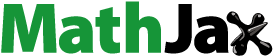
ABSTRACT
The increasing spread of ragworts is observed with concern. Ragworts like tansy ragwort (Jacobaea vulgaris Gaertn.) or marsh ragwort (J. aquatica) contain pyrrolizidine alkaloids (PA) which may induce hepatotoxic effects. Grazing animals usually avoid ragworts if their pasture management is appropriate. Preserved feed prepared from ragworts contaminated meadows may, however, lead to a significant exposure to PA. Previous studies on toxicity of PA for dairy cows revealed inconsistent results due to feeding ragwort plant material which was associated with heterogeneous PA exposure and thus failed to conclusively deduce critical PA doses. Therefore, the aim of the present study was to expose dairy cows (n = 4 per group) in a short-term scenario for 28 days with increasing PA doses (PA1: 0.47 mg PA/kg body weight (BW)/day (d); PA2: 0.95 mg PA/kg BW/d; PA3: 1.91 mg PA/kg BW/d) via oral administration by gavage of a defined PA-extract. While group PA3 was dosed with the PA-extract alone, groups PA2 and PA1 received PA-extracts blended in similar volumes with molasses to provide comparable amounts of sugar. Additionally, two control groups were treated either with water (CONWater) or with molasses (CONMolasses) to assess the effects of sugar without PA interference. While clinical traits including dry matter intake, milking performance, rectal body temperature, ruminal activity and body condition score (BCS) were not influenced by PA exposure, activities of enzymes indicative for liver damages, such as gamma-glutamyltransferase (GGT), aspartate aminotransferase (AST) and glutamate dehydrogenase (GLDH), increased significantly over time at an exposure of 1.91 mg total PA/kg BW/d.
1. Introduction
Pyrrolizidine alkaloids (PA) are toxic secondary plant metabolites, present in more than 6000 plant species and consisting of about 600 known isolated structures. PA are mostly present in the plant families Asteraceae, Boraginaceae, Fabaceae and Orchidaceae (Hartmann and Witte Citation1995; Hoogenboom et al. Citation2011; EFSA Citation2016).
The core structure of PA is an ester alkaloid of a necine base esterified with a necine acid. PA occur as monoesters, open-chain or macrocyclic diesters or even triesters. Chemical modifications lead to a great diversity of structures (Hartmann and Witte Citation1995; Fu et al. Citation2004). Furthermore, the nitrogen in the necine base can be oxidised resulting in the N-oxide form of the PA. Without this oxide, they are referred to as free base form. PA are mostly synthesised, stored and translocated in plants in the form of their corresponding N-oxides (Hartmann and Witte Citation1995).
In a situation of sufficient forage availability, most domesticated grazing animals avoid ragworts. In the production of preserved feed, especially hay or pressed pellets/cubes, from contaminated meadows ragworts can be mixed within these feedstuffs (Candrian et al. Citation1984; Berendonk et al. Citation2010). As a consequence, avoidant selection is barely possible for the animals and PA might be ingested through the offered individual feed or total mixed ration (TMR). Silage poses less of a risk, as a reduction of PA occurs during silage production (Gottschalk et al. Citation2015; Klevenhusen et al. Citation2022). The highest PA concentrations of feed prepared from contaminated meadows were found in Lower Austria during the flowering time in July and August, exceeding a total alkaloid concentration of 100 mg/kg in the green cuttings (Chizzola et al. Citation2015).
PA are protoxins. After ingestion, they are metabolically activated to reactive pyrrole esters by cytochrome P450 monooxygenases, mainly expressed in the liver. These pyrrole esters irreversibly bind to DNA or proteins and thereby induce toxic effects (Segall Citation1978; Mattocks et al. Citation1986; Hartmann and Witte Citation1995; Fu et al. Citation2004; Joosten et al. Citation2010; EFSA Citation2011; Wiedenfeld and Edgar Citation2011).
Previous studies have shown that the effects caused by PA include hepatoxicity, developmental toxicity, genotoxicity and carcinogenicity depending on dose, duration and species (Mattocks et al. Citation1986; Fu et al. Citation2004; Stegelmaier Citation2004; Hoogenboom et al. Citation2011; EFSA Citation2016). Acute toxicity assessment revealed lethal ragwort doses of 40–80 g/kg body weight (BW), 140 g/kg BW, and >1240 g/kg BW for horses, cows, sheep and goats, respectively (Lüscher et al. Citation2005). The ruminal PA metabolism reduces the N-oxide form to the corresponding free base form and finally results in saturated, non-toxic structures. Nevertheless, some PA show slow degradation rates (Taenzer et al. Citation2022). The ruminal metabolism provides an explanation for the decreased susceptibility of cows and other ruminants towards PA, compared to monogastric species (Cheeke Citation1988).
Ragwort intoxication in livestock is characterised by reduced feed intake, watery or bloody diarrhoea, decrease in BW, and it is accompanied by difficulties in walking and standing, as well as increased photosensitivity, rumen atony, colic-like symptoms and liver toxicity (Davidson Citation1935; Bull et al. Citation1968; Dickinson et al. Citation1976; Mattocks et al. Citation1986).
Studies regarding the effects of PA exposure on the health of dairy cattle are scarce. Previous studies were mostly performed using not always clearly defined plant material, particularly with regard to PA concentration and distribution in the administered plant batches (Dickinson et al. Citation1976; Shimshoni et al. Citation2015). For example, Dickinson et al. (Citation1976) used an analytical method for toxicants and milk where only the free base form was determined. However, the present N-oxides in feed are important regarding the overall toxicity due to the metabolism to the free base form and further rumen metabolites (Taenzer et al. Citation2022). Generally, the use of fresh, dried or ground plant material carries the risk of uneven PA administration with possible consequences for individually occurring toxic effects. The administration of 0.38 g dry matter (DM) Senecio brasiliensis/kg BW/day (d) in five calves over 24 d led to digestive complications during the trial, death of one calf after 45 d and fibrotic livers detected post-mortem (Torres and Coelho Citation2008). The administration of 10 g DM Jacobaea vulgaris/kg BW/d corresponding to approximately 16 mg PA/kg BW/d to four fistulated cows over 2 weeks led to a decrease in body weight as well as in milk yield (Dickinson et al. Citation1976). The experiment of Hoogenboom et al. (Citation2011) with three cows administered up to 200 g dried ragwort (2.3 g PA/kg DM; 0.66–0.77 mg PA/kg BW/d) did, however, not show a decrease in BW nor in milk yield. Based on those and other published results (e.g. Goeger et al. Citation1982; Johnson and Smart Citation1983; Stegelmaier Citation2004; Shimshoni et al. Citation2015; Mulder et al. Citation2016, Citation2020), the effective toxic dose of PA in ruminants including cows has poorly been defined so far mainly due to the uncertainty caused by PA variation and possible inhomogeneity of the used plant material.
The present study aims to identify PA dose-dependent effects in dairy cows in a short-term exposure scenario. An extract from dried tansy ragwort (Jacobaea vulgaris Gaertn.) was used as a PA source instead of plant material and was daily administered orally on a BW basis to avoid uncertainty caused by feeding.
