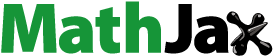
Abstract
Finding an alternative energy source that satisfies our energy needs while being ecologically beneficial is vital. This study aimed to synthesize biodiesel of ASTM standard from the non-edible seed oil of Capsella bursa-pastoris (L.) Medik over cheap MgO nanoparticles using various characterization techniques like XRD, SEM, FT-IR, GC-MS, and NMR. The optimum 92% biodiesel yield was achieved at a 1:15 oil-to-methanol ratio using 30 mg catalyst concentration at a temperature of 60 °C, and the 40 min reaction time. The low acid value (0.39 KOH mg/kg) and in-range kinematic viscosity (4.16 cSt at 40 °C) of biodiesel is close to the conventional diesel, whereas the low calorific value (27933 KJ/Kg) indicates the presence of more oxygen in biodiesel than conventional one. Very low sulfur % wt (0.0126) ensures the eco-friendly nature of synthesized biodiesel. 42.72 MJ/kg Higher heating value (HHV), 47 cetane number, 2.89 h oxidative stability, 0.041 mg/Kg water content, 349 °C Distillation temperature (for 90% recovery) and 0.037 carbon residue and presence of mostly unsaturated methyl esters (FAMEs) make the synthesized biodiesel engine friendly, good fuel efficacy and competitive source for the commercial production.
Introduction
Petroleum-based fuels continue to dominate the global transportation industry. The petroleum reservoirs are predicted to gradually run out and vanish over time [Citation1]. Finding an alternative energy source that satisfies our energy needs while being ecologically beneficial is vital. An option might be biodiesel made from agricultural waste [Citation2].
Initially, when petroleum supplies were cut off during World War 2, several nations utilized pure vegetable oil in their diesel engines. However, as the war ended and petro-fuels became available again, vegetable oil fuel was ignored [Citation2,Citation3]. Vegetable oil is transformed into fatty acids methyl esters by transesterification process and it is used to synthesize modern biodiesel fuel. Oils from biomass, vegetable oil, or animal fats may be used as feedstocks to synthesize biodiesel [Citation2]. Biodiesel is considered ecologically beneficial and biodegradable since it is produced by processing oils from biomass [Citation4].
Conventional diesel engines were tested with pure biodiesel and biodiesel combined with fossil fuel in various fractions, and it has been shown that no modifications are needed to the engine [Citation5]. Since vegetable oil has a low calorific value and a greater viscosity, trans-esterification decreases the viscosity and molecular weight by about one-third, improving the oil’s suitability as an engine fuel [Citation6]. As per the performance, the selection of oil, alcohol, and catalyst has a great impact [Citation5]. Catalytic transesterification of triglyceride and alcohol results in the formation of methyl ester and glycerol. It is the process used to convert biomass-derived oil into biodiesel [Citation2]. Triglycerides are made up of three long-chain fatty acids bonded to a basic glycerin molecule. As a result, the usual properties of oil vary depending on the type of fatty acid attached to the molecule. The characteristics of the feedstock can be determined from the oil composition, which in turn affects the quality of the biodiesel. Initially, homogeneous catalysts, such as potassium hydroxide, sodium hydroxide, or sodium methoxide were used to synthesize biodiesel [Citation7].
The process to be followed for converting oil into biodiesel must consider the oil’s composition and qualities [Citation8]. Before trans-esterification, oil with a greater concentration of free fatty acids has to be pre-treated. Esterification is used to pre-treat oil with high fatty acid content. Most often, sulfuric acid is used to facilitate esterification. Although, the high cost of biodiesel limits its commercialization. The key component is its feedstock, which typically represents between 70 and 80% of biodiesel costs. The best answer to this problem is non-edible feedstocks. Non-edible plants, such as Jatropha curcas, Pongamia pinnata, Madhuca indica, Silybum marianum (family Asteraceae), and Ricinus communis have 21–73% oil content [Citation9,Citation10]. For biodiesel synthesis, various non-edible feedstocks have been reported, such as date seed oil, rubber seed oil, palm kernel shell oil, and jatropha seed oil [Citation2].
The focus of research in biodiesel synthesis is on using catalysts to increase productivity, reduce energy consumption, and mitigate environmental impact. One of the key innovations to reduce the price of biodiesel is the change of catalytic systems from homogeneous to heterogeneous phases for biodiesel synthesis through trans-esterification. A heterogeneous catalyst is useful for biodiesel synthesis since it can be reused and works well in terms of cost and product quality. The trans-esterification reaction is catalysed by an acid catalyst as well as a basic catalyst. Metal oxide nanoparticles like cadmium oxide, cadmium sulfide, tungsten oxide, and copper oxide have dual functionalities and achieved excellent biodiesel yield over various non-edible oils at different oil-to-methanol volume ratios (O/M) [Citation11–14]. Over nonedible Diospyros malabarica oil (O/M = 1/9), Cadmium oxide nanoparticles catalyst (0.5 wt%) showed as high as 94% biodiesel yield in 12th run at reaction duration 180 min at 90 °C [Citation11]. Cadmium sulfide nanoparticle (0.5 wt%) showed the same biodiesel yield over Ailanthus altissima seed oil (O/M = 1/3) for 90 min at 60 °C [Citation12]. In the same way, the WO3 nanoparticle catalyst (2 wt%) acted over Cannabis sativa seed oil (O/M = 1/7) and showed the highest 91% biodiesel yield during 120 min at 80 °C [Citation13]. Copper oxide nanoparticle (0.18 wt%) catalyzed nonedible Citrus medica seed oil (O/M = 1/8) and attained a maximum 93% biodiesel yield of 85 °C for 120 min [Citation14].
