating cell growth and differentiation (e.g. mitogen-activated protein kinases) (Brunetti et al. Citation2013).
5. Conclusion
Salt (NaCl) imposed osmotic and oxidative stress, leading to decreases in chlorophyll and RWC, along with increases in H2O2 production, lipid peroxidation, and electrolyte leakage. To combat salt stress, the B. juncea plants increased proline, ASA, and GSH accumulation, as well as SOD, APX, GR, and GST activities. Flavonoid content also increased with increasing NaCl concentrations and with developmental stages (30, 60, and 90 DAT). Zinc foliar application to NaCl-stressed plants decreased H2O2 production, lipid peroxidation, and electrolyte leakage while maintaining chlorophyll levels and RWC. It also further enhanced proline content, activities of antioxidant enzymes, and levels of non-enzymatic antioxidants. The increase in antioxidants and flavonoid content is thought to enhance ROS scavenging efficiency, thereby improving B. juncea tolerance to salt stress.
Disclosure statement
No potential conflict of interest was reported by the authors.
Additional information
Funding
References
- Ahanger MA, Agarwal RM. 2017. Salinity stress induced alterations in antioxidant metabolism and nitrogen assimilation in wheat (Triticum aestivum L.) as influenced by potassium supplementation. Plant Physiol Biochem. 115:449–460. doi: 10.1016/j.plaphy.2017.04.017
- Ahanger MA, Agarwal R, Tomar NS, Shrivastava M. 2015. Potassium induces positive changes in nitrogen metabolism and antioxidant system of oat (Avena sativa L. cultivar Kent). J Plant Interact. 10:211–223. doi: 10.1080/17429145.2015.1056260
- Ahanger MA, Tyagi SR, Wani MR, Ahmad P. 2014. Drought tolerance: role of organic osmolytes, growth regulators, and mineral nutrients. In: Ahmad P, Wani MR, editors. Physiological mechanisms and adaptation strategies in plants under changing environment, vol. 1. New York: Springer; p. 25–55.
- Ahmad P, Abdel Latef., Hashem A, AbdAllah EF., Gucel S, Tran L-SP. 2016b. Nitric oxide mitigates salt stress by regulating levels of osmolytes and antioxidant enzymes in chickpea. Front Plant Sci. 7: 347.
- Ahmad P, Allah EA, Hashem A, Sarwat M, Gucel S. 2016a. Exogenous application of selenium mitigates cadmium toxicity in Brassica juncea L. (Czern & Cross) by up-regulating antioxidative system and secondary metabolites. J Plant Growth Regul. 35:936–950 doi: 10.1007/s00344-016-9592-3
- Ahmad P, Ashraf M, Hakeem KR, Azooz M, Rasool S, Chandna R, Akram NA. 2014. Potassium starvation-induced oxidative stress and antioxidant defense responses in Brassica juncea. J Plant Interact. 9:1–9. doi: 10.1080/17429145.2012.747629
- Ahmad P, Hashem A, Abd-Allah EF, Alqarawi AA, John R, Egamberdieva D, Gucel S. 2015a. Role of Trichoderma harzianum in mitigating NaCl stress in Indian mustard (Brassica juncea L) through antioxidative defense system. Front. Plant Sci. 6:868
- Ahmad P, Sarwat M, Bhat NA, Wani MR, Kazi AG, Tran LSP, Zhang J-S. 2015b. Alleviation of cadmium toxicity in Brassica juncea L.(Czern. & Coss.) by calcium application involves various physiological and. PloS one. 10:e0114571 doi: 10.1371/journal.pone.0114571
- Ahmad P, Jaleel CA, Salem MA, Nabi G, Sharma S. 2010. Roles of enzymatic and nonenzymatic antioxidants in plants during abiotic stress. Crit Rev Biotechnol. 30:161–175. doi: 10.3109/07388550903524243
- Ali E, Mahmoud AM. 2013. Effect of foliar spray by different salicylic acid and zinc concentrations on seed yield and yield components of mungbean in sandy soil. Asian J Crop Sci. 5:33–40. doi: 10.3923/ajcs.2013.33.40
- Anderson ME. 1985. Determination of glutathione and glutathione disulfide in biological samples. Methods Enzymol. 113:548–555. doi: 10.1016/S0076-6879(85)13073-9
- Anjum NA, Aref IA, Pereira E, Ahmad A, Iqbal M. 2014. Glutathione and proline can coordinately make plants withstand the joint attack of osmotic and metal(loid) stresses. Front Plant Sci. 5:363. p. 4. DOI:10.3389/fpls.2014.00662.
