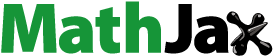
ABSTRACT
Ag nanoparticles (Ag NPs) with antibacterial properties were synthesized by using a one-step method. The reagents used were environmentally friendly, and no subsequent purification treatment was required. This method conformed to the concept of green chemistry, and the synthesized silver nanoparticles had biosafety and antibacterial properties. The structure of Ag NPs was investigated and characterized by X-ray diffraction (XRD), X-ray photoelectron spectroscopy (XPS), and transmission electron microscopy (TEM). The particle size of Ag NPs was approximately 14.01 nm, and the particle size distribution was relatively uniform. The antibacterial properties of Ag NPs were studied by conducting an inhibition circle experiment and investigating the growth curve. Lignin-capped Ag NPs (L-Ag NPs) were synthesized and characterized as inorganic antibacterial agents with good antibacterial activity and no biological toxicity.
GRAPHICAL ABSTRACT
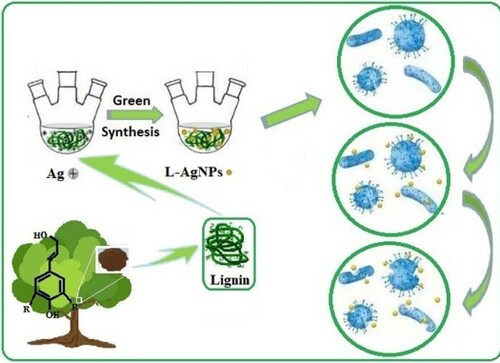
1. Introduction
To comply with the general principles of green chemistry, significant effort has recently been devoted to the synthesis of metal NPs using plants extracts as reducing agents (Citation1–3). These methods are eco-friendly and cost-effective ways to prepare metal nanocomposites with antibacterial activity. Plants contain a variety of phytochemicals, including soluble carbohydrates, phenolic acids, alkaloids, flavonoids, and terpenoids(Citation4,Citation5). These phytochemicals can be used as reducing agents and stabilizers to prepare metal NPs, including Ag NPs, which can be used as antibacterial agents(Citation6). Lignin is among the most abundant renewable natural resources in the world. It has received much research attention because of its good biodegradability (Citation7). Lignin contains a large number of active hydroxyl, aldehyde, and other reducing groups and has a special 3D structure (Citation8,Citation9). Therefore, lignin can be used as a green capping agent and dispersant of metal NPs (Citation10,Citation11). Such utilization of lignin significantly improves its application value. In addition, lignin is conducive to environmental protection and the utilization of biological resources. Thus, its use has a positive theoretical and practical significance.
Viruses and bacteria are widely distributed in nature. They spread rapidly, thereby seriously threatening the health and safety of humans. The development of effective antimicrobial agents and their application to daily life are conducive to controlling the spread of viruses and bacteria (Citation12). In recent years, the antibacterial properties of metal oxides, which are produced through the emergence of resistant strains to various organic antimicrobial agents, have received considerable attention. However, metal nanomaterials have stronger antibacterial activity than the same materials with large particles (Citation13). Silver nanoparticles (Ag NPs) are nontoxic, biosafe, and biocompatible antimicrobial agents that have been applied to many products, including drug carriers, cosmetics, medical materials, and household products (Citation14,Citation15). Ag NPs are powerful adsorbents that destroy the biochemical pathways of microorganisms through various pathways, e.g. the formation of reactive oxygen species (ROS) and the interruption of electron transfer reactions (Citation16,Citation17). In recent years, several reviews have reported the physicochemical properties and antibacterial activity of metal nanoparticles, such as Ag NPs (Citation18–21).
Sodium lignosulfonate, which is the main form of lignin, was used as a reactant, whereas ascorbic acid was used as the reducing agent. L-AgNPs were synthesized through a one-step method, and no other chemical reagents were needed. The reaction conditions were mild and simple, and high purity L-AgNPs can be obtained without purification (Citation22,Citation23). The prepared L-AgNPs were nontoxic and had strong antibacterial properties.