The transition metal and inner transition metals like Zr, Cu, Ce, and La modified montmorillonite clay catalyst (3.5 wt%) were found quite productive in biodiesel yield from non-edible seed oil [Citation15–17]. Copper-modified montmorillonite clay catalyst showed 83% biodiesel yield from Raphnus raphanistrum L. seed oil (O/M = 1/15) at 150 °C for 5 h. In contrast, zirconia-modified montmorillonite clay catalyst achieved a maximum 88% biodiesel yield from Lepidium perfoliatum Linn. Seed oil (O/M = 1/15) at 135 °C for 4h [Citation15,Citation16]. Munir et al. utilized Ce, Cu, La loaded montmorillonite clay catalyst for the conversion of nonedible Celastrus paniculatus seed oil (O/M = 1/12) with 89.42% biodiesel yield at 120°C for 3 h [Citation17]. Nonedible Prunus cerasoides D. Don had high seed oil content (54.5) and Cd-Mn impregnated montmorillonite clay catalyst (4 wt%) transformed 1:12 oil to methanol ratio of this feed stock into 85% biodiesel yield at 120 °C for 5h [Citation18].
Among acid catalysts, chlorinated SnO2 solid acid catalyst, the compartmentalized SnO2@γ-Al2O3 structures, magnetic Fe3O4 solid acid catalyst, and feldspar supported iron doped tin oxide nanomagnetic catalyst had drawn interest due to high biodiesel yield and reusability [Citation19–22]. However, high-rate equipment corrosion is a major demerit of acid-based catalysis. ZnO is amphoteric, and alkaline metal oxides are basic in nature. 15 mg ZnO catalyst was much more effective in converting Silybum marianum oil (O/M = 1/24) with 91% yield at 60 °C during 45 min of reaction time [Citation10]. The basicity and low cost of CaO had drawn interest. 20 mg CaO catalyst converted R. communis oil (O/M = 1/15) with 89% biodiesel yield at 60 °C for 100 min reaction time [Citation9]. However, in the presence of water, the catalytic activity of CaO was affected [Citation23]. Five to 20 wt% ZrO2 dispersed over CaO had higher basicity and stability than bare CaO [Citation24]. 5 wt% catalyst carried out >90% biodiesel yield over J. curcas oil (O/M = 1/15) at 75 °C during 3 h. CaO-ZrO2 mixed oxide (atomic ratio 0.5) over waste cooking oil and CaO-La2O3 mixed oxide over J. curcas feedstock had tried [Citation25,Citation26], but catalytic activity droped on successive cycles due to leaching of active sites. Ala et al. showed that CeO2 supported 15 wt% CaO catalyst had more than 20 times higher dual acid-base site than bare CeO2 nanoparticles, which caused optimum 90.14 wt% biodiesel yield from Loquat seed oil (O/M = 1/9) at 70 °C during 90 min of time [Citation2]. SrO has a higher basicity than CaO but it acted homogeneously [Citation27]. La2O3 modified with SrO (with atomic ratio 8) was perfectly heterogeneous [Citation28]. It had a strong interaction between metal oxides and adequate pore channels with additional basic sites at 80–220 °C (than La2O3) [Citation28]. 3 wt% catalyst loading brought 97.28% biodiesel yield from Prunus armeniaca seed oil (O/M = 1/9) at 65 °C during 75 min of residence time. The stable methyl ester production was continued up to six cycles. BaO has the highest basicity among alkaline metal oxide and alkali metal-promoted BaO was found quite efficient in transesterification (>85% methyl ester yield), but high toxicity and high methanol solubility limit its application for biodiesel production [Citation29]. Although, a dense hydrophilic polymeric membrane applied to BaO nanoparticle (0.039 wt% amount) was found quite effective and reusable up to five cycles with high biodiesel yield (>70%) over Toona ciliata seed oil (O/M = 1/9) at 90 °C for 2.5 h [Citation30]. MgO had basicity and water tolerance capacity (not over CaO) [Citation31]. The mesoporous MgO (prepared by the templating method) had a higher surface area and 2.5 times highly dense basic sites than conventional MgO catalyst. 3 wt% catalyst loading carried out average 95% methyl ester yield up to five runs from canola’s oil (O/M = 3/20) at 190 °C during 3h reaction time. Saman et al. prepared size-controlled porous MgO nanoparticles (22–25 nm) using (polyvinyl pyrrolidone), MgNO3 precursor, Ziziphus leave extract, and NaOH. 0.015 g of MgO nanoparticles carried out about 98% biodiesel yield over Ziziphus mauiritiana oil (O/M = 1/8) at 65 °C during 4 h reaction. Up to the 6th cycle, the conversion remained at 84% methyl ester without the formation of any saponification product [Citation32]. The introduction of 20 wt% Cs2O in mesoporous carbon-supported MgO catalyst (prepared by the combustion process in the presence of pomegranate seed extract) caused a rise in average particle size and attainment of optimum basicity (especially strong basic sites) [Citation33]. 4 wt% catalyst acted over olive oil (O/M = 1/15) and achieved 96% biodiesel yield at 65 °C in a 4 h reaction. Up to 4 cycles, >93% biodiesel yield was noticed without loss of active component. Cobalt tungstate dispersed reduced graphene oxide catalyst (0.8 wt%) achieved 99.7% biodiesel yield over Carthamus lanatus L. seed oil (O/M = 1/12) at 65°C for 2 h reaction [Citation34] with reusability up to 7 times.
