ABSTRACT
The present investigation deals with wet chemical preparation and characterisation of copper (Cu) and cadmium sulphide (CdS) nanoparticles (NPs) (using UV–visible spectra, Fourier transform infra-red scattering, X-ray diffraction, dynamic light scattering, field emission scanning electron microscopy and transmission electron microscopy) and their effectivity on mitotic and meiotic cells of Nigella sativa L. (Ranunculaceae) in comparison to ethyl methanesulphonate (EMS) and gamma irradiations. The objective of the study is to foresee whether Cu- and CdS-NPs can induce similar type of chromosomal aberrations as that of EMS and gamma irradiations, or not. Dry seeds of N. sativa (2n = 12) are exposed to Cu- and CdS-NPs (0.25, 0.50 and 1.0 µg/ml; 3 and 6 h), EMS (0.25, 0.50 and 1.0%; 3 and 6 h) and doses of gamma irradiations (25, 50, 100, 200 and 300 Gy). Cu-NPs (range: 25.7 to 120.4 nm; 33.2 nm ± 9.6) and CdS-NPs (range: 29.4 to 115.7 nm; 37.8 nm ± 10.7) are both cubical to spherical in shape. NPs are found to induce similar responses as that of the studied conventional mutagens, in relation to physiological and chromosomal (mitotic and meiotic) attributes. Uptake of Cu- and CdS-NPs in seedlings is also studied using atomic absorption spectroscopy. Results suggest that Cu- and CdS-NPs can act as mutagenic agent, a pioneer report of its kind.
1. Introduction
Nanotechnology has global significance due to its wide application in industry, medicine, biotechnology, agriculture, different aspect of life sciences, among others.[Citation1–12] Nanoparticles (NPs – at least one dimension ranging between 1 and 100 nm – [Citation1]) possess unique physico-chemical properties,[Citation9,Citation10] which can be drastically modified compared to bulk materials.[Citation13] They are frequently released in the environment by natural processes and anthropogenic activities [Citation4,Citation14–18] effecting biological systems through cellular uptake and interactions. It raises debate in scientific community regarding risks and benefits associated to it.[Citation19] NPs are reported to exert both advantageous [Citation20–23] and adverse [Citation24–31] effects on higher plants. Physico-chemical properties of synthesised NPs, their size and surface area and doses employed are determining criteria for their bioactivity.[Citation9,Citation10,Citation32]
NPs are reported to induce cytotoxic and genotoxic effects on mitotic cells of different plant species.[Citation7,Citation8,Citation32–42] Till date, cytotoxic effects of NPs on meiotic cells are rather lacking. Effect on meiotic cells of plant species are expected to bring about genetical changes. Halder et al. [Citation11,Citation12] reported that copper (Cu) and cadmium sulphide (CdS) NPs induce stable and heritable phenotypic changes in Macrotyloma uniflorum (Lam.) Verdc (Family: Leguminosae). Such findings trigger the necessity to acquire further scientific knowledge from plant system on cytogenetical aspects relating to NP studies.
The present investigation describes the effects of synthesised Cu- (Cu2+ – metallic NPs, used as microfertilisers and pesticides – [Citation43,Citation44]) and CdS-NPs (semiconductor in nature, also known as quantum dots, narrow band gap of 2.5 eV – [Citation45]; used widely in biology, medicine, technology and telecommunication – [Citation46–48]) on mitotic and meiotic cells of Nigella sativa L. (common name: black cumin; Family: Ranunculaceae; spice-yielding plant of commerce possessing immense therapeutic uses – [Citation49] and cytological novelty [Citation50]), in comparison to conventional mutagens namely, ethyl methanesulphonate (EMS) and gamma irradiations. The objective of the work is to foresee whether the synthesised NPs can induce similar type of chromosomal aberrations as that of the studied conventional mutagens or not. If NPs able to do so, they can explore new dimension in induce mutagenesis. Study also encompasses characterisation of Cu- and CdS-NPs (using UV–visible spectra – UV–vis, Fourier transform infra-red spectroscopy – FTIR, X-ray diffraction – XRD, dynamic light scattering – DLS, field emission scanning electron microscopy – FESEM and transmission electron microscopy – TEM) for assessment of their nature and size.. Furthermore, uptake of NPs is studied in seedlings by atomic absorption spectroscopy (AAS).
2. Materials and methods
2.1. Germplasm
Seed samples (moisture content: 5.0%; seed size: length – 2.85 mm ± 0.11, breadth – 1.68 mm ± 0.03; seed colour: jet black – RAL 9005-010-010) of N. sativa L. (Family: Ranunculaceae) was obtained from medicinal plant garden, Narendrapur Ramkrishna Mission, Government of West Bengal, India.
2.2. Preparation of copper and cadmium sulphide nanoparticles
Cu- and CdS-NPs were prepared using wet chemical co-precipitation methods as adopted earlier by Chatterjee et al. [Citation51] and Halder et al., [Citation11] respectively. Cu-NPs was prepared using 8 ml (10%, w/v) gelatin (Merck, India), 0.5 ml of 0.1 M CuCl2 (SRL, India) and 1.5 ml Milli-Q water (0.22 µ, Millpore membrane), and the ingredients were thoroughly mixed. Concentrated ammonia (25%, Merck, India; 3 drops) and hydrazine hydrate (5 M; 10 drops) were added drop wise into the solution, stirred for 3 minutes and left undisturbed until the characteristic reddish brown colouration was developed. The suspension was filtered (Whatman® No. 42) and stored in an airtight capped container.
CdS-NPs were synthesised from drop-wise addition of freshly prepared Na2S (16 ml) solution into the bi-compound mixture (18:16) of cadmium acetate and sodium dodecyl sulphate (SDS) under continuous stirring.
Bulk Cu and CdS (0.25 µg/ml) were prepared from the source compound used in NPs preparations without the capping agents (Cu: gelatin; CdS: SDS).
2.3. Assessment of nanoparticles
Opto-physical study of synthesised Cu- and CdS-NPs was carried out using UV–vis, FTIR – only for CdS-NPs, XRD, DLS, FESEM and TEM.
2.3.1. UV–vis spectrophotometry
UV–vis absorption spectra of Cu-NPs suspension were recorded in the wavelength ranging between 400 and 600 nm in Shimadzu UVPC-1601 UV–vis spectrophotometer, in diffused reflection mode. Gelatin solution containing ammonia and hydrazine hydrate (preparation identical to Cu-NPs suspension) was used as reference at room temperature (22 ± 1 °C).