2. Materials and methods
2.1. Production of the PA-extract
The used tansy ragwort (Jacobaea vulgaris Gaertn.) was harvested in summer 2019 from a meadow in northern Germany, which was naturally covered. The tansy ragwort was thereby picked by hand without the root. After drying the plant material, the PA-extract was prepared using aqueous methanol (90%) in several extraction steps. Afterwards, the methanol was removed via evaporation (Phytoplan, Heidelberg, Germany; Taenzer et al. Citation2022).
2.2. Experimental design and procedures
The trial was conducted at the experimental station of the Friedrich-Loeffler-Institut (FLI), Braunschweig, Germany, in agreement with the German Animal Welfare Act accepted by the Lower Saxony State Office for Consumer Protection and Food Safety (LAVES), Germany (file number 33.19425020419/3191).
The experiment was planned as a dose response study. For this, 20 pluriparous (2nd lactation), non-pregnant, clinically inconspicuous lactating German Holstein cows (169.7 ± 30 d in lactation; mean ± standard deviation (SD)) were assigned to five treatment groups (n = 4 per group) considering comparable milk yield and BW. Already over 3 weeks before the start of the experiment, all cows had been fed with the TMR that they also received later during the trial. The TMR consisted of 30% maize silage, 30% grass silage and 40% concentrate feed on a DM basis (). The cows were supplied with energy and nutrients, according to the recommendation of the Society of Nutrition Physiology (GfE Citation2001).
Table 1. Components, nutrient composition and energy content of the concentrate, the grass silage and the maize silage used for the total mixed ration.
Cows were housed in group pens, equipped with slatted floors and cubicles. Feed and water were offered ad libitum from computerised self-feeding stations (Insentec, B.V., Marknesse, The Netherlands), whereby the individual amounts of water and feed intake were recorded via ear transponders. Concentrate feed samples were collected weekly and pooled over the time of the trial. The maize and grass silage samples were collected and pooled twice a week. TMR samples were collected daily and then pooled.
To standardise the exposure levels corresponding to BW, the amount of PA-extract supposed to be fed was calculated based on the extract’s PA-concentration and the mean BW prior to the beginning of the experiment which resulted in the target-PA exposure of 0.45, 0.90 and 1.80 mg/kg BW/d for group PA1, PA2, PA3, respectively. The choice of the doses used in the present experiment was based on the carry over experiment reported by Hoogenboom et al. (Citation2011) where the PA exposure scenario relied on an increase in the amount of PA containing plant material during a period of 3 weeks and reached a maximum exposure of approximately 0.7 mg total PA/kg body weight when taking the reported daily dose of 450 mg total PA/d and an assumed body weight of 650 kg into account. With this exposure regimen, no clinical signs of toxicity were observed. As the focus of this experiment was on carry-over aspects and not on animal health, the authors concluded that the toxicity of PA for cows still needs to be investigated. Therefore, we designed our experiment as a dose–response experiment whereby a doubling of the doses was intended starting at a dose of 0.45 mg PA/kg body weight in order to have one dose lower than the mentioned 0.7 mg PA/kg body weight, one approximating this dose and a higher one. The realised PA-exposure deviated ≤6.1% from the target PA-exposure (). The PA-extract was administered via gavage, consistent in terms of time, once daily in a short-term exposure scenario for 28 d. Due to the high sugar content of the PA-extract, the control group CONMolasses was included in the trial’s design (). Group CONMolasses received volumes of sugar cane molasses (Hansa Melasse Handelsgesellschaft, Bremen, Germany) similar to those of group PA3, which obtained the total amount as PA-extract. For the control group CONWater, a similar volume of tap water was administered ().
Table 2. Pyrrolizidine alkaloid exposure and administration of the substances to the experimental groups.
Table 3. Nitrogen, methanol, carbohydrates and Klason lignin of the PA-extract and the sugar cane molasses [g/kg].
During the week before the start of the experiment, individual control samples of milk and blood were taken. On the day before the start of the trial (−1 d), an indwelling catheter was placed in a Vena jugularis externa for a stress-free blood sampling, and was removed again on day 3 of the experiment. The trial started with blood sampling directly before administration of the experimental substances (water, molasses, PA-extract). Oral administration of the substances was conducted daily after the morning milking via gavage. During the first 24 h, blood was sampled at 0.5 h, 1 h, 2 h, 3 h, 4 h, 5 h, 6 h, 12 h and 24 h after administration of the test substances (data not shown). During the first week of the trial, morning and afternoon milk as well as blood samples were taken once daily, except at days 4 and 6 where no blood samples were collected. In the following weeks (2–4), milk and blood were sampled once weekly, with an additional blood sampling on day 10 (Supplementary material, Figure S1). Blood was in each case sampled 1.5 h after the test substance administration. After storing it at 30°C for 30 min, the blood serum and the plasma were centrifuged (Heraeus Varifuge 3.0 R Heraeus, Osterode, Germany; 3000 U/min, 15°C, 15 min). Plasma for PA analysis was then stored at −20°C, while blood serum for analysis of clinical-chemical traits was kept at 80°C until further analyses. The cows were milked twice daily in the morning (5 a.m.) and afternoon (2 p.m.). The milk yield was determined automatically (Lemmer Fullwood GmbH, Lohmar, Germany), and the BW was recorded automatically by scale after leaving the milking parlour. Additionally, twice a week the morning and afternoon milk was sampled for further analyses of the milk components. Every week, the body condition score (BCS) was evaluated according to the 5-point scale proposed by Edmonson et al. (Citation1989), and a clinical monitoring of the cows including visual inspections of the mucous membranes and measurements of the rectal body temperature was performed. Furthermore, hints at central nervous disorders, like abnormal movement, blindness or cramps were examined. For detection of gastro-intestinal disorders, the appearance of faeces was evaluated optically concerning colour and consistency, and rumen contractions per 2 min were determined by auscultation. The stable climate data (temperature, humidity) were recorded with an automated sensor (smaXtec Climate Sensor, smaXtec animal care GmbH, Graz, Austria).
2.3. Analyses
2.3.1. Crude nutrients and further analyses of feed components, molasses, PA-extract and milk
The pooled and stored feed samples were dried at 60°C and ground in accordance with the methods of the Association of German Agricultural Inspection and Research Institutes (VDLUFA Citation2006) using the following methods: No. 3.1 for DM, No. 4.1.2 for Dumas combustion method for nitrogen, No. 5.1.1 for either extract, No. 6.1.1 for crude fibre, and No. 6.5.2 for aNDFOM. DM and total nitrogen in the PA-extract and in the molasses were also analysed by the aforementioned methods.
The milk samples were assayed for their composition (fat, protein, urea, and lactose) using an infrared milk analyser and somatic cell count (SCC) by flow cytometric measurement (Milchwirtschaftlicher Kontrollverband Mittelweser e.V., Rehburg-Loccum, Germany).
For the determination of the residues of methanol in the PA-extract and in the molasses the method after DIN EN 14,110 was used (LKS Labor, Lichtenwalde, Germany).
The quantification of carbohydrates in the PA-extract and in the molasses was done by an enzymatic assay for free glucose, fructose, sucrose and fructans according to Larsson and Bengtsson (Citation1983) and by an enzymatic-chemical-gravimetric procedure for low molecular weight (LMW) carbohydrates, soluble and insoluble non-starch polysaccharides (NSP), and Klason lignin (acid-insoluble residues) as described by Lærke et al. (Citation2015).