Rigorous research is underway to utilize non-edible seed oil (from widely available wild herbs) in high-yield biodiesel formation through cheap catalytic routes. It is simply the utilization of waste herbs in the country’s oil economy. Herein, we have utilized wild Capsella bursa-pastoris (L.) Medik seed oil was used in biodiesel production through cheap alkaline metal oxide catalysts. Capsella bursa-pastoris (L.) Medik (family Brassicaceae) is a widely available annual herb worldwide. It has a high oil content of 6–12 seeds in each locule (The details are shown in Supporting Information SCitation1 and Figure S2). It is a spring biennial plant among the most widespread plants in the world [Citation35]. The plant is cosmopolitan in distribution but commonly present in the world’s subtropical and temperate subtropical regions. The plant may survive in various climates, from sea level to the high Himalayas and at high and low temperatures [Citation36]. Being the second most prevalent dicotyledonous weed in the world, the plant is commonly grown in vegetable, rape, and wheat fields. The cosmopolitan distribution and common occurrence of the plant are because of the production of a large number of seeds that have the ability to survive for decades in the soil seed bank. The quantity of seeds produced by a plant varies based on its habitat as well as its genotype [Citation35]. The plant grows in various soils but grows well in clay to sandy-loam soil and is also well-adapted to drought conditions. It grows in many harsh and disturbed areas, including roadsides, gravel parking lots, waste fields, demolition plots, manure piles, and compost heaps [Citation37]. Various bifunctional/acidic/basic catalysts were utilized to convert non-edible seed oil into biodiesel potentially. This study is aimed to synthesize biodiesel from the seed oil of C. bursa-pastoris (L.) Medik by using cheap MgO nanoparticles as a catalyst. Different parameters, i.e. oil-to-methanol ratio, catalyst amount, reaction time, and reaction temperature, were investigated to find the suitable condition for the maximum biodiesel yield. Further, the qualitative and quantitative analysis of different fatty acid methyl esters (FAMEs) in biodiesel are carried out. The physical and fuel property of produced biodiesel is compared with ASTM standards.
Materials and methods
MgO nanoparticles synthesis
Magnesium nitrate (MgNO3.6H2O; Merck, purity 99%) and sodium hydroxide (NaOH, Merck, purity 98%) were used as the precursor material for the synthesis of magnesium oxide nanoparticles. 0.5 M sodium hydroxide solution was added drop-wise to 100 ml of 0.2 M magnesium nitrate (MgNO3.6H2O) solution under constant stirring. A white precipitate of magnesium hydroxide was visible in the beaker after some time. Using an expandable ion analyzer (EA 940, Orian, Korea), the pH of the solution was determined (12.5). To eliminate ionic contaminants, the precipitate was filtered and washed several times with methanol. After that, it was centrifuged for 5 min at a speed of 5000 revolutions per minute and dried at room temperature. At 500 °C, samples of dry white powder were annealed for 2 h [Citation38].
X-ray diffraction (XRD)
PI TLXRD is the most used method for identifying the fundamental crystallographic characteristics of nano-catalysts [Citation39]. By keeping an applied voltage and current at 40 KV and 30 mA at 2θ range of 10–90°, the XRD was carried out by using a SHIMADZU 6000 diffractometer outfitted with a Cukα (K = 1.54 A°) source. The crystallite sizes were calculated using the step size and scan rate at a speed of 10 °C per minute. Between 10 and 60 °C, all dimensions were obtained. XRDA31 software was used to calculate peak locations and peak broadening. The Scherer equation (Equation 5) was used to finish the computation and obtain a heterogeneous average diameter of nanoparticles.
(1)
(1)
‘k’ is shape factor = 0.9, ‘λ’ is radiation wavelength (1.54 Å), and ‘β’ is full width of half of the maximum (FWHM) intensity in radians.