Ethanol mounted CdS-NPs were analysed using ethanol as blank. Absorption of visible spectrum was studied between 200 and 600 nm.
2.3.2. FTIR
The powdered CdS-NPs were diluted (1:100) in potassium bromide (IR grade) for assessment of diffused reflectance at infra-red spectrum between the range of 400 and 4000 cm−1 using FTIR analyser (Jasco FT/IR-6300 Fourier transform infrared spectrometer).
2.3.3. XRD
XRD patterns of powdered Cu- and CdS-NPs were calculated on Shimdzu LabX X-ray diffractometer using CuKα radiation (λ = 1.5406 Å) with 2θ ranging between 10° and 90° in steps of 0.02° and 1s per step sampling time. Crystallite size (Dnkl) was estimated using Debye–Scherrer equation, Dnkl = kλ/βcosθ; where λ = wavelength of CuKα radiation, β = full width of half maximum intensity, θ = diffraction angle in radian at diffraction peak and k = shape factor constant.
2.3.4. DLS
Average hydrodynamic diameters of both NPs were measured using DLS analyser (Delsa™ Nano C, Beckman Coulter).
2.3.5. FESEM and TEM
Particle surface ornamentation and surface morphology were studied using FESEM (JEOL JSM-7600F, resolution 1.0–1.5 nm) and TEM (JEOL JEM-2100HR, resolution 0.14–0.23 nm). For TEM study, 100 times diluted samples were dropped dried on a carbon-coated Cu grid (Applied Biosystems, India).
Bulk Cu and CdS were oven dried (≤60 °C for 4 h), and the obtained powdered samples were analysed under stereo microscope (Stereo Zoom® Leica S8 APO) for size determination.
2.4. Treatments
Dry seeds of N. sativa were exposed to Cu- and CdS-NPs (0.25, 0.50 and 1.0 µg/ml, 3 and 6 h durations), EMS (0.25, 0.50 and 1.0%, 3 and 6 h durations; solution prepared in 0.2 M phosphate buffer, 0.2 M KH2PO4 49.7ml: 0.2 M K2HPO4 50.3 ml, pH 6.8) and gamma irradiations (25, 50, 100, 200 and 300 Gy, source 60Co, absorbance dose rate 47.4 Gy/min, source to distance 12 cm). Dry control and bulk Cu- and CdS controls (0.25 µg/ml, 3 h) were also kept for assessment. In each lot of treatments, 100 seeds were exposed.
2.5. Accumulation of Cu- and CdS-NPs in seedlings
A weight of 0.45 g ash (10 days old seedlings, dried at 300 °C for 24 h) for each treatment, including bulk controls of Cu and CdS, was dissolved in 25 ml concentrated tri-acid (nitric acid:sulphuric acid:perchloric acid::3:3:1) solution and the volume was reduced to 5 ml by heating under a fume hood (80 °C). The concentrated solutions were cooled to room temperature (24 ± 1 °C). Finally, the solutions were diluted by using ddH2O up to 25 ml and filtered in a Whatman® filter paper (No. 42). The clear solutions (three replicas for each set) were analysed in a spectra AAS 240 (Agilent Technologies with 520 and 326.1 nm for Cu2+ and Cd2+ ions, respectively).
2.6. Assessment of seed germination and mitotic study
Fifty seeds from each lot of treatment along with controls (dry control and Cu and CdS control) were allowed to germinate in Petri plates lined with moist filter paper (22 ± 1 °C). Bursting of seed coat and emergence of radical were taken as an index of germination.
Six roots (about 2 mm length) from each control and treatments were cut, fixed in 1:3 acetic alcohol for overnight and preserved in 70% alcohol under refrigeration (16 ± 1 °C). For each treatment including control, three slides (replicas; randomly two roots were taken) were prepared from root tip squashes in 45% acetic acid and observed under Olympus trinocular light microscope (CH20i).
Mitotic index [(Mi) = (total number of diving cells/total cells) × 100], type of abnormalities in both dividing and resting cells and percentage of total aberrations in dividing cells were scored. Dose-wise representation of mitotic index and total frequency of abnormal dividing cells were graphically plotted for each treated materials (Cu- and CdS-NPs, EMS and gamma irradiations). Pooled data across the doses were tabulated. Photomicrographs were taken from suitable preparations.
2.7. Raising of plant population
Fifty seeds from each dose of treated materials including controls were sown in the experimental field plot of Department of Botany, University of Kalyani (West Bengal plain, Nadia, latitude 20°50′ to 24°11′ N, longitude 88°09′ to 88°09′ E, elevation 48 ft above sea level; sandy-loamy soil, organic carbon 0.76%, soil pH 6.85) in late November to raise the M1 plant population (15 cm between plants, 25 cm between rows). No fertiliser was applied during any stage of plant growth. The plants were harvested in mid-April.
2.8. Meiosis
For meiotic studies, floral buds of suitable sizes were fixed (5 a.m. to 6.30 a.m.) from individual plant of each treated dose (all surviving plants were scored) in acetic alcohol 1:3 for overnight and preserved in 70% alcohol under refrigeration. Pollen mother cells (PMCs) and pollen grains obtained from anther squash preparations were stained in 2% acetocarmine solution. Fully stained pollen grains were considered as fertile.[Citation52] Data were scored from scattered metaphase I (MI) and anaphase I (AI) meiocytes and also from anaphase II (AII) cells. Pooled data across the doses were tabulated. Photomicrographs were taken from suitable preparations.
Frequency of seedless plants was counted on harvest from treated doses and controls.
3. Results
3.1. Characterisation of nanoparticles
Optical absorbance of NP suspension exhibits characteristic plasmon resonance peak at 574 nm for Cu-NPs ((a)) and well-defined doublet exciton peak at 478 and 483 nm for CdS-NPs ((b)).
Figure 1. ( a–g) Characterisation of copper and cadmium sulphide nanoparticles. (a–b) UV--vis spectra of (a) Cu-NPs and (b) CdS-NPs. (c) FTIR spectra of CdS-NPs. (d–e) XRD patterns of powdered NPs ((d) Cu; (e) CdS). (f–g) DLS pattern of size distribution of (e) Cu-NPs and (f) CdS- NPs.