2.3.2. PA analysis in feed, molasses and PA-extract and blood plasma
PA concentrations of the feed components including molasses were analysed at the Ludwig-Maximilians-University of Munich (LMU). For this, 2 g of each the molasses, feed and PA-extract were mixed with 40 ml aqueous formic acid (2%), then centrifuged and filtrated over pleated filter. This was followed by solid-phase extraction (SPE) purification with PCX cartridges. The cartridges were eluted with ammoniated methanol, and the eluates were evaporated using nitrogen at 50°C and reconstituted in 500 µl aqueous methanol (10%). The quantification was performed by LC-MS/MS applying external solvent calibration. The lowest calibration point was used as the quantification limit (LOQ) for an analyte for which the formal specifications still met. The limits of detection (LOD) across all analytes varied between 0.01 and 1.0 µg/kg.
Analysis of PA levels of the PA-extract was performed by LMU as follows: The PA-extract was diluted with water (1/2000; 1/10,000; 1/20,000; 1/100,000; w/w) and subsequently filtered through a syringe filter (0.2 µm, regenerated cellulose, Macherey-Nagel, Düren, Germany) prior to LC-MS/MS analysis. Quantification was performed via external calibration by measuring six calibration standards in methanol (10%) ranging from 0.5 to 50 ng/ml of each analyte, or via standard addition by preparing and measuring one additional spiked sample (+10 ng/ml) of the 1/20,000 diluted extract.
A Shimadzu HPLC system (DGU-405, LC-20AB, SIL-20AC HT, CTO-20AC, CBM-20A, Duisburg, Germany) coupled to an API4000 triple quadrupole MS (Sciex, Darmstadt, Germany) was used by the LMU. Analysis of the PA-extract was performed at the beginning of the study using acidic chromatographic solvent conditions (Kaltner et al. Citation2019), while later for analysis of the feed and the molasses, a basic solvent A (10 mM ammonium carbonate at pH 9) and pure acetonitrile as solvent B (Klein et al. Citation2022) were used to improve chromatographic separation. Injection volume was 10 µl at a flow rate of 0.4 ml/min or 0.3 ml/min, respectively. The exact mass spectrometric settings, gradient programme and mass transitions were described in detail by Kaltner et al. (Citation2019) and Klein et al. (Citation2022).
The determination of PA in blood plasma and the PA extract was performed in a parallel analysis by the German Federal Institute for Risk Assessment (BfR) as follows: Prior to LC-MS/MS analysis, PA-extract samples were prepared by dilution with aqueous methanol (5%). After filtration through a centrifugal filter (modified nylon 0.2 µm, VWR, Radnor, Pennsylvania) the diluted PA-extract was directly analysed using a 10-point external calibration (working range 1 to 400.0 µg/kg). Plasma samples were prepared by injecting 200 µl of the plasma in 800 µl acetonitrile during shaking. The samples were sonicated, then centrifuged and 800 µl of the supernatant was dried under a stream of nitrogen. The residue was dissolved in 400 µl aqueous methanol (5%) and filtered through a centrifugal filter. Quantitation in plasma samples was achieved using a 10-point matrix-matched calibration (working range 0.5 to 200 µg/kg), whereby samples of group CONWater were not analysed.
All measurements of the BfR were conducted on an Agilent 1290 infinity series UHPLC system (Agilent Technologies, Santa Clara, USA). Chromatographic reversed-phase (RP) separation with 2 µl injection volume was performed on a C18 Hypersil Gold column (150 mm × 2.1 mm; 1.9 μm particle size) with guard column (Thermo Fisher Scientific) at a flow rate of 0.3 ml/min by gradient elution within 15 min (Supplementary material, Table S1). The binary mobile phase was composed of water as mobile phase A and methanol as mobile phase B, both containing 0.1% formic acid and 5 mmol ammonium formate. Electrospray ionisation tandem mass spectrometry (ESI-MS/MS) data were acquired in the positive ionisation mode on a QTRAP 6500 MS/MS system (Sciex, Washington, D.C., USA). Three MRM transitions were measured per analyte (Supplementary material, Table S2).
2.3.3. Clinical-chemical traits
The blood serum analyses for liver-associated parameters like albumin, bilirubin, urea, alkaline-phosphatase (ALP), creatinine, gamma-glutamyl-transferase (GGT), total protein, alanine aminotransferase (ALT), aspartate-aminotransferase (AST), glutamate dehydrogenase (GLDH) were performed using an automated analysis system (IndikoTM Plus, Thermo Fisher Scientific, Hennigsdorf, Germany).
2.4. Calculations
The energy content of the ration was calculated by using the equations for estimation of metabolisable energy (ME) and gross energy (GE) as provided by the Society of Nutrition Physiology (GfE Citation2001). These parameters are necessary to calculate the energy balance (EB).
Net energy intake (NEI):
Energy for maintenance requirement (NEM):
Net energy requirement for milk production (NEL):
Energy content of milk:
The EB was calculated by the difference between NEI, NEM and NEL (GfE Citation2001):
Energy corrected milk yield (ECM) was calculated according to the following equation by the Society of Nutrition Physiology (GfE Citation2001)
Temperature humidity index (THI) was calculated according to Hahn (Citation1999) using dry-bulb temperature [td, °C] and relative humidity (RH) expressed as decimal number:
2.5. Statistics
Results are presented as least square means (LS means) with standard errors (SE). All data were statistically analysed using the MIXED procedure (Littell et al. Citation1998) of SAS software (version 9.4; SAS Institute Inc., Cary, NC, USA). The mean value of the last two measurements before the first administration of the test substances was included as covariates in the model, except for the performance parameters (dry matter intake, bodyweight, energy balance and energy corrected milk yield), where the mean value of day −21 to day −8 before the start of the trial was used as covariate. For each measured variable, different covariance structures (compound symmetry (cs), variance components (vc), autoregressive (1) (AR (1)), unstructured (un)) were compared and selected for the best Akaike information criterion corrected for small sample sizes (AICC). The day and the group as well as their interaction were set as fixed factors. The cow was implemented as a random effect. Tukey adjusted t-test (PDIFF) evaluated differences between LS means and those were seen as significant if p-values were ≤0.05, while p-values ≤0.1 were considered as tendencies. If the model residuals did not show normal distribution, Box–Cox transformed data were used for statistics (Gurka et al. Citation2006) and transformed back for uniform presentation. In this case, the back-transformed 95% confidence intervals were used instead of SE.
3. Results
3.1. Composition of feed, molasses and the PA-extract
The analysed nutrient composition and the calculated NEL-concentrations of the feed are shown in . Analysis revealed that the PA-extract contained a methanol residue of 2.8 g/kg (). This resulted in a daily methanol exposure of 0.32 mg/kg BW/d, 0.64 mg/kg BW/d and 1.27 mg/kg BW/d for groups PA1, PA2, and PA3, respectively. Methanol concentration of molasses remained undetectable <0.001 g/kg. The DM content of the PA-extract and the molasses amounted to 68% and 79%, respectively, and total carbohydrates content to 34% and 37% (), respectively. Therefore, the administration of PA-extract and molasses resulted in additional sugar intake of 0.16 ± 0.01 g/kg BW/d for all groups except group CONWater. The total PA concentration of the PA-extract was 4184.4 mg PA/kg (). No PA were detected in maize and grass silage, whereas traces of individual PA different from the pattern of the PA-extract were found in concentrate feed and molasses.