Scanning electron-microscopic technique (SEM)
Scanning electron microscope Model JEOL JSM-5910 & HT7800 Ruli was used to perform scanning electron microscopy of the synthesized nanoparticles. SEM microscope field emissions were operated with a 20 kV accelerating voltage to produce the scanned pictures. It enabled the qualitative assessment of the surface of catalysts and contributed to explaining the phenomena that happened during calcining and pre-treatment. Malvern Zetasizer Nano ZS96 Analyzer was used to find the particle size using the DLS technique. The samples were dispersed in double-deionized water. At 25 °C, the analysis was carried out in a disposable-sized cuvette. The device had a count rate of 291.4 kcps for 60 s.
Oil extraction from feedstock
To remove the dust, seeds were washed in distilled water and then oven-dried at 50 °C. After that, around 10 g of dried seeds were finally ground up using a mortar and pestle. Petroleum ether was used as a solvent in the Soxhlet extraction treatment of the powdered seed to determine the oil contents of the feedstock. A 250 mL round-bottom flask of Soxhlet apparatus was filled with ether. A thimble holding crushed powder of seeds was positioned centrally in the apparatus and heated up to 60 °C for 5–6 h. Ether was recycled at 55 °C in the rotary evaporator. The total oil content was determined by using the formula [Citation9].
(2)
(2)
W1 represents the weight of the empty flask, W2 is the weight of the powdered seed before oil extraction, W3 is the weight of the flask after the oil extraction, and W4 is the weight of the feedstock’s oil content. Mechanical extraction was used to get large amounts of oil. It was carried out using an electrical expeller model, YZS-130A/C. The crude oil was filtered with Whatman filter paper–42. Then, the filtered oil was separated in a glass jar [Citation40].
Determining the feedstock’s free fatty acid (FFA) composition
Through aqueous acid-base titration, the free fatty acid (FFA) concentration was determined. One milliliter of feedstock oil and 9 ml of isopropyl alcohol were combined in a beaker, and two drops of phenolphthalein as an indicator were then added to the mixture. This was allowed to titrate until the pink color (endpoint) showed against 0.025 M of KOH solution. To determine the average KOH solution used for the sample titration, the sample titration was performed three times. The following equation was used to determine crude oil’s FFA value [Citation10]
(3)
(3)
A = amount of KOH used, B = Amount of KOH used during blank titration, C = Concentration of KOH (g/l), V = Volume of oil sample.
Biodiesel synthesis process
Due to the low FFA (0.39 mg KOH/g) content of the feedstock, we adopted the transesterification method to synthesize biodiesel. The percentage yield of biodiesel was calculated using the following formula [Citation41].
(4)
(4)
Assessment of physical and chemical properties of biodiesel
Different fuel properties of the synthesized biodiesel were determined through the ASTM standard technique shown in [Citation42]. Fourier transform infrared (FT-IR) spectroscopy, Gas Chromatography-Mass Spectrometry (GC-MS), and Nuclear magnetic resonance (NMR) spectroscopic analyses have been performed to confirm the synthesis and study of the chemical properties of C. bursa-pastoris biodiesel (CBPB).
Table 2. Fuel properties of the CBPB and ASTM standard methods adopted for property checking.
NMR spectroscopy of biodiesel sample
The Avance NEO Bunker 600 MHz spectrometer was used to perform the 1H and 13C NMR spectrometry at 21 °C on 11.75 T. A 5 mm BBF smart probe was equipped in the spectrometer. As internal standard solvents for authentication, chloroform-d and Si(CH3)4 were utilized. When measuring the spectrum for 1H NMR (300 MHz), 1.0 scans, 8 scans of recycle time, and a pulse duration of 30° were used. Accordingly, the spectrum of the 13C NMR (75 MHz) was conducted at 1.89 with a 160 scan recycle delay and 300 pulse duration. In relation to the residual solvent peak, the conversion of triglycerides to associated fatty acid methyl esters (FAMEs) was measured in ppm. The following formula was used to calculate the conversion yield [Citation10].
(5)
(5)
C = Oil to biodiesel conversion percentage, Ame = methoxy protons’ integration value in biodiesel, ACH2 = α-methylene protons’ integration value in biodiesel
Fourier transform infrared (FT-IR) analysis of CBPB
The existing functional groups were identified using FT-IR analysis based on the presence of bands in the samples. The various stretching and bending vibrations seen in samples of biodiesel are reflected in the FT-IR spectrum [Citation43]. The VARIAN Model-AA280Z FT-IR spectrometer was used to obtain the infrared spectra for the synthesized biodiesel. A graphite tube atomizer model GTA-120 was installed in the spectrometer. Each spectrum was obtained in a brief period of time using 4 scans at a resolution of 4 cm1. In the range of 400–4000 cm−1, the spectrometry was performed. A computer software application spectrum was used to process all the results.