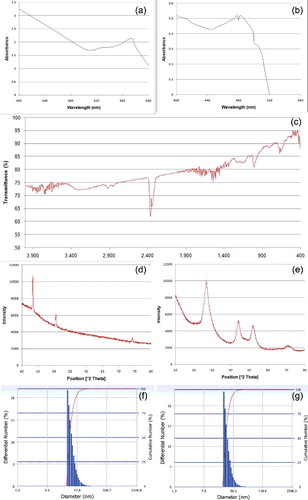
Multiple inversed absorption peak bands for CdS-NPs, using FTIR analyser, are recorded in the spectrum regions of 400 to 420, 580 to 750, 1000 to 1050, 2260 to 2390 and 3000 to 3500 cm−1 ((c)).
XRD pattern of powdered Cu-NPs shows characteristic sharp triplet peak at 43.3°, 51.5° and 74.6° ((d)) corresponding to the index of scattering form (1, 1, 1), (2, 0, 0) and (2, 0, 0) crystal faces, respectively. Prominent broad diffraction peaks of CdS-NPs are observed at 26.6°, 44.2° and 53.02° ((e)) and are considered as conformation of existence of (1, 1, 1), (2, 0, 0) and (3, 1, 1) planes, respectively. Average crystallite sizes of Cu- and CdS-NPs calculated from Scherrer equation are 43.08 nm ± 7.46 and 8.82 nm ± 0.91, respectively.
Hydrodynamic diameters of prepared Cu- and CdS-NPs analysed from diffused light scattering by particle Brownian motion are estimated to range from 25.7 to 120.4 nm (33.2 nm ± 9.6) ((f)) and 29.4 to 115.7 nm (37.8 nm ± 10.7) ((g)), respectively. Polydispersity indices are determined as 0.442 and 0.330 for Cu- and CdS-NPs, respectively.
Size of Cu- and CdS nanoclusters are determined from FESEM study and found to range from 220 to 800 nm ((a)) and 20 to 800 nm ((b)), respectively, with uneven surface topology.
Figure 2. FESEM ((a) Cu-NPs; (b) CdS-NPs) images showing nanoclusters and TEM ((c) Cu-NPs; (d) CdS-NPs) images of NPs (right-handed arrow – gelatin envelop; dotted arrow – metallic core).
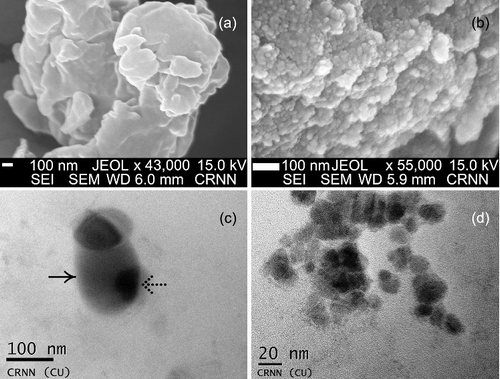
TEM image of Cu-NPs reveals parabolic to hyperbolic crystals ranging from 24 to 117 nm (41.7 nm ± 9.4) centred with 12 to 38 nm (26.2 nm ± 8.7) metallic core ((c)). The CdS NPs are spherical to cuboid in nature ((d)) with 30 to 80 nm size range (37.4 nm ± 8.5).
Bulk Cu (range: 2.47 to 80.0 µm; mean: 14.87 µm ± 8.7) and CdS (range: 1.77 to 50.0 µm; mean: 10.11 µm ± 5.9) clusters are much higher in sizes than the prepared NPs.
3.2. Accumulation of Cu- and CdS-NPs detected in the form of Cu2+ and Cd2+
Cu- and CdS-NPs accumulation (µg/g) in seedlings is mostly dose-dependent (). Cu2+ and Cd2+ accumulation are also observed in low amount in bulk materials.
Table 1. Accumulation Cu- and CdS-NPs in controls and treatments.
3.3. Petri plate germination frequency
As compared to controls (dry: 72%; bulk Cu control – 74%; bulk CdS control – 68%), Petri plate germination frequency is reduced mostly in all the doses of gamma irradiations (74% in 25 Gy to 12% in 300 Gy), EMS (66% in 0.25%, 6 h to 4% in 1.0%, 6 h), Cu-NPs (70% in 0.50 µg/ml, 6 h to 62% in 1.0 µg/ml, 3 h) and CdS-NPs (74% in 0.50 µg/ml, 6 h to 56% in 0.50 µg/ml, 3 h). Reduction in germination frequency is not dose-dependent and less affected in NP treatments than that of EMS and gamma irradiation.
3.4. Mitotic index and cytological aberration types and frequency
Mitotic index is 22% in both dry control and bulk Cu control and 18% in bulk CdS control. Compared to controls, significant (p < 0.05) reduction in the frequency of dividing cells is studied between/among doses (EMS: 11% to 5%, gamma irradiations: 18% to 8%, Cu-NPs: 11% to 6% and CdS-NPs: 22% to 7%) of treatments ((a)–3(g)). Reduction in mitotic index is not dose-dependent. The effect is pronounced (excepting CdS-NPs, 6 h duration) in higher concentrations and prolonged duration (6 h) of treatments.
Figure 3. ( a–g) Mitotic index and frequency of abnormal dividing cells in relation to respective controls of N. sativa. (a–b) Cu-NPs. (c–d) CdS-NPs. (e–f) EMS. (g) Gamma irradiations. (A) Frequency of abnormal cells; (B) mitotic index.
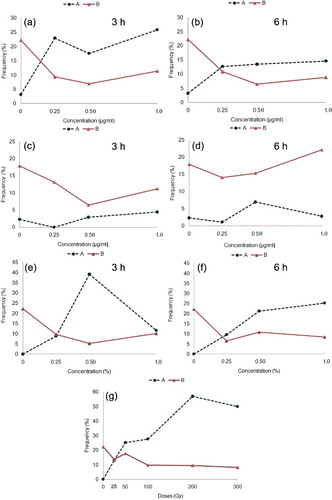
Mitotic cells at metaphase of untreated control show 2n = 12 chromosomes always ((a)). Bulk Cu- (3%) and CdS (2%) treatments show low frequency of metaphase cells with sticky and clumped configuration of chromosomes. Cytological aberrations studied in different treatments are diplochromatid nature ((c)), stickiness, ring configuration ((b)), fragmentation ((b), 4(d), 4(e)), polyploid cell formation ((d)), differential condensation ((e)), bridges with or without fragment(s) ((g) and 4(h)), laggards ((f)), micronuclei ((k)) and giant cell ((l)). Chromatin fragmentation ((j)), depletion of chromatin materials ((i)) and cell and nuclear shape deformities are also observed. Bridges are mostly single and paired. CdS-NPs (0.50 µg/ml, 6 h) treatment shows criss-cross bridge formation. Bridges with fragment(s) are noted in 200 Gy gamma irradiations (9%) and in 1.0 µg/ml, 3 h CdS-NPs (1%) treatments. Micronuclei (1–3) of variable sizes are observed in all treatments. Micronuclei are either condensed or uncondensed type.