2. Experimental
2.1. Materials
AgNO3 and ascorbic acid were obtained from the Tianjin Beichen reagent factory. Sodium lignosulfonate was purchased from Shanghai McLean Biochemical Technology Co., Ltd.. Peptone, yeast extract, and agar were procured from Shandong Yubao Biotechnology Co., Ltd. Candida albicans (ATCC10231) and Escherichia coli (ATCC25922) strains were incubated for 24 h at 37 °C. All reagents were analytically pure and did not require further processing.
The equipment used in the experiment included a UV-visible 2550 ultraviolet spectrophotometer (Shimadzu, Japan), a D8 Venture X-ray single crystal diffractometer (Brooke, Germany), and HT7800 transmission electron microscope (Hitachi, Japan).
2.2. Preparation of L-AgNPs
Sodium lignosulfonate (0.30 g) was accurately weighed, placed in a round-bottomed flask, and dissolved in 30 mL of water. The solution was mixed under magnetic force at room temperature for 3 min. Ascorbic acid (0.03 g) was weighed and dissolved in 30 mL distilled water before being added dropwise to 30 mL of 0.01 mol/L silver nitrate solution until the solution was completely dissolved by ultrasound. The solution was heated in a water bath at 37 °C for 5 h.
2.3. Bacteriostatic circle test
Several sterile qualitative filter paper discs (6 mm in diameter) were prepared along with distilled water, 10 mg/mL lignin solution, 10 mg/mL L-AgNPs, 1 mg/mL L-AgNPs, and 0.1 mg/mL L-AgNPs. The filter paper discs were soaked in five samples, removed after 10 min, sterilized with high-pressure steam, and dried.
A liquid culture medium was then prepared using 6 g peptone, 3 g yeast extract, 6 g NaCl, 16 g agar, and 600 mL of distilled water. The pH value was adjusted from 7.2–7.4 by using a 6 M sodium hydroxide solution. The solution was placed in a high-pressure steam sterilization pot, sterilized at 120 °C for 30 min, and removed for cooling. C. albicans and E. coli were then added into the liquid culture medium to produce a solid culture medium. Five filter paper discs containing the samples were pasted on each culture dish. Each dish was placed in a constant temperature incubator at 37 °C for 24 h. The growth of the bacteriostatic circle was then observed.
2.4. Cell viability assay
The cytotoxicity of L-AgNPs was examined by cell-counting kit-8 (CCK-8, Dojindo Laboratories, Japan). The A549 cells were seeded in 96-well plates at a density of 4000 cells mL−1. After 24 h of incubation, the medium was replaced by a new one containing L-AgNPs at various concentrations (0, 1×10−7, 1×10−6, 1×10−5, 1×10−4, 1×10−3, 1×10−2, and 1×10−1 μg/mL). The cells were incubated for another 24 h. Freshly prepared CCK-8 (10 μL) solution in culture medium (90 μL) was added to each well. After 1 h to 4 h of incubation, the CCK-8 medium solution was carefully removed. The plate was gently shaken for 5 min at room temperature, and the optical density (OD) of the mixture was measured at 450 nm. Cell viability was assessed by using the following equation:
3. Results and discussion
3.1. L-AgNP preparation
L-AgNPs are prepared with sodium lignosulfonate as reactant and ascorbic acid as reducing agent. The reaction process was optimized. The effects of reaction time, reaction temperature, and the amount of ascorbic acid were investigated. As shown in a, during L-AgNP preparation, the absorption peak intensity of L-AgNPs increased with increasing temperature. The absorption peak intensity was strongest at 40 °C. Therefore, 37 °C was selected as the reaction temperature. With increasing reaction time (b), the absorption peak intensity of L-AgNPs strengthened and stabilized after 5 h. Thus, the chosen reaction time was 5 h. c shows that no reaction was observed when ascorbic acid was not added. The optimum amount of ascorbic acid was 1 mg/mL. Through the optimization of reaction conditions, the optimal reaction conditions were determined to be as follows: temperature of 37 °C, synthesis time of 5 h, and reductant dosage of 1 mg/mL.