Qualitative and quantitative evaluation of the biodiesel
The Shimadzu Japan-made Model QP-2010 Plus GC-MS spectrometer was used to identify various FAMEs in the biodiesel sample. Biodiesel in the amount of 0.1 µL was injected into the spectrometer. C6H14 was used as a solvent, while helium was used as a vector gas at a specific rate of ‘1.44 mL/min.’ The injector and detector temperature was set at 250 °C and the column temperature was adjusted to a range of 50–300 °C with an 80° per minute flow rate. The mass spectrograph was set to scan with electron impact ionization between 50 and 1000 m/z values.
Results and discussion
X-ray diffraction (XRD)
shows X-ray diffraction pattern of cuboidal MgO nanoparticles. The diffraction peaks at 2θ angles of 37.52, 42.78, 61.87, 74.49, and 78.62° that are corresponding to the 111, 200, 220, 311, and 222 Miller indices, respectively (JCPDS No. 87-0653) [Citation39,Citation44]. The mean size (25 nm) of the nanoparticles was calculated from the peaks at 200 and 220 planes with the help of Scherrer’s equation [Citation10].
Surface and morphological characteristics study of nanoparticles
We studied the surface and morphological characterizations of the nanoparticles using the scanning electron microscope Jeol Model JSM-6390LV. It is clear from that the particles have a smooth surface and are cubic in shape [Citation45]. The production of a large number of irregularly pitted microcrystallites was predicted based on CO2 ejection from carbonate-rich regions [Citation46]. The nanoparticles’ average size was 23 nm [Citation32]. The DLS method was used to determine the particle size using the Malvern Zetasizer Nano ZS96 Analyzer. The samples were dispersed in double-deionized water. At 25 °C, the analysis was carried out in a disposable-sized cuvette. The device had a count rate of 291.4 kcps for 60 s.
Raw oil extraction and assessment of FFA contents
The European Union has recommended the chemical extraction of raw oil through a Soxhlet apparatus [Citation9]. The oil extraction using Soxhlet verifies that the feedstock contains 29.6% oil content. After the chemical extraction, a large quantity of oil was extracted using mechanical extraction. The FFA content was calculated using the acid-base titration technique. The FFA value of the current feedstock was 0.39 mg KOH/g, which is less than the limit specified by ASTM D-6751 [Citation40].
The effect of various parameters on biodiesel yield during trans-esterification
The trans-esterification technique was adopted to synthesize biodiesel. To get optimum biodiesel yield, we varied the parameters, such as the oil-to-methanol ratio, catalyst concentration, reaction duration, and reaction temperature [Citation47].
Oil to methanol ratio
In this study, for optimum yield of biodiesel, the oil-to-methanol ratios were kept at 1:5, 1:10, 1:15, 1:20, and 1:25. shows that the oil-to-methanol ratio has a positive effect on biodiesel yield and negative effect on glycerin formation. The maximum yield of biodiesel was achieved at a 1:15 methanol-to-oil ratio. No significant increase in yield was observed in high ratios (1:20 and 1:25), but as methanol concentration increases, biodiesel yield increases [Citation40,Citation47]. Since the transesterification reaction is reversible, a higher molar ratio increases the miscibility and interaction between the triglyceride and alcohol molecules [Citation48].
The minimum stoichiometric molar ratio of oil to methanol for a successful transesterification reaction is 1:3. As transesterification is a reversible reaction, a substantial amount of methanol is required to break the bonds between fatty acids and glycerin [Citation49]. However, if the concentration of methanol is too high, it cannot optimize biodiesel yield, hinders the ester recovery process, and raises product costs [Citation40]. The greater ratios of oil to methanol than 1:70 slow the separation of esters from glycerol [Citation50].
Catalyst concentration
In this study, different catalyst concentrations, 10, 20, 30, 40, and 50 mg, were studied to find the suitable catalyst concentration for optimum yield because the catalyst concentration directly affects the biodiesel yield. The transesterification reaction occurs successfully when a desirable amount of catalyst is available [Citation39,Citation43]. This work demonstrates that the maximum biodiesel yield and minimum glycerin yield were achieved at 30 mg catalyst concentration (). With the increase in the catalyst concentration, biodiesel yield increases, and the reaction time decreases because more active sites are available for reactants to convert them into products [Citation51]. A suitable concentration of catalysts also reduces the cost of the product by reducing the energy consumption. Increasing the amount of catalyst increases the yield of soap due to emulsification. Increasing the amount of catalyst to more than 30 g also increases the viscosity of the reaction solution, thereby decreasing the yield of biodiesel [Citation9,Citation52].
Reaction temperature
To determine the optimum temperature for biodiesel yield, the transesterification reactions were conducted at five different temperatures (50, 55, 60, 65, and 70 °C) while keeping the other parameters constant. The temperature was kept variable because it has a direct effect on the biodiesel yield and reaction time [Citation10,Citation28]. Results reveal that when increasing the temperature from 55 to 60 °C, biodiesel yield increased to 92%, and glycerin yield decreased to 5%. Furthermore, a slight decrease was also observed in the yield at 70 °C (). There is a significant relationship between the reaction temperature and the biodiesel yield and reaction time because a high temperature reduces the viscosity of the solution, increases the solubility of the reactants, and accelerates the transfer rate of reactants to the product [Citation45,Citation46]. The decrease in biodiesel yield above 65 °C may be due to an increase in miscibility, which reduces phase separation and yield [Citation10]. Above 65 °C, the molar ratio of methanol to oil decreases due to the evaporation of methanol from the reaction mixture, thereby decreasing the biodiesel yield [Citation9,Citation53]. Similar findings have been reported in other studies [Citation40,Citation54,Citation55].