Figure 4. ( a–h) Mitotic cells ((a–e) metaphase, (f–h) anaphase) of N. sativa in (a) control and (b–i) Cu- and CdS-NPs treated material. (a) 2n = 12. (b) Differentially condensed chromosome with a fragment (right-handed arrow) and a ring (dotted arrow). (c) Diplochromatid nature of chromosomes with unoriented chromosomes. (d) Cell with >2n = 12 chromosomes. (e) Differential condensation and fragmentation of chromosomes. (f) Anaphase with lagging fragments. (g) Chromosome bridge formation. (h) I: Anaphasic bridge with two equal size fragment, II: Double bridge with two minute fragments. (i) Cells without or with depleted chromatin. (j) Cell with fragmented chromatin mass. (k) Interphase cell with two unequal sized micronuclei. (l) Giant cell. Scale bar = 20 µm.
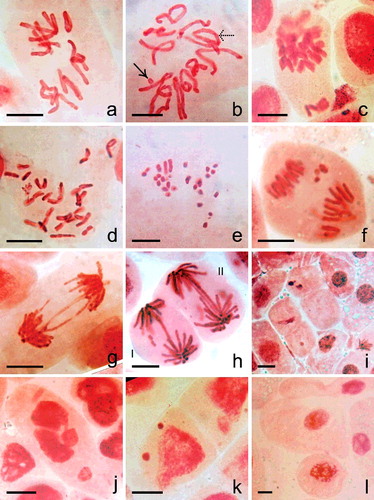
Total frequency of mitotic abnormalities is not dose-dependent and varied significantly (p < 0.05) between/among treatments (EMS: 9% to 39%, gamma irradiations: 13% to 57%, Cu-NPs: 13% to 26%, and CdS-NPs: 1% to 7%). Frequency of resting cells with micronuclei ranges from 1% to 2% in EMS, 1% to 17% in gamma irradiations, 0.5% to 3% in Cu-NPs and 1% to 7% in CdS-NPs. Polyploid cells with differential chromosome condensation (1%) are observed only in Cu-NPs treatments (0.50 and 1.0 µg/ml, 3 h; 0.25 µg/ml, 6 h).
Pooled data estimated across doses () in treatments in comparison to controls reveal significant (p < 0.05) variation for mitotic index, frequency of abnormal dividing cells, fragments, bridges and micronuclei. Across treated materials, the frequency of abnormal dividing cells and micronuclei are in the following order: gamma irradiations>EMS>Cu-NPs>CdS-NPs and gamma irradiations>CdS-NPs>EMS>Cu-NPs, respectively.
Table 2. Pooled data across doses of treated materials in root tip mitosis of N. sativa.
3.5. Field germination frequency and survivability
As compared to controls (dry – 40%; bulk Cu – 36%; bulk CdS – 42%), germination frequency is reduced mostly in treatments (EMS: 18% to 14%, gamma irradiations: 22% to 6%, Cu-NPs: 36% to 28%; CdS-NPs: 48% to 26%). Germination frequency is less affected in NP treatments than EMS and gamma irradiations. No germination of seeds could be recorded in 1.0%, 3 and 6 h EMS and 300 Gy gamma irradiations. Survivability of M1 plants is 33% in EMS, 67% to 100% in gamma irradiations, 46% to 100% in Cu-NPs and 40% to 100% in CdS-NPs.
3.6. Meiotic studies
Pooled meiotic configurations across doses of treatments at MI, AI and AII and pollen grain fertility are presented in . PMCs from control and treated plants mostly show 2n = 12 chromosomes ((a)). Few meiocytes in NP treated materials (0.50 µg/ml, 6 h CdS-NPs) show variation in chromosome number at MI (n = 7 – 0.86% – (j); n > 6 – 0.29% – (g)) and AI (2n = 24 – 0.46% – (s)). Compared to respective control (5.88II + 0.24I to 6II), mean chromosome association per cell at MI among treatments ranges between 5.64II + 0.49I and 5.96II + 0.07I. Bivalent and univalent frequency per cell assessed across controls and treatments are non-random (p < 0.001) in distribution as evinced from χ2 test of heterogeneity (DF 6). In controls (including bulk materials), AI (100.0%) and AII (100.0%) cells are cytologically balanced (6/6 – (n)) compared to treatments (AI: 72% to 96%, AII – 76% to 95%). Pollen grain fertility is reduced in treatments than controls (dry control – 96%; bulk Cu – 96%; bulk CdS – 90%; treated materials – 59% to 76%).
Table 3. Meiotic configurations and pollen fertility in treated materials.
Figure 5. Meiosis in (a) control and (b–x) nanoparticles treated plants. (a) 6II. (b) Pachytene stage with two fragments. (c) Diakinesis cell showing breakage of an arm (dotted arrow) in a bivalent. (d) MI with 5II + 2I + 3 fragments (right-handed arrow). (e–f) Chromosomal grouping (4II + 2II) at MI. (g) Fragments in association to bivalent and univalent at MI possibly 2n > 12. (h) Intense chromosomal fragmentation at MI. (i) Cytomictic behaviour involving two meiocytes. (j) Hyperploid PMC – 7II at MI. (k) Hypoploid cell with condensed type chromosomal fragments. (l) Agglutination of chromatin and fragments. (m) Differential condensation of chromosomes at MI. (n) 6/6 separation at AI. (o) 3/5/4 separation of chromosomes at AI. (p) 5/7 AI separation with interchromosomal connections. (q) 2n > 12 with sticky chromosomes at AI. (r) Dicentric chromatid bridge with an acentric fragment. (s) 2n = 24; AI showing tripolar organisation of chromosomes. (t) AII with one disorganised pole. (u) AII with bridge. (v) Unequal tripolarity. (w,x) AII with multiple groups and fragments. Scale bar = 10 µm.