Table 4. Concentration [mg/kg] of different PA in the feed [DM], the molasses [OM] and the PA-extract [OM] containing the percentual composition.
3.2. Environmental conditions
The temperature in the barn nearly constantly increased from day 1 to day 17. Afterwards, the temperature declined until day 28. The warmest period was the third week of the trial, with a mean temperature higher than 20°C (). During the first week, the relative humidity increased while during the second week the value decreased. Afterwards, it increased again until the end of the trial (). In this study, THI paralleled the progression of temperature. The THI increased until its maximum on days 17 and 18 and decreased afterwards until the end of the trial ().


![Figure 1. Temperature [°C] Display full size, relative humidity [%] Display full size and temperature-humidity-index (THI) Display full size in the barn during the trial. The area between the green dashed lines shows the “stress threshold” (68 < THI < 72) according to Pinto et al. (Citation2019). The values are presented as 24 hours means with half-whiskers as standard deviation.](/cms/asset/5612de2b-f542-4ab0-a969-cdd45a358069/gaan_a_2261806_f0001_oc.jpg)
3.3. Clinical signs, performance and realised PA-exposure
The weekly detailed clinical examination did not show any changes in the appearance of the mucous membranes and sclerae in any of the treatment groups. The BCS remained inconspicuous and varied between 2.25 and 2.75 across all groups. No signs of central nervous disorders were recognisable, and the behaviour was unremarkable throughout the trial. The rumen contractions amounted almost exclusively to 2–3 contractions in 2 min and no abnormal faeces could be detected in the animals. The rectal temperature ranged between 37.9°C and 38.7°C and was not affected by treatment nor time.
After termination of the experiment, the individually recorded BW were used for calculating the realised PA-exposure (, ). The targeted PA exposure was fulfilled by 95% and resulted in realised mean concentrations in blood plasma of 0.47, 0.95 and 1.91 mg PA/kg BW/d for group PA1, PA2 and PA3, respectively (, ). Due to the very low PA concentrations of the concentrate feed and the molasses, the control groups were only slightly exposed.
Figure 2. Time course of pyrrolizidine alkaloid (PA)-exposure per kg bodyweight (BW) [a] and PA-concentration in plasma [b] in dairy cows depending on PA-exposure groups: CONMolasses



![Figure 2. Time course of pyrrolizidine alkaloid (PA)-exposure per kg bodyweight (BW) [a] and PA-concentration in plasma [b] in dairy cows depending on PA-exposure groups: CONMolasses Display full size (<0.01 mg PA/kg BW/d), PA1 Display full size (0.47 mg PA/kg BW/d), PA2 Display full size (0.95 mg PA/kg BW/d), PA3 Display full size (1.91 mg PA/kg BW/d). Symbols represent LS means (n = 4) and half-whiskers as standard error, or whiskers as 95% confident interval for Box–Cox and reverse transformed data.](/cms/asset/8a6e73c5-501e-427b-af06-8e8cf686ffd3/gaan_a_2261806_f0002_oc.jpg)
PA were detected in blood plasma prior to the first treatment at day 0 with an individual concentration of up to 0.83 ng/ml. The mean concentration of PA in plasma for group CONMolasses (≤0.01 ng/ml) stayed constant over the whole trial. PA concentrations could be detected in blood plasma, which were dependent on the administered PA-extract dosage. During the trial, group PA1 showed a mean concentration of PA in plasma of 22.6 ± 3.2 ng/ml, while the mean concentration of group PA2 (69.2 ± 3.9 ng/ml) was about three times higher. One cow in group PA2 demonstrated the highest concentration over the whole trial with a maximum concentration of 292 ng/ml. This increased the mean of the PA concentration in plasma for the whole group PA2. Leaving this aspect aside, the mean PA concentration in the plasma ranged from 30.6 to 67 ng/ml for the three other individual animals of group PA2. In group PA3, a mean concentration of 116.2 ± 8.5 ng/ml was measured. There was a high degree of individual variation between the animals (69.8-166.2 ng/ml), but within each animal the PA concentration in blood plasma changed only slightly during the trial. Individual PA composition in blood plasma differs from that of the PA-extract. In blood plasma, the most abundant individual PA were in decreasing order jacobine Noxide, jaconine, jacoline in group PA1, and jacobine N-oxide, jaconine N-oxide and jaconine in groups PA2 and PA3 (data not shown), while the most abundant PA in the extract were jaconine, jaconine N-oxide and seneciphylline N-oxide in decreasing order ().
All groups showed a decreasing DM intake () during the period of 3 d before the start of the experiment until day 0. Afterwards, all groups displayed a similar progression with an increase in the mean DM intake until day 11 and a small negative peak on day 7. Until the end of the trial, the mean DM intake then slightly decreased. This course resulted in a significant time effect (p < 0.001). The BW () showed a decrease from day −3 to day 1 of the trial across all groups. The mean BW increased afterwards until day 7 followed by a decrease until day 12 and after that returned to the value of 1 week before the start of the experiment and stayed constant. This variation resulted in a significant time effect (p < 0.001) but stayed unaffected by treatment. The EB () showed a progression similar to the DM intake in the week before the start of the trial, with a sharp negative peak on day 0 across all groups. The EB increased again afterwards, showing a strong rise around day 10 and then decreased in a relatively constant way. From the beginning of the trial, the EB of the PA groups stayed slightly under the EB of the control groups. Fluctuations during the study resulted in significant time effects (p < 0.001) and the differences between the groups led to a tendency towards group effects (p = 0.068). The milk yield, milk fat, protein, urea and lactose content as well as the somatic cell count (SCC) stayed unaffected by treatment, while the fat, urea and lactose content of the milk as well as the fat-to-protein ratio indicated a significant time dependent (pDay < 0.001) variation during the trial (). Nevertheless, the resulting ECM () was not significantly affected by the treatments but showed a sharp decrease from day −1 to day 1 of the study. Subsequently, the mean ECM increased until day 4 and then fluctuated until the end of the trial in a slightly negative direction, resulting in a significant time effect (p < 0.001).
Figure 3. Time course, from one week before the start until the end of the trial, of dry matter (DM)intake [a], bodyweight (BW) [b], energy balance (EB) [c] and energy corrected milk yield (ECM) [d] in dairy cows depending on pyrrolizidine alkaloid (PA) exposure groups: CONWater




![Figure 3. Time course, from one week before the start until the end of the trial, of dry matter (DM)intake [a], bodyweight (BW) [b], energy balance (EB) [c] and energy corrected milk yield (ECM) [d] in dairy cows depending on pyrrolizidine alkaloid (PA) exposure groups: CONWater Display full size (<0.01 mg PA/kg BW/d), CONMolasses Display full size (<0.01 mg PA/kg BW/d), PA1 Display full size (0.47 mg PA/kg BW/d), PA2 Display full size (0.95 mg PA/kg BW/d), PA3 Display full size (1.91 mg PA/kg BW/d). Symbols represent LS means (n = 4) and half-whiskers as standard error. The light blue bar shows day 0, the start of the trial.](/cms/asset/25090a34-b405-4943-b137-1e8e956c6b85/gaan_a_2261806_f0003_oc.jpg)
Table 5. Milk yield, energy corrected milk yield (ECM), fat-, protein-, urea-, lactose content of the milk, as well as somatic cell count (SCC).