Reaction time
Similar to the other parameters discussed previously, the time of reaction plays a significant role in the yield of biodiesel, particularly on an industrial scale, because prolonged reaction time increases the cost of production due to increased energy expenditure. So, it should be minimized [Citation53,Citation54,Citation56,Citation57]. To determine the minimum ideal reaction time for maximum biodiesel yield, different reaction times of 15, 30, 45, 60, and 75 min were studied over 30 mg catalyst and 60 °C reaction temperature. Maximum 92% biodiesel yield was achieved at 40 min of reaction time (). A decline in biodiesel yield was observed at a longer reaction time. According to the literature, hydrolysis of esters occurs at a slower rate, resulting in the production of more soap [Citation55]. Other researchers reported the same results [Citation40,Citation54,Citation55,Citation57].
Overall, 30 mg of catalyst attained a biodiesel yield as high as 92% over C. bursa-pastoris (L.) Medik seed oil (in 1:15 oil to methanol ratio) in 40-min reaction at 60 °C. The comparative biodiesel yield of various non-edible plants over different catalysts is shown in . It can be said that the current catalyst has taken a minimum duration (40 min) and the lowest temperature (60 C) to reach the highest biodiesel yield (92%) than the rest of the catalysts. Furthermore, the wide distribution of this annual herb (C. bursa-pastoris (L.) Medik) on arid land has the potential to strengthen the oil economy through the production of biodiesel.
Table 1. The comparative biodiesel yield of various non-edible herbs over different catalyst.
Physical and fuel properties of biodiesel
The ASTM D-664 specified the acid value (0.80 mg KOH mg/kg) for biodiesel [Citation58], while the CBPB has an acid value of 0.39 mg KOH mg/kg. The purification quality of biodiesel affects its acid value [Citation58]. Likewise, the kinematic viscosity of CBPB is 4.16 mm2/s at 40 °C. This value is also close to conventional diesel [Citation59]. Furthermore, the density value of CBPB is 0.835 kg/L, and it also falls in the range specified by ASTM D-4052 [Citation60]. The calorific value of the synthesized biodiesel is 27933 kJ/kg, which is also within the range of ASTM D-5865. As biodiesel contains more oxygen than conventional diesel, it has a lower calorific value [Citation40,Citation61] ().
The flash point of CBPB is 109 °C, which is within the limit of ASTM D-93 (130 °C). It depends on the contents of methanol in the biodiesel. The flash point of biodiesel is reduced to 50% by increasing the methanol contents by 0.5% ().
The ASTM D-613 has defined a cetane number of 45 for biodiesel, while the CBPB has a cetane number of 47 (). The cetane number can also be reduced by adding a small amount of nitric acid iso-octyl [Citation62,Citation63]. The value of the Clod Point (CP) is −3.7 °C, and the Pour Point (PP) is −8.1 °C. These values fall within the range of ASTM standards [Citation64]. The sulfur content of the synthesized biodiesel is 0.0126 ppm. It is less than the specified value of ASTM D-5453. Therefore, the CBPB is environmentally friendly biodiesel [Citation40,Citation49].
The oxidative stability of CBPB is 2.89 h, which is very close to the minimum limit of ASTM EN-14112. Biodiesel tends to react with O2 at room temperature. It reveals the relative susceptibility of fuel to oxidative degradation [Citation65]. However, biodiesel’s oxidation susceptibility is desirable from the environmental point of view [Citation66]. It is undeniably a major flaw and a barrier to commercialization. During storage or use, oxidation modifies the physicochemical and tri-biological characteristics of biodiesel. This phenomenon has a direct impact on the properties of biodiesel, namely its acid number, density, iodine value, kinematic viscosity, polymer content, and peroxide value. Although, the susceptibility of biodiesel to oxidation can be reduced by adding various antioxidants [Citation67,Citation68].
We have determined that CBPB has 0.041 water content, which is below the ASTM D-6304 limit. Water content is a crucial aspect of the quality of fuel. Due to FAMEs, biodiesel is more hygroscopic and hydrophilic than conventional fuels. Therefore, its capacity to absorb moisture is greater. High moisture contents in biodiesel promote biological growth in the fuel tanks; this leads to the corrosion of fuel tanks as well as the assimilation of slime and sludge, thereby clogging the engine filters and fuel pipes; this causes the destruction of the fuel injection system of the vehicle [Citation69].