- Anjum NA, Sofo A, Scopa A, Roychoudhury A, Gill SS, Iqbal M, Lukatkin AS, Pereira E, Duarte AC, Ahmad I. 2015. Lipids and proteins – major targets of oxidative modifications in abiotic stressed plants. Environ Sci Pollut Res. 22(6):4099–4121. doi: 10.1007/s11356-014-3917-1
- Ashraf M, McNeilly T. 2004. Salinity tolerance in brassica oilseeds. Crit Rev Plant Sci. 23:157–174. doi: 10.1080/07352680490433286
- Bates L, Waldren R, Teare I. 1973. Rapid determination of free proline for water-stress studies. Plant Soil. 39:205–207. doi: 10.1007/BF00018060
- Bose J, Rodrigo-Moreno A, Shabala S. 2014. Ros homeostasis in halophytes in the context of salinity stress tolerance. J Exp Bot. 65:1241–1257. doi: 10.1093/jxb/ert430
- Bradford MM. 1976. A rapid and sensitive method for the quantitation of microgram quantities of protein utilizing the principle of protein-dye binding. Anal Biochem. 72:248–254. doi: 10.1016/0003-2697(76)90527-3
- Brunetti C, Di Ferdinando M, Fini A, Pollastri S, Tattini M. 2013. Flavonoids as antioxidants and developmental regulators: relative significance in plants and humans. Int J Mol Sci. 14:3540–3555. doi: 10.3390/ijms14023540
- Dionisio-Sese ML, Tobita S. 1998. Antioxidant responses of rice seedlings to salinity stress. Plant Sci. 135:1–9. doi: 10.1016/S0168-9452(98)00025-9
- Dixon DP, Skipsey M, Edwards R. 2010. Roles for glutathione transferases in plant secondary metabolism. Phytochem. 71:338–350. doi: 10.1016/j.phytochem.2009.12.012
- Ebrahimian E, Bybordi A. 2011. Exogenous silicium and zinc increase antioxidant enzyme activity and alleviate salt stress in leaves of sunflower. J Food Agric Environ. 9:422–427.
- El-Tayeb M. 2005. Response of barley grains to the interactive effect of salinity and salicylic acid. Plant Growth Regul. 45:215–224. doi: 10.1007/s10725-005-4928-1
- Fang Z, Bouwkamp JC, Solomos T. 1998. Chlorophyllase activities and chlorophyll degradation during leaf senescence in non-yellowing mutant and wild type of Phaseolus vulgaris L. J Exp Bot. 49:503–510.
- Foyer CH, Halliwell B. 1976. The presence of glutathione and glutathione reductase in chloroplasts: a proposed role in ascorbic acid metabolism. Planta. 133:21–25. doi: 10.1007/BF00386001
- Gapińska M, Skłodowska M, Gabara B. 2008. Effect of short- and long-term salinity on the activities of antioxidative enzymes and lipid peroxidation in tomato roots. Acta Physiol Plant. 30:11–18. doi: 10.1007/s11738-007-0072-z
- Gill SS, Tuteja N. 2010. Reactive oxygen species and antioxidant machinery in abiotic stress tolerance in crop plants. Plant Physiol Biochem. 48:909–930. doi: 10.1016/j.plaphy.2010.08.016
- Gupta B, Huang B. 2014. Mechanism of salinity tolerance in plants: physiological, biochemical, and molecular characterization. Int J Plant Genomic. 2014: Article ID 701596.
- Habibi G. 2014. Hydrogen peroxide (H2O2) generation, scavenging and signaling in plants. In: Parvaiz A., editor. Oxidative damage to plants. San Diego (CA): Elsevier; p. 557–584.
- Hasanuzzaman M, Fujita M. 2013. Exogenous sodium nitroprusside alleviates arsenic-induced oxidative stress in wheat (Triticum aestivum L.) seedlings by enhancing antioxidant defense and glyoxalase system. Ecotoxicol. 22:584–596. doi: 10.1007/s10646-013-1050-4
- Hashem A, Abd_Allah E, Alqarawi A, El-Didamony G, Alwhibi M, Egamberdieva D, Ahmad P. 2014. Alleviation of adverse impact of salinity on faba bean (Vicia faba L.) by arbuscular mycorrhizal fungi. Pak J Bot. 46:2003–2013.