3.4. Clinical-chemical traits
Total protein, albumin, bilirubin, urea, ALP, and creatinine measured in blood serum were not significantly influenced by treatments but varied significantly over time (pDay < 0.001, except total protein: pDay = 0.005 and creatinine: pDay = 0.023, ). The concentration of the total protein () showed an initial decrease across all groups with the minimum on day 3 and increased afterwards. After day 10, total protein concentration stayed nearly stable. Albumin concentration () showed like total protein a similar negative peak until day 3. The mean concentration increased until day 14 and then slowly decreased until the end of the trial. Bilirubin values () showed a peak at day 1 followed by a decrease in the mean concentration until day 5. Afterwards, the mean concentration stayed stable. Urea () varied during the experiment. The mean concentration increased from day 0 to day 1 just to decrease again until day 3. Thereafter, the mean value increased until day 10 followed by a slow decrease until the end of the experiment. The mean concentration of ALP () decreased nearly constantly from the beginning of the trial until day 21 and increased afterwards, depending on the treatment during the last week of the trial. The concentration of group PA3 started to increase from day 14 on and resulted in a significant difference between the concentrations of group PA3 and group CONWater on day 28 (p = 0.029). The mean concentration of creatinine () showed a peak on day 2. Afterwards, it sharply decreased until day 5, started to increase again until day 10 and fell back on the starting concentration on day 14. Thereafter, the mean value increased until the trial ended.
Figure 4. Time course of total protein [a], albumin [b], bilirubin [c], urea [d], alkaline phosphatase (ALP) [e], creatinine [f] in blood serum of dairy cows depending on pyrrolizidine alkaloid (PA) exposure groups: CONWater




![Figure 4. Time course of total protein [a], albumin [b], bilirubin [c], urea [d], alkaline phosphatase (ALP) [e], creatinine [f] in blood serum of dairy cows depending on pyrrolizidine alkaloid (PA) exposure groups: CONWater Display full size (<0.01 mg PA/kg BW/d), CONMolasses Display full size (<0.01 mg PA/kg BW/d), PA1 Display full size (0.47 mg PA/kg BW/d), PA2 Display full size (0.95 mg PA/kg BW/d), PA3 Display full size (1.91 mg PA/kg BW/d). Symbols represent LS means (n = 4) with half-whiskers as standard error, or whiskers as 95% confident interval for Box–Cox and reverse transformed data.](/cms/asset/305190aa-2803-4b62-9709-abb29d0113b8/gaan_a_2261806_f0004_oc.jpg)
The mean concentration of ALT () dropped from the beginning of the trial until day 15 with a slight increase from day 3 to day 7. After day 15, the mean concentration increased constantly until the end of the experiment resulting in a significant time effect over the entire trial (p < 0.001). GGT activity () decreased slightly in all groups at a comparable level until day 7 and remained nearly constant in groups CONWater, CONMolasses, and PA1 until the trial’s end. In contrast, GGT activity increased in groups PA2 and PA3 until day 28, as expressed in the significant interactions between time and treatment (p = 0.011). Compared to the initial GGT activity the final value was significantly higher on day 28 in group PA3 (p = 0.003). Furthermore, the difference between GGT activity of group CONMolasses and group PA3 on day 28 was significant (p = 0.002). The time and treatment-related activities of AST and GLDH followed almost that of GGT. Both liver enzymes slightly decreased until day 7 of the experiment before they started to increase only in group PA3 ().
Figure 5. Time course of alanine aminotransferase (ALT) [a], gamma-glutamyltransferase (GGT) [b], aspartate aminotransferase (AST) [c], glutamate dehydrogenase (GLDH) [d] in blood serum of dairy cows depending on pyrrolizidine alkaloid (PA) exposure groups: CONWater




![Figure 5. Time course of alanine aminotransferase (ALT) [a], gamma-glutamyltransferase (GGT) [b], aspartate aminotransferase (AST) [c], glutamate dehydrogenase (GLDH) [d] in blood serum of dairy cows depending on pyrrolizidine alkaloid (PA) exposure groups: CONWater Display full size (<0.01 mg PA/kg BW/d), CONMolasses Display full size (<0.01 mg PA/kg BW/d), PA1 Display full size (0.47 mg PA/kg BW/d), PA2 Display full size (0.95 mg PA/kg BW/d), PA3 Display full size (1.91 mg PA/kg BW/d). Symbols represent LS means (n = 4) with whiskers as 95% confident interval for Box–Cox and reverse transformed data.](/cms/asset/eb60221e-ad92-4b91-8aaa-30ec03b5daa9/gaan_a_2261806_f0005_oc.jpg)
4. Discussion
Contrary to the often-used plant materials, the PA-extract applied in this study was much better suited to attain accurate PA exposure levels. The well-characterised PA-extract together with the administration regiment allowed achieving the targeted PA exposure levels (). Besides the PA-extract, traces of PA detected in molasses and concentrate feed contributed to the overall PA exposure of the cows (). Taken together, the trace contamination of both accounted, however, for only less than 0.0002% of the groups’ PA exposure, and was thus seen as negligible concerning the study results.
For the present study, a PA-extract from Jacobaea vulgaris Gaertn. was employed in contrast to previous studies where intact, dried and/or ground plant material was used (Dickinson et al. Citation1976; Torres and Coelho Citation2008; Hoogenboom et al. Citation2011; Shimshoni et al. Citation2015; de Nijs et al. Citation2017). Individually detected PA of the PA-extract summed up to a total PA concentration of 4184 mg PA/kg, here ().
It is important to know the specific composition of the PA-extract, as the individual alkaloids might have different toxicities (Macel et al. Citation2004; Berendonk et al. Citation2010; Rutz et al. Citation2020; Taenzer et al. Citation2022). Different ragwort species are characterised by varying PA patterns (Witte et al. Citation1992) and additionally, the season as well as the part of the plant, e.g. stem, leaf or blossom further modify the level and pattern of PA (Bosshard et al. Citation2003; Berendonk et al. Citation2010). Because of this variation in pattern and toxicity of PA, similar total PA exposure levels might have different toxic outcomes. Furthermore, it needs to be stressed that the extraction procedure applied to the plant material used for the present study might also have resulted in PA patterns different from genuine PA compositions.