3.2. L-AgNP characterization
a shows the UV-vis spectrogram of the prepared L-AgNPs. The maximum absorption wavelength of the L-AgNPs was 420 nm, which was consistent with the results reported in the literature (Citation24). The typical isotherms for nitrogen (N2) adsorption of the samples are shown in b. The adsorption isotherm of L-AgNPs was a typical type III adsorption isotherm. The L-AgNP sample had a specific surface area of 18.7537 m2/g.
The TEM of L-AgNPs is shown in . The particles of L-AgNPs were spherical and well dispersed. The particle size distribution diagram was used to calculate the particle size of approximately 14.01 nm, and the particle size distribution was relatively uniform. As shown in b, the selected area electron diffraction of L-AgNPs had evident symmetrical diffraction spots, and its lattice fringes were clearly observed. The calculated lattice spacing was 0.238 nm. The crystal surface spacing was very close to 0.2359 nm of the (111) crystal surface spacing of Ag. TEM results showed that Ag NPs were successfully synthesized.
presents an X-ray diffraction (XRD) diagram of pure lignin and L-AgNPs. a shows the peaks at 2θ = 38.12° (111), 44.2° (200), 64.43°(220), 77.47° (311), which are consistent with the standard crystal silver structure (Ag PDF#04-0783) (Citation25). These Bragg reflections corresponded to the crystalline planes of the face-centered cubic crystal lattice of metallic silver. However, lignin does not contain these diffraction peaks. The diffraction peaks after the synthesis of L-AgNPs showed that high purity Ag NPs were successfully prepared by this method. To further study the crystal structure of L-AgNP samples, Jade 9.0 software was used to perform a full spectrum fitting of the XRD data (b). The doped lattice parameters obtained by the fitting refinement were as follows: a = 4.09366, b = 4.09366, c = 4.09366; atomic positions α = 90°, β = 90°, and γ = 90°. L-AgNPs were an orthorhombic crystal system. The space group was Fm3 m (225), and the criterion of the fit was a residual factor Rp = 1.12% and a weighted residual variance Rwp = 2.77%. The values were less than 10%, indicating that the experimental value fitted the theoretical value. The XRD results of L-AgNPs were consistent with their crystal data simulation results, thereby confirming that the compound had a pure phase.
a shows the XPS full spectrum scanning spectrogram of L-AgNPs. This finding showed that the main elements in L-AgNPs were C, O, and Ag. b shows the peaks of C1s (284.8 and 286.3 eV) from left to right. These peaks represented the carbon atoms of aliphatic (C-C) and carboxyl (-C-OH) groups in lignin. The peaks at 288.2 and 289.5 eV represented sodium carbonate and bicarbonate, respectively (Citation26). c shows the peak of O1s in L-AgNPs. The peaks at 531 and 533.5 eV were attributed to C-O and hydroxyl (-C-OH) in lignin, and Na KLL was found at 536 eV (Citation27). In addition to C and O elements, an abundance of Ag was observed. d shows the peak diagram, where 367.8 and 373.9 eV represented Ag3d5/2 and Ag3d3/2, respectively. Their splitting energies were 5.7 eV. The peaks at 371.8 and 376.6 eV were attributed to the high binding energy loss peaks of the components in the silver spin orbit, indicating that the Ag in the sample was almost Ag (0). The results showed that Ag NPs were successfully synthesized (Citation28).
3.3. Antibacterial properties of L-AgNPs
The filter paper soaked in the L-AgNP solution was placed in a solid medium, and the antibacterial activity of these NPs was tested with the use of an antibacterial ring. Gram-positive C. albicans and Gram-negative E. coli were selected as antibacterial targets (), and the antibacterial properties of L-AgNPs were evaluated. As shown in a and b, the bacteriostatic circles of L-AgNPs to E. coli and C. albicans was evident after incubation at 37 °C for 24 h. a shows that L-AgNPs had good inhibitory effect on E. coli. In accordance with the amount of L-AgNPs added (0.1–10 mg/mL), the diameters of the inhibition zones were 14 ± 1, 16 ± 1, and 18 ± 1 mm. b shows the bacteriostatic circle of L-AgNPs to C. albicans. In accordance with the amount of L-AgNPs added (0.1–10 mg/mL), the diameters of the inhibition zones were 12 ± 1, 14 ± 1, and 20 ± 1 mm. shows bacteriostatic circles, thereby indicating that L-AgNPs had strong inhibitory effects on E. coli and C. albicans (Citation29,Citation30).