- Hayat S, Hayat Q, Alyemeni MN, Wani AS, Pichtel J, Ahmad A. 2012. Role of proline under changing environments: a review. Plant Signal Behav. 7:1456–1466. doi: 10.4161/psb.21949
- Heath RL, Packer L. 1968. Photoperoxidation in isolated chloroplasts: I. Kinetics and stoichiometry of fatty acid peroxidation. Arch Biochem Biophys. 125:189–198. doi: 10.1016/0003-9861(68)90654-1
- Hiscox JT, Israelstam G. 1979. A method for the extraction of chlorophyll from leaf tissue without maceration. Can J Bot. 57:1332–1334. doi: 10.1139/b79-163
- Hoagland DR, Arnon DI. 1940. Crop production in artificial culture solutions and in soils with special reference to factors influencing yield absorption of inorganic nutrients. Soil Sci. 50:463–483.
- Huang C, He W, Guo J, Chang X, Su P, Zhang L. 2005. Increased sensitivity to salt stress in an ascorbate-deficient Arabidopsis mutant. J Exp Bot. 56:3041–3049. doi: 10.1093/jxb/eri301
- Husen A, Iqbal M, Aref IM. 2016. IAA-induced alteration in growth and photosynthesis of pea (Pisum sativum L.) plants grown under salt stress. J Environ Biol. 37(3):421–429.
- Hussein M, Embiale A, Husen A, Aref IM, Iqbal M. 2017. Salinity-induced modulation of plant growth and photosynthetic parameters in faba bean (Vicia faba) cultivars. Pak J Bot. 49(3):867–877.
- Ibrahim S, Ibrahim HA, Omer AM. 2012. Comparative study of the effects of some organic extract on sugar beet yield under saline conditions. Aust J Basic Appl Sci. 6:664–674.
- Iqbal N, Umar S, Khan NA. 2015. Nitrogen availability regulates proline and ethylene production and alleviates salinity stress in mustard (Brassica juncea). J Plant Physiol. 178:84–91. doi: 10.1016/j.jplph.2015.02.006
- Jiang W, Sun X, Xu H, Mantri N, Lu H. 2014. Optimal concentration of zinc sulfate in foliar spray to alleviate salinity stress in Glycine soja. J Agr Sci Tech. 16:445–460.
- Luck H. 1971. Catalases. In: Bregmeyer H. U., editor. Methods of enzymatic analysis. New York (NY): Academic Press; p. 885–893.
- Majer P, Neugart S, Krumbein A, Schreiner M, Hideg E. 2014. Singlet oxygen scavenging by leaf flavonoids contributes to sunlight acclimation in Tilia platyphyllos. Environ Exper Bot. 100:1–9. doi: 10.1016/j.envexpbot.2013.12.001
- Nakano Y, Asada K. 1981. Hydrogen peroxide is scavenged by ascorbate-specific peroxidase in spinach chloroplasts. Plant Cell Physiol. 22:867–880.
- Novo LAB, Covelo EF, González L. 2014. Effect of salinity on zinc uptake by Brassica juncea. Int J Phytoremediation. 16:704–718. doi: 10.1080/15226514.2013.856844
- Osredkar J, Sustar N. 2011. Copper and zinc, biological role and significance of copper/zinc imbalance. J Clinic Toxicol. S3:001. doi: 10.4172/2161-0495.S3-001
- Prasad KVSK, Saradhi PP. 1995. Effect of zinc on free radicals and proline in Brassica and Cajanus. Phytochemistry. 39(1):45–47. doi: 10.1016/0031-9422(94)00919-K
- Rajendrakumar CS, Suryanarayana T, Reddy AR. 1997. DNA helix destabilization by proline and betaine: possible role in the salinity tolerance process. FEBS Lett. 410:201–205. doi: 10.1016/S0014-5793(97)00588-7
- Rasool S, Ahmad A, Siddiqi TO, Ahmad P. 2013. Changes in growth, lipid peroxidation and some key antioxidant enzymes in chickpea genotypes under salt stress. Acta Physiol Plant. 35:1039–1050. doi: 10.1007/s11738-012-1142-4
- Samreen T, Shah HU, Ullah S, Javid M. 2017. Zinc effect on growth rate, chlorophyll, protein and mineral contents of hydroponically grown mungbeans plant (Vigna radiata). Arab J Chem. 10:S1802–S1807. doi: 10.1016/j.arabjc.2013.07.005
- Siddiqui SN, Umar S, Iqbal M. 2015. Zinc-induced modulation of some biochemical parameters in a high-and a low-zinc-accumulating genotype of Cicer arietinum L. grown under Zn-deficient condition. Protoplasma. 252:1335–1345. doi: 10.1007/s00709-015-0767-8
- Singh S, Parihar P, Singh R, Singh VP, Prasad SM. 2015. Heavy metal tolerance in plants: role of transcriptomics, proteomics, metabolomics, and ionomics. Front Plant Sci. 6:1143.