Besides the PA, the used PA-extract consisted of approximately up to one-third of carbohydrates nearly exclusively composed of individual low molecular weight sugars () which could give rise to a ruminal dysbiosis associated with a ruminal acidosis (Sharp et al. Citation1982). Some signs of acute rumen acidosis are, among others, a decreased milk yield and in the worst case it could even mean death (Snyder and Credille Citation2017). Therefore, the co-occurrence of PA and large amounts of sugars in the PA-extract would result not only in increasing PA exposures but also in growing ruminal sugar load when PA dosage is increased. To avoid or minimise such effects, similar volumes of a mixture of PA-extract and molasses were administered to all dosing groups (). Furthermore, two control groups were implemented, a water control group (CONWater) and a molasses control group (CONMolasses) to identify possible pure sugar effects without the influence of PA. However, no significant differences were found between the two control groups during the trial in any of the recorded parameters. Therefore, it can be excluded for the parameters described here that the administration of a high daily ruminal load of sugars had an extra influence on the results observed in the PA dosing groups.
Furthermore, residues of methanol were detected in the PA-extract. Due to toxic metabolites of methanol, its intake should be critically evaluated. In 2009, the Deutsche Landwirtschafts-Gesellschaft (DLG) requested a maximum limit of 0.2% methanol in crude glycerol for feeding purposes derived from biodiesel production. It is recommended not to add more than 5% of crude glycerine to the feed (DLG, Citation2009). Based on the methanol concentration of 2.8 g/kg measured in the PA-extract employed in the present trial () the additional methanol exposure was 0.32, 0.64 and 1.27 mg/kg BW/d for group PA1, PA2 and PA3, respectively. These exposure levels are lower than the accepted daily methanol intake associated with the recommended maximum glycerol usage in feeding. Therefore, the additional methanol exposure through the PA extract in this trial should not have contributed to or interfered with the results.
The first symptoms of PA poisoning are loss of appetite, standing apart from other animals, depression, characterised by lack of interest, aversion to move or wandering as well as diarrhoea and ascites (Bull et al. Citation1968; Johnson Citation1978; Craig et al. Citation1991). Previous studies have shown that cows respond very individually to PA exposure (Johnson Citation1978; Craig et al. Citation1991). In the present study, no signs of loss of appetite were observed as DM intake did not differ between the groups (). Fluctuations in DM intake in the course of the experiment occurred for all treatment groups in a similar manner and caused similarly directed variation in BW and EB (). The slight drop in DM intake in the third week of the trial coincided with an increase in THI () but was not influenced by the treatment. Clinical examinations showed throughout the trial no signs of central nervous disorders due to an increase in PA exposure. The rumen contractions stayed in normal ranges with approximately three contractions within 120 s. Furthermore, faeces appeared inconspicuous, suggesting that rumination and digestion were not adversely affected by PA exposure. Taenzer et al. (Citation2022) had shown that the ruminal metabolism acts as PA detoxification with the metabolisation towards the non-toxic unsaturated form. Therefore, there might be no PA induced effects concerning the clinical traits under the given conditions.
Nevertheless, Dickinson et al. (Citation1976) showed that a very high exposure of 16 mg PA/kg BW/d (supplied as dried and chopped plant material via rumen cannula) led to a uniformly decreased BW and milk yield in dairy cows. Mulder et al. (Citation2020) did, however, not observe any decrease in feed intake nor milk yield after an administration of 200 g plant material of three weeds (weed 1: mixture of J. vulgaris and S. inaequidens; weed 2: S. vulgaris; weed 3: Echium vulgare) per day with PA concentrations up to 3767 mg/kg (~1.1 ± 0.1 mg PA/kg BW/d) in the plant material mixture of J. vulgaris and S. inaequidens. In the present study, no decrease in BW nor milk yield was visible with increasing PA exposure. The initial drop in DM intake and the related parameters BW, ECM and EB were probably caused by the stress through the high frequency of blood sampling during the first day of the experiment (). These results concerning BW and milk yield are comparable to studies of Craig et al. (Citation1991) with similar PA doses (1.3 mg PA/kg BW/d, provided by whole plant material) administered over a period of 2 months that did not affect the body weight. Furthermore, Hoogenboom et al. (Citation2011) did not observe any changes concerning milk yield nor regarding the milk components protein, fat or lactose content, which was also confirmed by the present study ().
Looking at the PA concentrations in the blood of the individual animals as indicators of the inner PA exposure, one cow of group PA2 was identified with PA concentrations (243.5 ± 56.1 ng/ml) even higher than those of the animals in group PA3. That individual cow in group PA2 impressed consistently with higher blood PA levels compared to her counterparts. This could hint at an individual sensitivity to PA due to a genetic component for PA kinetics and/or a prior history of liver or other diseases. Interestingly, the described cow demonstrated not only higher PA levels in blood but also a more pronounced increase in GGT, GLDH and AST compared to her counterparts which could suggest an altered hepatic PA metabolism which may be due to hepatocellular lesions. Rats receiving 0.3 mg riddelliine/kg BW/d over 105 weeks developed hepatocyte cytomegaly as a non-carcinogenic effect (Chan et al. Citation2003). Taking into consideration the elevated liver enzyme activities observed in the present study, histopathological changes cannot be excluded.
Besides this individual story, the highest PA exposure generally led to a significant time-dependent increase in serum enzymes indicative of liver damage (GGT, GLDH and AST) with a similar trend for ALP (Kauppinen Citation1984; Meyer and Harvey Citation1998; Stojević et al. Citation2005). The exponential increase in liver lesion indicating enzyme activities in peripheral blood including GGT in group PA3, and also in group PA2 from week 3 to 4 of the experiment might suggest the onset of chronic toxicity which would have been more visible in an exposure time exceeding the 4-week period investigated in the present study. These increases in liver enzymes in the present study are supported by the results of Johnson (Citation1978) where the enzymes AST and GGT increased after about 14 d due to an administration of ≥1.5 g tansy ragwort/kg BW/d. A case study by Shimshoni et al. (Citation2015) described significant increases in the concentrations of GGT, AST, creatinine and ALP and a lethality of 30% in cattle (15–18-months-old) within 26 weeks corresponding to an average total PA (Heliotropium europaeum) exposure of 33 mg/kg BW/d. In contrast, in the study of Molyneux et al. (Citation1991) an exposure of 4.5 mg riddelliine/kg BW/d over a period of 20 d did not affect the serum enzymes GGT and AST; this is consistent with our observations where a significant difference occurred only at day 28 and supports the progressive character of a PA poisoning. Besides the significant changes in GGT, GLDH and AST on day 28 () of the present study, there was a visible increase in ALP activity in blood in the highest exposed group (PA3) and on the same day, even significant differences compared to the group CONWater () were reached. Based on other studies (Craig et al. Citation1991; Shimshoni et al. Citation2015) a progressive increase in ALP in a prolonged exposure scenario might have been expected and is supported by the fact that nearly all animals of group PA2 and PA3 indeed showed a numerical ALP increase on day 28.
While GGT, GLDH and AST were proven to respond sensitively to the PA exposure scenario, the other clinical-chemical traits (total protein, albumin, bilirubin, urea, creatinine () and ALT () stayed inconspicuous. Among these non-sensitive parameters, particularly albumin is of interest. Albumin is produced exclusively by the liver (Kraft and Dürr Citation2013). A compromised liver function could lead to lowered albumin concentrations in the blood due to a decrease in hepatic albumin synthesis. At a high PA exposure of 16 mg/kg BW/d, the concentration of albumin in blood plasma decreased which was traced back to a reduced liver activity and a resulting reduced albumin synthesis (Dickinson et al. Citation1976). The albumin concentrations were not affected in the present experiment. However, this can be attributed to the markedly lower PA exposure.