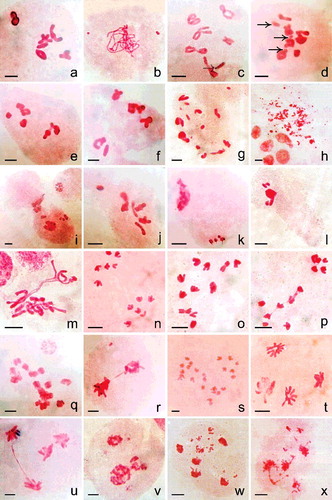
Abnormalities recorded in meiotic cells of treatments are sticky chromosomes, chromosomal fragments (1 to many – (b), 5(d), 5(g), 5(l)), lack of pairing between homologues, differential chromosome condensation ((m)), intense chromosomal fragmentation ((h)), aneuploid variation in chromosome number ((j) and 5(k)) and chromosomal grouping at MI ((e) and 5(f)); laggards (1–2), unequal separation of chromosomes (5/7 – (p) and 3/5/4 – (o), 4/8 and possibly >2n = 12 – (q)) and bridges with ((r)) or without a fragment at AI; tripolarity ((v)), unequal multipolar groups ((w) and 5(x)), disorganised groups of chromosomes ((t)), laggards and bridges at AII ((u)). EMS and NPs treatments predominantly show meiocytes with sticky chromosomes at MI. Chromosomal fragmentation and multipolarity are observed in gamma irradiation and in NPs treatments. Cytomixis ((i)) involving two PMCs (100 Gy gamma irradiations and 0.50 µg/ml, 6 h CdS-NPs; discrete in both cases) is also observed. PMCs squashes performed in a plant from 0.25 µg/ml, 3 h Cu-NPs treatment show total lack of microsporogenous tissues. Two meiocytes at pachytene (Cu-NPs: 1.0 µg/ml, 3 h) are with unequal sized fragments ((b)). A PMC at diakinesis is found with arm breakage ((c)). Relatively, gamma irradiations and NP treatments induce higher frequency of aberrations than EMS in meiotic cells.
After harvesting of all mature M1 plants, the frequency of seedless plants are counted as 0% in controls, 40% to 50% in gamma irradiations, 67% in EMS, 28% to 36% in Cu-NPs and 38% to 91% in CdS-NPs ((a)–6(d)).
4. Discussion
4.1. Characterisation of synthesised nanoparticles
Visible absorption of Cu-NPs showing prominent plasmon resonance peak at 574 nm confirms the presence of metallic Cu nanocrystallite in the suspension.[Citation53] Spectral analysis of CdS-NPs exhibiting double excitation peak along with higher absorption efficiency towards lower spectral series indicates blue-shifting of light absorption. Increase in absorption tendency of both NPs at high energy band spectrum signifies decreasing size of the NPs.[Citation54] Sizing effect noted for CdS-NPs is the consequence of photon energy generated electron-hole pairs confinement at conductance band of semiconductor NP.[Citation55]
Infra-red absorption of CdS-NPs indicates the chemical purity as well as compositional complexity of the synthesised NPs. Small and weak transmissional depression between 400 and 420 cm−1 establishes Cd–S bonding nature [Citation56,Citation57] of the NPs. Absorption band of 550 to 750 cm−1 is attributed to the crystalline disulphide (S–S) bond [Citation58] implying nanocrystal aggregates of CdS-NPs. Inverse peaks localised in 1000 to 1050, 2260 to 2390 and 3000 to 3500 cm−1 are distinguishing signal of ‘S–O’, ‘S–H’ bond and intermolecular H-bonds, respectively.[Citation59,Citation60] This explains the presence of lattice water as well as intermolecular water strongly attached to the surface of the CdS-NPs.
XRD pattern of Cu-NPs is well agreed with cubic nature of the metallic NP.[Citation12,Citation61,Citation62] Broad diffraction peaks of CdS-NPs also correspond with cubic phase confinement associated with decreasing size of quantum crystals.[Citation63] Baseline evenness in XRD along with low polydisperity index in DLS for both NPs signifies the purity and dispersity of the nanosuspensions. Size variation between DLS and XRD for the studied NPs is possibly due to progressive agglomerisation of the nanocrystals in the suspension. XRD shows efficiency in measuring nanograins even from the agglomerates [Citation64]; while, DLS only measures clustered grains in the suspension.[Citation65]
TEM micrographs of both metallic and semiconductor NPs nearly corroborate with the size measurement data obtained from DLS analysis. However, FESEM images of Cu- and CdS-NPs are not coinciding with the results of TEM and DLS as SEM measures the nanoclusters rather than individual crystalline grain. Size variation in NPs has been reported in assessment between SEM image and XRD method.[Citation66]
4.2. Mitotic and meiotic analyses
The present findings reveal that EMS, gamma irradiations and NPs cause cytotoxicity in mitotic cells by significantly (p < 0.001) reducing the frequency of dividing cells and inducing mitotic aberrations in relation to controls. Cytotoxic effects are reported earlier by conventional mutagens [Citation67–73] and NPs.[Citation8,Citation32,Citation33,Citation35,Citation36,Citation38,Citation74,Citation75] Nagaonkar et al. [Citation40] reported that Cu-NPs cause a gradual reduction in mitotic index with concomitant increase in mitotic abnormality in Allium cepa. D'Amato [Citation76] suggested that suppression of mitotic index was due to ‘prophase poisoning’. Sparrow et al. [Citation77] reported that mitotic inhibition was related to physiological disturbances.
Pooled mitotic data across doses of treated materials including controls reveal significant variations (p < 0.05) in mitotic index, total frequency of mitotic abnormalities and types of aberration. Assessment of mitotic data signifies that NPs can cause similar consequences as that of EMS and gamma irradiations. Cu-NPs show higher degree of cytotoxicity than CdS-NPs. Mitotic aberrations noted due to treatments can broadly be categorised as (1) stickiness of chromosomes caused due to depolymerisation of DNA, (2) chromosome breakages leading to formation of rings, bridges, fragments and micronuclei, (3) inhibition of centromeric division bringing about the formation of diplochromosomes and (4) discrepancies in spindle apparatus giving rise to polyploid cells and laggards. Frequency and spectrum of mitotic aberrations and the occurrence of micronuclei in resting cells are considered as important tools to assess sensitivity of plant species towards treating agents, and form an integral part of study on induced mutagenesis.