Figure 6. a: Inhibition circles of L-AgNPs against E.coli; b: Inhibition circles of L-AgNPs against C. albicans.
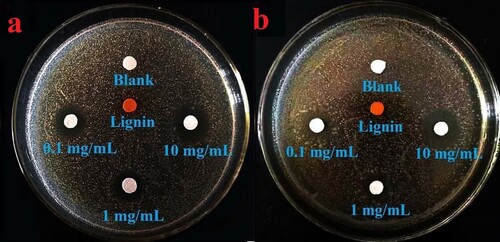
To observe the inhibitory effect of L-AgNPs on E. coli and C. albicans, the growth curves of L-AgNPs in 36 h were measured. As shown in , with prolonged culture time, the colony numbers of E. coli and C. albicans gradually decreased, and the curve showed a downward trend. This finding showed that L-AgNPs had an evident inhibitory effect on both strains, and such effect increased with time. The colony numbers of E. coli tended to be stable after 20–24 h, thereby indicating that the L-AgNPs inhibited the growth of E. coli in 24 h. L-AgNPs inhibited the growth of C. albicans in approximately 15 h (Citation31).
3.4. Biological toxicity of L-AgNPs
We tested the biological toxicity of L-AgNPs to reveal the feasibility of L-AgNPs as antibacterial agents. We used A549 cells as a biological experimental model. The cytotoxicity of L-AgNPs was detected by CCK-8. shows that almost 98% of A549 cells were still alive when exposed to L-AgNPs. Thus, L-AgNPs have very low cytotoxicity and are suitable as antibacterial agents (Citation32).
4. Conclusions
L-AgNPs were prepared by using a simple and green chemical method. Structural analysis has proven that the prepared L-AgNPs have uniform size distribution and high purity. The antibacterial experiment on E. coli and C. albicans proved that L-AgNPs had good antibacterial properties, while CCK8 experiment showed that L-AgNPs were biologically non-toxic and could be used as antibacterial materials in life.
Disclosure statement
No potential conflict of interest was reported by the author(s).
Additional information
Funding
References
- Ahmed, S.; Ahmad, M.; Swami, B.L.; Ikram, S. A Review on Plants Extract Mediated Synthesis of Silver Nanoparticles for Antimicrobial Applications: A Green Expertise. J. Adv. Res 2016, 7, 17–28.
- Ahmed, M.K.; Menazea, A.A.; Mansour, S.F.; Al-Wafi,, R. Differentiation Between Cellulose Acetate and Polyvinyl Alcohol Nanofibrous Scaffolds Containing Magnetite Nanoparticles/Graphene Oxide via Pulsed Laser Ablation Technique for Tissue Engineering Applications. J. Mater. Res. Technol 2020, 9, 11629–11640.
- Kuppusamy, P.; Yusoff, M.M.; Maniam, G.P.; Govindan, N. Biosynthesis of Metallic Nanoparticles Using Plant Derivatives and Their new Avenues in Pharmacological Applications–An Updated Report. Saudi. Pharma. J 2016, 24, 473–484.
- Aisida, S.O.; Ugwu, K.; Akpa, P.A.; Nwanya, A.C.; Ejikeme, P.M.; Botha, S.; Ahmad, I.; Maaza, M.; Ezema, F.I. Biogenic Synthesis and Antibacterial Activity of Controlled Silver Nanoparticles Using an Extract of Gongronema Latifolium. Mater. Chem. Phys 2019, 237, 121859.
- Aisida, S.O.; Ugwu, K.; Akpa, P.A.; Nwanya, A.C.; Nwankwo, U.; Botha, S.; Ejikeme, P.M.; Ahmad, I.; Maaza, M.; Ezema, F.I. Biosynthesis of Silver Nanoparticles Using Bitter Leave (Veronica amygdalina) for Antibacterial Activities. Surf. Interfaces 2019, 17, 100359.