For CBPB, the iodine value was calculated at 127, which is slightly higher than the limit of ASTM D-4607. The iodine value reveals biodiesel’s oxidative stability, saturation, and unsaturation degree [Citation70]. Similarly, the refractive index of CBPB is 1463. The refractive index depends on the molecular weight of the oil, degree of saturation, size of its chain, and degree of conjugation. It confirms the successful conversion of crude oil to biodiesel [Citation71]. We also checked the synthesized biodiesel’s carbon residue, which is below the limit specified by ASTM D-4530 ().
According to the results of this study, the Higher Heating Value (HHV) for CBPB is 42.72, within the range specified by ASTM D-240. HHV, like other fuel properties, is also an important characteristic of biodiesel because it provides information about energy contents and fuel efficiency [Citation72]. It is calculated from the biodiesel’s fatty acid composition, iodine content, and saponification value [Citation73]. Fossil fuels have a slightly higher HHV (49.65 MJ/kg) than biodiesel [Citation74]. The distillation temperature for recovery of 90% biodiesel is 349 °C, also found below the limit of ASTM D-1160-06.
1H-NMR spectroscopy of CBPB
At 3.528 ppm, the singlet peak for methoxy proton (−OCH3) was attained. It confirms the higher conversion of raw oil to FAMEs [Citation75]. At 2.003–2.047, the triplet peak for alpha-methylene proton (α-CH2) was observed. These two peaks confirmed the formation of FAMEs from triglycerides. At 0.839–0.900 ppm, the peaks for terminal methyl protons (CH3) were attained. For beta-carbonyl methylene protons, the peaks were achieved at 1.275–1.638 ppm. The indication peaks for the olefinic hydrogen were achieved at 5.211–5.409 ppm () [Citation76]. These are the confirmative peaks for the effective conversion of triglycerides to biodiesel [Citation40,Citation77].
13C-NMR study of CBPB
At 24.88–34.21 ppm, long-chain ethylene carbons (–CH2–) peaks were achieved. The singlet peak obtained at 51.34 ppm confirms the presence of (–O–CH3) [Citation24]. For ester carbonyl carbon (–CO), the peaks were attained at 174.19 ppm; for olefinic carbons, the peaks were attained at 129.59–130.09 ppm. The peaks at 127.77 and 128.13 ppm indicate the presence of a vinylic (C−H) substituent (). Other studies have also reported the same function groups’ peaks in this range [Citation78].
Fourier transform infrared analysis of CBPB
The FT-IR spectroscopy confirms the presence of different functional groups and the corresponding bond linkages as well as vibrations of stretching and bending. It is a powerful analytical tool for recognizing the macromolecular pools (e.g. carbohydrates, lipids, and proteins) and monitoring biochemical changes [Citation79]. FAMEs are observed to absorb electromagnetic radiation of wavelength in the infrared region [Citation80]. According to Soon [Citation81], the position of the carbonyl group depends on molecular structure as well as substituent effects. The stretching absorption band at 2934 cm−1 for the methylene group and 2869 cm−1 for the methyl group was found. The methoxycarbonyl (methyl ester) group was observed at 1751 cm−1. Similarly, the absorption band for methylene bend was obtained at 1481 cm−1. An absorption band attained at 1390 cm−1 confirms the presence of trimethyl. The absorption band obtained at 1339 cm−1 shows the methyne C–H bend. The absorption band for aromatic ether (aryl–O stretching) was obtained at 1227 cm−1. The absorption band obtained at 1113 cm−1 shows alkyl-substituted ether and C–O stretching. The absorption band of the cyclic ethers stretching was obtained at 1062 cm−1. The absorption band obtained at 975 cm−1 is for methyne of skeletal C–C vibration. The methylene –(CH2)n– rocking peak was obtained at 765 cm−1. The last absorption band obtained at 681 cm−1 is for the alkyne C–H bend () [Citation82]. An FTIR spectroscopic study was performed to confirm the various functional groups formed during the transesterification process. There are three main IR bands for ester formation were observed; one is the carbonyl group at 1725–1750 cm−1, the second is C–O at 1000–1300 cm−1 [Citation40], and the third one at 1435 cm−1 for –O–CH3 found [Citation83,Citation84]. The absorption bands obtained in FT-IR spectroscopy confirm that the transesterification process occurred successfully, and it is also a suitable method for converting triglyceride to fatty acid methyl esters [Citation85,Citation86].
Qualitative and quantitative analysis of CBPB
For the qualitative and quantitative study of the FAMEs produced after the successful occurrence of transesterification, gas chromatography and mass spectroscopy were conducted. It is evident from the result of GC-MS that the CBPB has FAMEs of 17 different types. The peak of each single fatty acid methyl ester was confirmed with the help of NIST 02 library match software. Each fatty acid methyl ester was identified from its retention time (). It is clear from the result that the major FAMEs are Linolenic acid methyl ester, Linoleic acid methyl ester, Oleic Acid methyl ester, Elaidic acid methyl ester, and 11-Eicosadienoic acid methyl ester (Figure S3). The GC-MS result shows that most of the FAMEs are unsaturated quantitatively. These FAMEs indicate that biodiesel has improved fuel properties, thus recommending high fuel efficiency [Citation87].