Similar types of meiotic abnormalities are studied in different treatments. NPs induce distinct chromosomal breakages and the effect is intense in few PMCs (Cu-NPs). Aneuploid variations in chromosome number are scored in a plant following NPs treatment. PMCs squash preparation of the same plant showed cytomixis and it is possibly the contributing factor behind the aneuploid variation noted. Pollen fertility is reduced in treatments as compared to controls which signifies possible hindrance exerted by the treated materials either during pollen formation or in developmental maturation process or in both.
Types of mitotic and meiotic aberrations induced by Cu- and CdS-NPs resemble that of the conventional mutagens like EMS and gamma irradiation. Present findings indicate that the studied NPs possess mutagenic potentiality. Several mitotic cycles lead to meiotic cell division. NPs inducing aberrations in meiotic cells are significant as persistence of such changes can bring about heritable alteration in the genotype. In the context, it is noteworthy to mention that present group of workers have already reported that Cu- and CdS-NPs can induce heritable phenotypic changes in M. uniflorum (Lam.) Verdc. [Citation11,Citation12]
Occurrence of seedless plants at M1 in all the treated materials and more so in Cu- and CdS-NPs is rather significant. Seed formation is a result of cumulative effects of many important processes like sporogenesis, pollination, fertilisation and embryogeny. Conventional mutagens as well as NPs have affected any or all the sequential processes of seed development reflecting the possibility of genetical changes in the formed seeds.
5. Conclusion
EMS [Citation78–80] and gamma irradiations [Citation81,Citation82] are effective mutagens that interacts with DNA to bring about mutational changes. NPs are also reported to interact with DNA in different plant species.[Citation32,Citation83–85] The present investigation highlights the potentiality of Cu- and CdS-NPs as mutagenic agents, a pioneer report of its kind.
Compliance with ethical standards
The research does not include any animals including human beings. Informed consent in this regard is not applicable.
Acknowledgments
The authors are thankful to the authority of Medicinal Plant Garden, Narendrapur Ramkrishna Mission, Government of West Bengal, India for generous supply of seed samples. The authors are also thankful to Centre for Research in Nanoscience & Nanotechnology (CRNN), University of Calcutta, West Bengal (for FESEM, TEM and DLS studies), UGC Consortium Centre, Kolkata (XRD analysis) and Department of Zoology, University of Kalyani (AAS analysis), for various help. The authors are thankful to anonymous reviewers for valuable suggestions.
Disclosure statement
No potential conflict of interest was reported by the authors.
Additional information
Funding
References
- Roco MC. Broader societal issues of nanotechnology. J Nanoparticle Res. 2003;5(3–4):181–189.
- Lam CW, James JT, McCluskey R, et al. Pulmonary toxicity of single-wall carbon nanotubes in mice 7 and 90 days after intratracheal instillation. Toxicol Sci. 2004;77(1):126–134.
- Caruthers SD, Wickline SA, Lanza GM. Nanotechnological applications in medicine. Curr Opin Biotechnol. 2007;18(1):26–30.
- Nowack B, Bucheli TD. Occurrence, behavior and effects of nanoparticles in the environment. Environ Pollut. 2007;150(1):5–22.
- Scrinis G, Lyons K. The emerging nano-corporate paradigm: nanotechnology and the transformation of nature, food and agri-food systems. Int J Sociol Agric Food. 2007;15(2):22–44.
- Singh M, Singh S, Prasada S, et al. Nanotechnology in medicine and antibacterial effect of silver nanoparticles. Digest J Nanomater Biostruct. 2008;3(3):115–122.
- Nair R, Varghese SH, Nair BG, et al. Nanoparticulate material delivery to plants. Plant Sci. 2010;179(3):154–163.
- Castiglione MR, Giorgetti L, Geri C, et al. The effects of nano-TiO2 on seed germination, development and mitosis of root tip cells of Vicia narbonensis L. and Zea mays L. J Nanoparticle Res. 2011;13(6):2443–2449.
- Remédios C, Rosário F, Bastos V. Environmental nanoparticles interactions with plants: morphological, physiological, and genotoxic aspects. J Bot. 2012; 2012(Article ID 751686):1–8. doi:10.1155/2012/751686.
- Masarovičová E, Kráľová K. Metal nanoparticles and plants. Ecol Chem Eng S. 2013;20(1):9–22.
- Halder S, Mandal A, Das D, et al. Effective potentiality of synthesised CdS nanoparticles in inducing genetic variation on Macrotyloma uniflorum (Lam.) Verdc. BioNanoSci. 2015;5(3):171–180.
- Halder S, Mandal A, Das D, et al. Copper nanoparticle induced macromutation in Macrotyloma uniflorum (Lam.) Verdc. (Family: Leguminosae): a pioneer report. Genet Resour Crop Evol. 2015;62(2):165–175.
- Nel A, Xia T, Mädler L, et al. Toxic potential of materials at the nanolevel. Science. 2006;311(5761):622–627.
- Biswas P, Wu CY. Nanoparticles and the environment. J Air Waste Manag Assoc. 2005;55(6):708–746.
- Buzea C, Pacheco II, Robbie K. Nanomaterials and nanoparticles: sources and toxicity. Biointerphases. 2007;2(4):MR17–MR71.
- Tervonen T, Linkov I, Figueira JR, et al. Risk-based classification system of nanomaterials. J Nanoparticle Res. 2009;11(4):757–766.
- Lidén G. The European commission tries to define nanomaterials. Ann Occup Hyg. 2011;55(1):1–5.
- Rico CM, Majumdar S, Duarte-Gardea M, et al. Interaction of nanoparticles with edible plants and their possible implications in the food chain. J Agric Food Chem. 2011;59(8):3485–3498.
- USEPA. Nanotechnology white paper; science policy council. Washington, DC: USEPA; 2007.
- Doshi R, Braida W, Christodoulatos C, et al. Nano-aluminium: transport through sand columns and environmental effects on plants and soil communities. Environ Res. 2008;106(3):296–303.
- Khodakovskaya M, Dervishi E, Mahmood M, et al. Carbon nanotubes are able to penetrate plant seed coat and dramatically affect seed germination and plant growth. ACS Nano. 2009;3(10):3221–3227.