5. Conclusions
The current results are only valid for the applied study conditions including the used PA pattern which might have been influenced by the extraction procedure and the dosage regimen. It was shown that blood levels of GGT, AST and GLDH were the most sensitive toxicological endpoints in dairy cows of the current study with a short-term PA exposure scenario which included the administration of a PA-extract once daily for a period of 28 d. PA hepatoxicity in cows develops in a time-dependent manner. The levels of these enzymes increased significantly until the end of the exposure period when provided a dose of 1.91 mg PA/kg BW/d, which might hint at the beginning of liver damages. Therefore, we cannot exclude that this possible onset in chronic liver toxicity even occurs at lower PA doses in different long-term scenarios. Thus, we have to recommend that the prevention of contamination of preserved feed for cows by PA containing plants is the only possibility to protect cows from PA toxicity. Based on the limitations of the present experiment, future studies should take into consideration a prolonged PA exposure, different dosing regimens (e.g. more than once daily) and the PA pattern. The questions of reversibility of liver lesions after cessation of PA exposure also justify future examinations. Regarding the effects of specific PA or particular PA patterns, testing similar PA exposure levels but consisting of distinctly different PA composition would be of particular interest. Finally, the question arose whether the PA had dose-dependent effects only on the liver itself or also on other organs.
Supplemental Material
Download PDF (688.2 KB)Acknowledgments
Special thanks goes to Nicola Mickenautsch and the entire laboratory staff of the Institute of Animal Nutrition, Friedrich-Loeffler-Institut. We also want to thank the staff of the Experimental Station Braunschweig of the Friedrich-Loeffler-Institut and Imke Steen for their great effort during the experiment.
Disclosure statement
No potential conflict of interest was reported by the author(s).
Supplementary material
Supplementary data for this article can be accessed at https://doi.org/10.1080/1745039X.2023.2261806.
Additional information
Funding
References
- Berendonk C, Cerff D, Hünting K, Wiedenfeld H, Becerra J, Kuschak M. (2010). Pyrrolizidine alkaloid level in Senecio jacobaea and Senecio erraticus-the effect of plant organ and forage conservation. In Prococeedings of the 23rd Geneneral Meeting of the European Grassland Federation, Grassland in a Changing World. Vol. 15; Kiel, Germany. p. 669–671.
- Bosshard A, Joshi J, Lüscher A, Schaffner UJA. 2003. Tansy ragwort and other ragwort species in Switzerland: an overview. Agrarforschung. 10:231–235.
- Bull LB, Culvenor CT, Dick A. 1968. The pyrrolizidine alkaloids: their chemistry, pathogenicity and other biological properties. 9.
- Candrian U, Luthy J, Schmid P, Schlatter C, Gallasz E. 1984. Stability of pyrrolizidine alkaloids in hay and silage. J Agr Food Chem. 32:935–937.
- Chan PC, Haseman JK, Prejean JD, Nyska A. 2003. Toxicity and carcinogenicity of riddelliine in rats and mice. Toxicol Lett. 144:295–311.
- Cheeke PR. 1988. Toxicity and metabolism of pyrrolizidine alkaloids. J Anim Sci. 66:2343–2350.
- Chizzola R, Bassler G, Kriechbaum M, Karrer G. 2015. Pyrrolizidine alkaloid production of jacobaea aquatica under different cutting regimes. J Agr Food Chem. 63:1293–1299.
- Craig A, Pearson E, Meyer C, Schmitz J. 1991. Serum liver enzyme and histopathologic changes in calves. Am J Vet Res. 52:12.
- Davidson J. 1935. The action of retrorsine on rat’s liver. J Pathol Bacteriol. 40:285–295.
- de Nijs M, Mulder PP, Klijnstra MD, Driehuis F, Hoogenboom RL. 2017. Fate of pyrrolizidine alkaloids during processing of milk of cows treated with ragwort. Food Addit Contam. 34:2212–2219.
- [DLG] Deutsche Landwirtschafts-Gesellschaft. 2009. Zum Einsatz von Glycerin in der Fütterung. DLG-Fütterungsempfehlung. Stellungnahme des DLG-Arbeitskreises Futter und Fütterung. Frankfurt am Main: DLG e.V.
- Dickinson JO, Cooke MP, King RR, Mohamed PA. 1976. Milk transfer of pyrrolizidine alkaloids in cattle. J Am Vet Med Assoc. 169:1192–1196.
- Edmonson AJ, Lean IJ, Weaver LD, Farver T, Webster G. 1989. A body condition scoring chart for Holstein Dairy-Cows. J Dairy Sci. 72:68–78.
- [EFSA] European Food Safety Authority. 2011. Evaluation of the FoodEx, the food classification system applied to the development of the EFSA comprehensive European Food consumption database. EFSA J. 9:1970.
- [EFSA] European Food Safety Authority. 2016. Dietary exposure assessment to pyrrolizidine alkaloids in the European population. EFSA J. 14:e04572.
- Fu PP, Xia Q, Lin G, Chou MW. 2004. Pyrrolizidine alkaloids—genotoxicity, metabolism enzymes, metabolic activation, and mechanisms. Drug Metab Rev. 36:1–55.
- [GfE] Gesellschaft für Ernährungsphysiologie. 2001. Ausschuss für Bedarfsnormen der Gesellschaft für Ernährungsphysiologie: Empfehlung zur Energie- und Nährstoffversorgung der Milchkühe und Aufzuchtrinder. Frankfurt am Main, Germany: DLG-Verlag.
- Goeger D, Cheeke P, Schmitz J, Buhler D. 1982. Toxicity of tansy ragwort (Senecio jacobaea) to goats. Am J Vet Res. 43:252–254.
- Gottschalk C, Ronczka S, Preiß-Weigert A, Ostertag J, Klaffke H, Schafft H, Lahrssen-Wiederholt M. 2015. Pyrrolizidine alkaloids in natural and experimental grass silages and implications for feed safety. Ani Feed Sci Tech. 207:253–261.
- Gurka MJ, Edwards LJ, Muller KE, Kupper LL. 2006. Extending the Box–Cox transformation to the linear mixed model. J R Stat Soc Ser A Stat Soc. 169:273–288.
- Hahn G. 1999. Dynamic responses of cattle to thermal heat loads. J Anim Sci. 77:10–20.
- Hartmann T, Witte L. 1995. Chapter four - chemistry, biology and chemoecology of the pyrrolizidine alkaloids. In: Pelletier SW, editor. Alkaloids: chemical and biological perspectives. Vol. 9. Pergamon; p. 155–233.
- Hoogenboom LAP, Mulder PPJ, Zeilmaker MJ, van den Top HJ, Remmelink GJ, Brandon EFA, Klijnstra M, Meijer GAL, Schothorst R, Van Egmond HP. 2011. Carry-over of pyrrolizidine alkaloids from feed to milk in dairy cows. Food Addit Contam. 28:359–372.
- Johnson A. 1978. Tolerance of cattle to tansy ragwort (Senecio jacobaea). Am J Vet Res. 39:1542–1544.