- Smart RE, Bingham GE. 1974. Rapid estimates of relative water content. Plant Physiol. 53:258–260. doi: 10.1104/pp.53.2.258
- Tang X, Mu X, Shao H, Wang H, Brestic M. 2015. Global plant-responding mechanisms to salt stress: physiological and molecular levels and implications in biotechnology. Crit Rev Biotechnol. 35:425–437. doi: 10.3109/07388551.2014.889080
- Tavallali V, Rahemi M, Maftoun M, Panahi B, Karimi S, Ramezanian A, Vaezpour M. 2009. Zinc influence and salt stress on photosynthesis, water relations, and carbonic anhydrase activity in pistachio. Sci Hort. 123:272–279. doi: 10.1016/j.scienta.2009.09.006
- Tuna AL, Kaya C, Ashraf M, Altunlu H, Yokas I, Yagmur B. 2007. The effects of calcium sulphate on growth, membrane stability and nutrient uptake of tomato plants grown under salt stress. Environ Exp Bot. 59:173–178. doi: 10.1016/j.envexpbot.2005.12.007
- Tuteja N, Ahmad P, Panda BB, Tuteja R. 2009. Genotoxic stress in plants: shedding light on DNA damage, repair and DNA repair helicases. Mutat Res Rev Mutat Res. 681:134–149. doi: 10.1016/j.mrrev.2008.06.004
- van Rossum MW, Alberda M, van der Plas LH. 1997. Role of oxidative damage in tulip bulb scale micropropagation. Plant Sci. 130:207–216. doi: 10.1016/S0168-9452(97)00215-X
- Velikova V, Yordanov I, Edreva A. 2000. Oxidative stress and some antioxidant systems in acid rain-treated bean plants: protective role of exogenous polyamines. Plant Sci. 151:59–66. doi: 10.1016/S0168-9452(99)00197-1
- Wani AS, Ahmad A, Hayat S, Fariduddin Q. 2013. Salt-induced modulation in growth, photosynthesis and antioxidant system in two varieties of Brassica juncea. Saudi J Biol Sci. 20:183–193. doi: 10.1016/j.sjbs.2013.01.006
- Weisany W, Sohrabi Y, Heidari G, Siosemardeh A, Badakhshan H. 2014. Effects of zinc application on growth, absorption and distribution of mineral nutrients under salinity stress in soybean (Glycine max L.). J Plant Nutr. 37:2255–2269. doi: 10.1080/01904167.2014.920386
- Weisany W, Sohrabi Y, Heidari G, Siosemardeh A, Ghassemi-Golezani K. 2011. Physiological responses of soybean (Glycine max L.) to zinc application under salinity stress. Aust J Crop Sci. 5:1441.
- Winkel-Shirley B. 2002. Biosynthesis of flavonoids and effects of stress. Curr Opin Plant Biol. 5:218–223. doi: 10.1016/S1369-5266(02)00256-X
- Yan K, Shao H, Shao C, Chen P, Zhao S, Brestic M, Chen X. 2013. Physiological adaptive mechanisms of plants grown in saline soil and implications for sustainable saline agriculture in coastal zone. Acta Physiol Plant. 35:2867–2878. doi: 10.1007/s11738-013-1325-7
- Yousuf PY, Ahmad A, Aref IM, Ozturk M, Hemant GA, Iqbal M. 2016a. Salt-stress-responsive chloroplast proteins in Brassica juncea genotypes with contrasting salt tolerance and their quantitative PCR analysis. Protoplasma. 253:1565–1575. doi: 10.1007/s00709-015-0917-z
- Yousuf PY, Ahmad A, Ganie AH, Iqbal M. 2016b. Salt stress-induced modulations in the shoot proteome of Brassica juncea genotypes. Environ Sci Pollut Res. 23:2391–2401. doi: 10.1007/s11356-015-5441-3
- Zhishen J, Mengcheng T, Jianming W. 1999. The determination of flavonoid contents in mulberry and their scavenging effects on superoxide radicals. Food Chem. 64:555–559. doi: 10.1016/S0308-8146(98)00102-2