- Sheykhbaglou R, Sedghi M, Shishevan MT, et al. Effects of nano-iron oxide particles on agronomic traits of soyabean. Not Sci Biol. 2010;2(2):112–113.
- Dhoke SK, Mahajan P, Kamble R, et al. Effect of nanoparticles suspension on the growth of mung (Vigna radiata) seedlings by foliar spray method. Nanotech Develop. 2013;3(e1):1–5. doi: 10.4081/nd.2013.e1.
- Lu CM, Zhang CY, Wen JQ, et al. Research of the effect of nanometer materials on germination and growth enhancement of Glycine max and its mechanism. Soybean Sci. 2002;21(3):168–171.
- Yang L, Watts DJ. Particle surface characteristics may play an important role in phytotoxicity of alumina nanoparticles. Toxicol Lett. 2005;158(2):122–132.
- Lin D, Xing B. Phytotoxicity of nanoparticles: inhibition of seed germination and root growth. Environ Pollut. 2007;150(2):243–250.
- Lee WM, An YJ, Yoon H, et al. Toxicity and bioavailability of copper nanoparticles to the terrestrial plants mung bean (Phaseolus radiatus) and wheat (Triticum aestivum) : plant agar test for water-insoluble nanoparticles. Environ Toxicol Chem. 2008;27(9):1915–1921.
- Stampoulis D, Sinha SK, White JC. Assay-dependent phytotoxicity of nanoparticles to plants. Environ Sci Technol. 2009;43(24):9473–9479.
- Ma YH, Kuang LL, Xiao H, et al. Effect of rare earth oxide nanoparticles on root elongation of plants. Chemosphere. 2010;78(3):273–279.
- Speranza A, Leopold K, Maier M, et al. Pd-nanoparticles cause increased toxicity to kiwifruit pollen compared to soluble Pd(II). Environ Pollut. 2010;158(3):873–882.
- Mushtaq YK. Effect of nanoscale Fe3O4, TiO2 and carbon particles on cucumber seedlings germination. J Environ Sci Health A Tox Hazard Subst Environ Eng. 2011;46(14):1732–1735.
- Kumari M, Mukherjee A, Chandrasekaran N. Genotoxicity of silver nanoparticles in Allium cepa. Sci Total Environ. 2009;407(19):5243–5246.
- Shaymurat T, Gu J, Xu C, et al. Phytotoxic and genotoxic effects of ZnO nanoparticles on garlic (Allium sativum L.): a morphological study. Nanotoxicology. 2012;6(3):241–248.
- Ghosh M, Sinha S JM, Chakraborty A, et al. In vitro and in vivo genotoxicity of silver nanoparticles. Mutat Res. 2012;749(1–2):60–69.
- Patlolla AK, Berry A, May L, et al. Genotoxicity of silver nanoparticles in Vicia faba: a pilot study on the environmental monitoring of nanoparticles. Int J Environ Res Public Health. 2012;9(5):1649–1662.
- Prokhorova IM, Kibrik BS, Pavlov AV, et al. Estimation of mutagenic effect and modifications of mitosis by silver nanoparticles. Bull Exp Biol Med. 2013;156(2):255–259.
- Abdel-Azeem EA, Elsayed BA. Phytotoxicity of silver nanoparticles on Vicia faba seedlings. New York Sci J. 2013;6(12):148–156.
- Abou-Zeid HM, Moustafa Y. Physiological and cytogenetic responses of wheat and barley to silver nanopriming treatment. Int J Appl Biol Pharma Technol. 2014;5(4):265–278.
- Raskar SV, Laware SL. Effect of zinc oxide nanoparticles on cytology and seed germination in onion. Int J Curr Microbiol App Sci. 2014;3(2):467–473.
- Nagaonkar D, Shende S, Rai M. Biosynthesis of copper nanoparticles and its effect on actively dividing cells of mitosis in Allium cepa. Biotechnol Prog. 2015;31(2):557–565.
- Rajeshwari A, Kavitha S, Alex SA, et al. Cytotoxicity of aluminum oxide nanoparticles on Allium cepa root tip–effects of oxidative stress generation and biouptake. Environ Sci Pollut Res Int. 2015;22(14):11057–11066.
- Taranath TC, Patil BN, Santosh TU, et al. Cytotoxicity of zinc nanoparticles fabricated by Justicia adhatoda L. on root tips of Allium cepa L.–a model approach. Environ Sci Pollut Res Int. 2015;22(11):8611–8617.
- Selivanov VN, Zorin EV. Sustained action of ultrafine metal powders on seeds of grain crops. Perspekt Materialy. 2001;4:66–69.
- Raikova OP, Panichkin LA, Raikova NN. Studies on the effect of ultrafine metal powders produced by different methods on plant growth and development. Nanotechnologies and Information Technologies in the 21st Century. In Proceedings of the International Scientific and Practical Conference; pp. 108–111; Moscow, Russia, 2006.
- Khataee A, Movafeghi A, Nazari F, et al. The toxic effects of L-Cysteine-capped cadmium sulfide nanoparticles on the aquatic plant Spirodela polyrrhiza. J Nanoparticle Res. 2014;16:(2774):1–10.
- Medintz IL, Uyeda HT, Goldman ER, et al. Quantum dot bioconjugates for imaging, labelling and sensing. Nat Mater. 2005;4:435–446.
- Michalet X, Pinaud FF, Bentolila LA, et al. Quantum dots for live cells, in vivo imaging, and diagnostics. Science. 2005;307(5709):538–544.
- Shen YJ, Lee YL. Assembly of CdS quantum dots onto mesoscopic TiO2 films for quantum dot-sensitized solar cell applications. Nanotechnology. 2008;19(4):1–7.
- Datta AK, Saha A, Bhattacharya A, et al. Black cumin (Nigella sativa L.) – a review. J Plant Dev Sci. 2012;4(1):1–43.
- Saha A, Datta AK. Gamma-rays induced reciprocal translocation in black cumin (Nigella sativa L.). Cytologia. 2002;67(4):389–396.
- Chatterjee AK, Sarkar RK, Chattopadhyay AP, et al. A simple robust method for synthesis of metallic copper nanoparticles of high antibacterial potency against E. coli. Nanotechnology. 2012;23(8):085103.
- Marks GE. An aceto-carmine glycerol jelly for use in pollen-fertility counts. Stain Technol. 1954;29(5):277.