- Johnson A, Smart R. 1983. Effects on cattle and their calves of tansy ragwort (Senecio jacobaea) fed in early gestation. Am J Vet Res. 44:1215–1219.
- Joosten L, Mulder PPJ, Vrieling K, van Veen JA, Klinkhamer PGL. 2010. The analysis of pyrrolizidine alkaloids in jacobaea vulgaris; a comparison of extraction and detection methods. Phytochem Anal. 21:197–204.
- Kaltner F, Stiglbauer B, Rychlik M, Gareis M, Gottschalk C. 2019. Development of a sensitive analytical method for determining 44 pyrrolizidine alkaloids in teas and herbal teas via LC-ESI-MS/MS. Anal Bioanal Chem. 411:7233–7249.
- Kauppinen K. 1984. ALAT, AP, ASAT, GGT, OCT activities and urea and total bilirubin concentrations in plasma of normal and ketotic dairy cows. Zentralblatt für Veterinärmedizin Reihe A. 31:567–576.
- Klein LM, Gabler AM, Rychlik M, Gottschalk C, Kaltner F. 2022. A sensitive LC–MS/MS method for isomer separation and quantitative determination of 51 pyrrolizidine alkaloids and two tropane alkaloids in cow’s milk. Anal Bioanal Chem. 414:8107–8124.
- Klevenhusen F, These A, Taenzer J, Weiß K, Pieper R. 2022. Effects of ensiling conditions on pyrrolizidine alkaloid degradation in silages mixed with two different Senecio spp. Arch Anim Nutr. 76:93–111.
- Kraft W, Dürr UM. 2013. Klinische Labordiagnostik in der Tiermedizin. Stuttgart: Schattauer Verlag.
- Lærke HN, Arent S, Dalsgaard S, Bach Knudsen KE. 2015. Effect of xylanases on ileal viscosity, intestinal fiber modification, and apparent ileal fiber and nutrient digestibility of rye and wheat in growing pigs. J Anim Sci. 93:4323–4335.
- Larsson K, Bengtsson S. 1983. Determination of readily available carbohydrates in plant material. Natl Lab Agri Chem Meth Rep J. 22:10.
- Littell RC, Henry P, Ammerman CB. 1998. Statistical analysis of repeated measures data using SAS procedures. J Anim Sci. 76:1216–1231.
- Lüscher A, Siegrist S, Suter M, Stutz C, Gago R, Bucheli T. 2005. Kreuzkrautarten in Wiesen und Weiden: Vorbeugen–früh erkennen–früh bekämpfen. Unkrautbekämpfung-Neue Technologien, reduzierter Herbizideinsatz und Alternativen. 14:1–7.
- Macel M, Vrieling K, Klinkhamer PGL. 2004. Variation in pyrrolizidine alkaloid patterns of Senecio jacobaea. Phytochem. 65:865–873.
- Mattocks A, Driver HE, Barbour R, Robins D. 1986. Metabolism and toxicity of synthetic analogues of macrocyclic diester pyrrolizidine alkaloids. Chem Biol Interact. 58:95–108.
- Meyer DJ, Harvey JW. 1998. Evaluation of hepatobiliary system and skeletal muscle and lipid disorders. Veterinary laboratory medicine. Interpretation and diagnosis. 2nd Edn., Philadelphia, London, Toronto, Montreal. Meyer, DJ, Vet. archive. 75(1):67–73.
- Molyneux R, Johnson A, Olsen J, Baker D. 1991. Toxicity of pyrrolizidine alkaloids from Riddell groundsel (Senecio riddellii) to cattle. Am J Vet Res. 52:146–151.
- Mulder PP, de Witte SL, Stoopen GM, van der Meulen J, van Wikselaar PG, Gruys E, Groot MJ, Hoogenboom RL. 2016. Transfer of pyrrolizidine alkaloids from various herbs to eggs and meat in laying hens. Food Addit Contam. 33:1826–1839.
- Mulder PP, Klijnstra MD, Goselink RM, van Vuuren AM, Cone JW, Stoopen GM, Hoogenboom RL. 2020. Transfer of pyrrolizidine alkaloids from ragwort, common groundsel and viper’s bugloss to milk from dairy cows. Food Addit Contam. 37:1906–1921.
- Pinto S, Hoffmann G, Ammon C, Amon B, Heuwieser W, Halachmi I, Banhazi T, Amon T. 2019. Influence of barn climate, body postures and milk yield on the respiration rate of dairy cows. Ann Anim Sci. 19:469–481.
- Rutz L, Gao L, Küpper J-H, Schrenk D. 2020. Structure-dependent genotoxic potencies of selected pyrrolizidine alkaloids in metabolically competent HepG2 cells. Arch Toxicol. 94:4159–4172.
- Segall H. 1978. Pyrrolizidine alkaloids derived from Senecio jacobaea. Toxicol Lett. 1:279–284.
- Sharp W, Johnson R, Owens F. 1982. Ruminal VFA production with steers fed whole or ground corn grain. J Anim Sci. 55:1505–1514.
- Shimshoni JA, Mulder PP, Bouznach A, Edery N, Pasval I, Barel S, Abd-El Khaliq M, Perl S. 2015. Heliotropium europaeum poisoning in cattle and analysis of its pyrrolizidine alkaloid profile. J Agr Food Chem. 63:1664–1672.
- Snyder E, Credille B. 2017. Diagnosis and treatment of clinical rumen acidosis. Vet Clin: Food Anim Pract. 33:451–461.
- Stegelmaier B. 2004. Pyrrolizidine alkaloids. Part three. Classes of Toxicants. Clin Vet Toxicol Mosby Inc: St Louis, MO, USA. 370–377.
- Stojević Z, Piršljin J, Milinković-Tur S, Zdelar-Tuk M, Ljubić BB. 2005. Activities of AST, ALT and GGT in clinically healthy dairy cows during lactation and in the dry period. Veterinarski Arhiv. 75:67–73.
- Taenzer J, Gehling M, Klevenhusen F, Saltzmann J, Dänicke S, These A. 2022. Rumen metabolism of Senecio pyrrolizidine alkaloids may explain why cattle tolerate higher doses than monogastric species. J Agr Food Chem. 70:10111–10120.
- Torres MBA, Coelho KI. 2008. Experimental poisoning by Senecio brasiliensis in calves: quantitative and semi-quantitative study on changes in the hepatic extracellular matrix and sinusoidal cells. Pesq Vet Bras. 28:43–50.
- [VDLUFA] Verband Deutscher Landwirtschaftlicher Untersuchungs- und Forschungsanstalten. 2006. Handbuch der Landwirtschaftlichen Versuchs-und Untersuchungsmethodik (VDLUFA-Methodenbuch). Darmstadt: VDLUFA- Verlag, Bd. III Die chemische Untersuchung von Futtermitteln.
- Wiedenfeld H, Edgar J. 2011. Toxicity of pyrrolizidine alkaloids to humans and ruminants. Phytochem Rev. 10:137–151.
- Witte L, Ernst L, Adam H, Hartmannt T. 1992. Chemotypes of two pyrrolizidine alkaloid-containing Senecio species. Phytochem. 31:559–565.