- Menazea, A.A.; Ismail, A.M.; Awwad,, N.S.; Ibrahium,, H.A. Physical Characterization and Antibacterial Activity of PVA/Chitosan Matrix Doped by Selenium Nanoparticles Prepared via one-pot Laser Ablation Route. J. Mater. Res. Technol 2020, 9, 9598–9606.
- Ahmed, M.K.; Meera Moydeen, A.; Ismail, A.M.; El-Naggar,, M.E.; Menazea, A.A.; El-Newehy,, M.H. Wound Dressing Properties of Functionalized Environmentally Biopolymer Loaded with Selenium Nanoparticles. J. Mol. Struct 2021, 1225, 129138.
- Navarro Gallon, S.M.; Alpaslan, E.; Wang, M.; Larese-Casanova, P.; Londono, M.E.; Atehortua, L.; Pavon, J.J.; Webster, T.J. Characterization and Study of the Antibacterial Mechanisms of Silver Nanoparticles Prepared with Microalgal Exopolysaccharides. Mater. Sci. Eng. C 2019, 99, 685–695.
- Sakagami, H.; Kushida, T.; Oizumi, T.; Nakashima, H.; Makino, T. Distribution of Lignin-Carbohydrate Complex in Plant Kingdom and its Functionality as Alternative Medicine. Pharm. Ther 2010, 128, 91–105.
- Liu, Y.R.; Nie, Y.; Lu, X.M.; Zhang, X.P.; He, H.Y.; Pan, F.J.; Zhou, L.; Liu, X.; Ji, X.Y.; Zhang, S.J. Cascade Utilization of Lignocellulosic Biomass to High-Value Products. Green Chem. 2019, 21, 3499–3535.
- Marulasiddeshwara, M.B.; Dakshayani, S.S.; Sharath Kumar, M.N.; Chethan, R.; Raghavendra Kumar, P.; Devaraja, S. Facile-one pot-Green Synthesis, Antibacterial, Antifungal, Antioxidant and Antiplatelet Activities of Lignin Capped Silver Nanoparticles: A Promising Therapeutic Agent. Mater. Sci. Eng. C 2017, 81, 182–190.
- Menazea, A.A.; Abdelghany, A.M. Precipitation of Silver Nanoparticle Within Silicate Glassy Matrix via Nd:Yag laser for Biomedical Applications. Radiat. Phys. Chem 2020, 174, 108958.
- Roselli, M.; Finamore, A.; Garaguso, I.; Britti, M.S.; Mengheri, E. Zinc Oxide Protects Cultured Enterocytes from the Damage Induced by Eschrichia Coli. J. Nutr 2003, 133, 4077–4082.
- Hazarika, Z.; Jha, A.N. Computational Analysis of the Silver Nanoparticle-Human Serum Albumin Complex. ACS Omega 2020, 5, 170–178.
- Pugazhendhi, A.; Prabakar, D.; Jacob, J.M.; Karuppusamy, I.; Saratale, R.G. Synthesis and Characterization of Silver Nanoparticles Using Gelidium Amansii and its Antimicrobial Property Against Various Pathogenic Bacteria. Microb. Pathog 2018, 114, 41–45.
- Ahn, J.M.; Eom, H.J.; Yang, X.; Meyer, J.N.; Choi, J. Comparative Toxicity of Silver Nanoparticles on Oxidative Stress and DNA Damage in the Nematode, Caenorhabditis Elegans. Chemosphere 2014, 108, 343–352.
- Maurer, L.; Meyer, J. A Systematic Review of Evidence for Silver Nanoparticle-Induced Mitochondrial Toxicity. Environ. Sci. Nano 2016, 3, 311–322.
- Johnston, H.J.; Hutchison, G.; Christensen, F.M.; Peters, S.; Hankin, S.; Stone, V. A Review of the in Vivo and in Vitro Toxicity of Silver and Gold Particulates: Particle Attributes and Biological Mechanisms Responsible for the Observed Toxicity. Critical. Rev. Toxicol 2010, 40, 328–346.