- Lisiecki I, Pileni MP. Synthesis of copper metallic clusters using reverse micelles as microreactors. J Am Chem Soc. 1993;115(10):3887–3896.
- Domracheva NE, Vorobeva VE, Gruzdev MS, et al. Blue shift in optical absorption, magnetism and light-induced superparamagnetism in γ -Fe2O3 nanoparticles formed in dendrimer. J Nanoparticle Res. 2015;17:(83):1–8.
- Stroyuk AL, Kryukov AI, Kuchmii SY, et al. Quantum size effects in the photonics of semiconductor nanoparticles. Theor Exp Chem. 2005;41(2):67–91.
- Lu X, Gao H, Chen J, et al. Poly(acrylic acid)-guided synthesis of helical polyaniline/CdS composite microwires. Nanotechnology. 2005;16:113–117.
- Lu X, Yu Y, Chen L, et al. Preparation and characterization of polyaniline microwires containing CdS nanoparticles. Chem Commun. 2004;2004(13):1522–1523.
- Khiew PS, Huang NM, Radiman S, et al. Synthesis and characterization of conducting polyaniline-coated cadmium sulphide nanocomposites in reverse microemulsion. Mat Lett. 2004;58(3–4):516–521.
- Lee HL, Mohammed IA, Belmahi M, et al. Thermal and optical properties of CdS nanoparticles in thermotropic liquid crystal monomers. Materials. 2010;3(3):2069–2086.
- Bagabas A, Alshammari A, Aboud MFA, et al. Room-temperature synthesis of zinc oxide nanoparticles in different media and their application in cyanide photodegradation. Nanoscale Res Lett. 2013;8(1/516):1–10.
- Patel NH, Deshpande MP, Bhatt SV, et al. Structural and magnetic properties of undoped and Mn doped CdS nanoparticles prepared by chemical co-precipitation method. Adv Mat Lett. 2014;5(11):671–677.
- Zhang AQ, Tan QZ, Li HJ, et al. pH-dependent shape changes of water-soluble CdS nanoparticles. J Nanoparticle Res. 2014;16(Article id 2197):1–13.
- Prabhu RR, Khadar MA. Characterization of chemically synthesized CdS nanoparticles. Pramana. 2005;65(5):801–807.
- Dieckmann Y, Cölfen H, Hofmann H, et al. Particle size distribution measurements of manganese-doped ZnS nanoparticles. Anal Chem. 2009;81(10):3889–3895.
- Liu HH, Surawanvijit S, Rallo R, et al. Analysis of nanoparticle agglomeration in aqueous suspensions via constant-number monte carlo simulation. Environ Sci Technol. 2011;45(21):9284–9292.
- Mercy A, Selvaraj RS, Boaz BM, et al. Synthesis, structural and optical characterisation of cadmium sulphide nanoparticles. Indian J Pure Appl Phys. 2013;51(6):448–452.
- Datta AK, Biswas AK. X-ray sensitivity in Nigella sativa L. Cytologia. 1983;48(2):293–303.
- Datta AK, Biswas AK, Sen S. Gamma radiation sensitivity in Nigella sativa L. Cytologia. 1986;51(3):609–615.
- Kumar S, Dubey DK. Effect of gamma rays, EMS and dES on meiosis in Lathyrus sativus. J Cytol Genet. 1998;33:139–147.
- Rang S, Datta AK. Influence of some physical and chemical factors on gamma radiation sensitivity in Nigella sativa L. (black cumin). J Natl Bot Soc. 1998;52:17–22.
- Datta SK, Chakrabarty D, Verma AK, et al. Gamma ray induced chromosomal aberrations and enzyme related defense mechanism in Allium cepa L. Caryologia. 2011;64(4):388–397.
- Mukherjee S, Datta AK. Mitotic and meiotic consequences of gamma irradiations on dry seeds of Nigella sativa L. (black cumin). J Plant Dev Sci. 2011;3(3–4):233–238.
- Kamble GC, Patil AS. Comparative mutagenicity of EMS and gamma radiation in wild chickpea. Int J Sci Environ Technol. 2014;3(1):166–180.
- Babu K, Deepa MA, Shankar SG, et al. Effect of nano-silver on cell division and mitotic chromosomes: A prefatory siren. Int J Nanotechnol. 2008;2(2):1–7.
- Corneanu M, Corneanu G, Ardelean A, et al. The radiobiological effect of the TiO2 – cyclodextrin suspension. Paper presented at: The Fifth International Conference on Quantum, Nano and Micro Technologies; 2011, August 21–27; Nice/Saint Laurent du Var, France.
- D'Amato F. The cytological study of chemical mutagens. Genet Iber. 1952;4:3–20.
- Sparrow AH, Mosses MJ, Steel R. A cytological and cytochemical approach to an understanding of radiation damage in dividing cells. Brit J Radiol. 1952;25(292):182–188.
- Alexander P, Stacey KA. Comparison of the changes produced by ionizing radiations and by the alkylating agents: evidence for a similar mechanism at the molecular level. Ann Acad Sci. 1958;68(3):1225–1237.
- Stacey KA, Cobb M, Concens SF, et al. The reactions of the radiomimetic alkylating agents with macromolecules in vitro. Ann Acad Sci. 1958;68(3):682–701.
- Freese E. Molecular mechanism of mutations. In: Hollaender A, editor. Chemical Mutagens. New York: Plenum Press; 1971. p.1–56.
- Koller PC. The cytological effects of irradiation at low intensities. J Heredity. 1953;6:5–22.
- Lea DE. Action of radiations on living cells. 2nd ed. Cambridge: Cambridge Univ. Press; 1955.
- Ghosh M, Bandyopadhyay M, Mukherjee A. Genotoxicity of titanium dioxide (TiO2) nanoparticles at two trophic levels: plant and human lymphocytes. Chemosphere. 2010;81(10):1253–1262.
- Rodriguez E, Azevedo R, Fernandes P, et al. Cr(VI) induces DNA damage, cell cycle arrest and polyploidization: a flow cytometric and comet assay study in Pisum sativum. Chem Res Toxicol. 2011;24(7):1040–1047.
- Atha DH, Wang H, Petersen EJ, et al. Copper oxide nanoparticle mediated DNA damage in terrestrial plant models. Environ Sci Technol. 2012;46(3):1819–1827.