- Lemire, J.A.; Harrison, J.J.; Turner, R.J. Antimicrobial Activity of Metals: Mechanisms, Molecular Targets and Applications. Nat. Rev. Microbiol 2013, 11, 371–384.
- Prabhu, S.; Poulose, E.K. Silver Nanoparticles: Mechanism of Antimicrobial Action, Synthesis, Medical Applications, and Toxicity Effects. Int. Nano. Lett 2012, 2, 32.
- Tran, Q.H.; Le, A.T. Silver Nanoparticles: Synthesis, Properties, Toxicology, Applications and Perspectives. Adv. Nat. Sci. Nanosci. Nanotechnol 2013, 4, 033001.
- Barapatre, A.; Aadil, K.R.; Jha, H. Synergistic Antibacterial and Antibiofilm Activity of Silver Nanoparticles Biosynthesized by Lignin-Degrading Fungus. Bioresour. Bioprocess 2016, 3, 8.
- Zhong, J.F.; Xu, L.; Qin, X.L. Efficient Antibacterial Silver Nanoparticles Composite Using Lignin as a Template. J. Compos. Mater 2015, 49, 2329–2335.
- Hu, S.X.; Hsieh, Y. Silver Nanoparticle Synthesis Using Lignin as Reducing and Capping Agents: A Kinetic and Mechanistic Study. Int. J. Biol. Macromol 2016, 82, 856–862.
- Zhang, Q.T.; Chen, C.Z.; Wan, G.C.; Lei, M.; Chi, M.C.; Wang, S.F.; Min, D.Y. Solar Light Induced Synthesis of Silver Nanoparticles by Using Lignin as a Reductant, and Their Application to Ultrasensitive Spectrophotometric Determination of Mercury(II). Microchim. Acta 2019, 186, 727.
- Shchukarev, A.V.; Korolkov, D.V. XPS Study of Group IA Carbonates. Cent. Eur. J. Chem 2004, 2, 347–362.
- Nohira, H.; Tsai, W.; Besling, W.; Young, E.; Petry, J.; Conard, T.; Vandervorst, W.; De Gendt, S.; Heyns, M.; Maes, J.; Tuominen, M. Characterization of ALCVD-Al2O3 and ZrO2 Layer Using X-ray Photoelectron Spectroscopy. J. Non-Cryst. Solids 2002, 303, 83–87.
- Ferraria, A.M.; Carapeto, A.P.; Botelho do Rego, A.M. X-ray Photoelectron Spectroscopy: Silver Salts Revisited. Vacuum 2012, 86, 1988–1991.
- Haglan, A.M.; Abbas, H.S.; Akköz, C.; Karakurt, S.; Güne, E. Characterization and Antibacterial Efficiency of Silver Nanoparticles Biosynthesized by Using Green Algae Enteromorpha intestinalis. Int. Nano Lett 2020, 10, 197–205.
- Shivakumar, M.; Nagashree, K.; Yallappa, S.; Manjappa, S.; Manjunath, K.; Dharmaprakash, M. Biosynthesis of Silver Nanoparticles Using pre-Hydrolysis Liquor of Eucalyptus Wood and its Effective Antimicrobial Activity. Enzyme. Microb. Tech 2017, 97, 55–62.
- Ashmore, D.A.; Chaudhari, A.; Barlow, B.; Barlow, B.; Harper, T.; Vig, K.; Miller, M.; Singh, S.; Nelson, E.; Pillai, S. Evaluation of E. Coli Inhibition by Plain and Polymer-Coated Silver Nanoparticles. Rev. Inst. Med. Trop. Sao. Paulo 2018, 60, e18.
- Nix, C.E.; Harper, B.J.; Conner, C.G.; Richter, A.P.; Velev, O.D.; Harper, S.L. Toxicological Assessment of a Lignin Core Nanoparticle Doped with Silver as an Alternative to Conventional Silver Core Nanoparticles. Antibiotics 2018, 7, 40.