Table 3. A primary table of reported FAMEs, retention time, and percentage.
Conclusion
Biodiesel is one of the current renewable and green energy sources capable of meeting the future energy demand. In the present study, widely available non-edible Capsella bursa-pastoris (L.) Medik. Seed oil was used to produce biodiesel through trans-esterification over MgO nanoparticles. In reference to process parameters, it is found that biodiesel yield was highly sensitive to catalyst loading followed by methanol: oil ratio and reaction time. Maximum biodiesel yield of 92% was obtained at a ratio of 1:15 oil to methanol, 30 mg catalyst concentration, 60 °C, and 40 min of reaction time. Compared with the literature report on various non-edible oils over different catalysts, the time duration (40 min) and temperature (60 °C) are minimal for getting >90% biodiesel conversion. Monitoring and controlling the amount and quality of produced biodiesel and its potential for dependable commercialization was the primary goal of the fuel characteristics test and the application of different analytical techniques. The fuel properties are found within the limit of ASTM standard limits. Further, quantitative analysis confirms that most fatty acid methyl esters (FAMEs) are unsaturated. The acid value and kinematic viscosity values of synthesized biodiesel are close to the conventional diesel, whereas it has a higher oxygen content (low calorific value) than conventional diesel. Low water content and very low sulfur content make it engine-friendly as well as eco-friendly. HHV value, centane number, oxidative stability, and carbon residue value of biodiesel reflects its high energy content and fuel efficiency. The optimisation study can help determine the economic impact of advancing biodiesel production. Overall, the widely available C. bursa-pastoris seed oil, its potential conversion into biodiesel over MgO nanoparticles, and fuel quality close to ASTM standards make it a competitive source for commercial biodiesel production. Furthermore, the wide distribution of this annual herb on arid land has the potential to strengthen the oil economy through the production of biodiesel.
Authors contributions
The present work has been written with equal, direct, and intellectual contributions from all authors, who have also given their approval for publishing in this journal.
Supplemental Material
Download MS Word (736 KB)Acknowledgments
We all are expressing our heartfelt gratitude to the Researchers Supporting Project number (RSP2024R435) of King Saud University. JA acknowledges Queen’s University Belfast for supporting research. All data is provided in full in the results section of this paper.
Disclosure statement
No potential conflict of interest was reported by the author(s).
Data availability statement
All the data is presented in the manuscript.
Additional information
Funding
References
- Mirhashemi FS, Sadrnia H. On the maximal strength of a first-order electroweak phase transition and its gravitational wave signal. J Energy Inst. 2019;2019:1–23. doi: 10.1016/j.joei.2019.04.003.
- Al-Muhtaseb AH, Osman AI, Sahaya P, et al. Circular economy approach of enhanced bifunctional catalytic system of CaO/CeO2 for biodiesel production from waste loquat seed oil with life cycle assessment study. Energy Convers Manag. 2021;236:114040. doi: 10.1016/j.enconman.2021.114040.
- Knothe G, Razon LF. Biodiesel fuels. PECS. 2017;58:36–59. doi: 10.1016/j.pecs.2016.08.001.
- Campbell JE, Block E. Land-use and alternative bioenergy pathways for waste biomass. Environ Sci Technol. 2010;44(22):8665–8669. doi: 10.1021/es100681g.
- Chhabra P, Mosbach S, Karimi IA, et al. Practically useful models for kinetics of biodiesel production. ACS Sustain Chem Eng. 2019;7(5):4983–4992. doi: 10.1021/acssuschemeng.8b05636.
- Erdiwansyah R, Mamat MSM, Sani K, et al. An overview of higher alcohol and biodiesel as alternative fuels in engines. Energy Rep. 2019;5:467–479. doi: 10.1016/j.egyr.2019.04.009.
- de Lima AL, Ronconi CM, Mota CJ. Heterogeneous basic catalysts for biodiesel production. Catal Sci Technol. 2016;6(9):2877–2891. doi: 10.1039/C5CY01989C.
- Wong KY, Ng JH, Chong CT, et al. Biodiesel process intensification through catalytic enhancement and emerging reactor designs: a critical review. Renew Sust Energy Rev. 2019;116:109399. doi: 10.1016/j.rser.2019.109399.
- Ahmad Jan H, Šurina I, Zaman A, et al. Synthesis of biodiesel from Ricinus communis L. seed oil, a promising non-edible feedstock using calcium oxide nanoparticles as a catalyst. Energy. 2022;15(17):6425. doi: 10.3390/en15176425.
- Jan HA, Šurina I, Al-Fatesh AS, et al. Biodiesel synthesis from milk thistle (Silybum marianum (L.) Gaertn.) seed oil using ZnO nanoparticles as a catalyst. Energy. 2022;15(20):7818. doi: 10.3390/en15207818.
- Arshad S, Ahmad M, Munir M, et al. Assessing the potential of green CdO2 nano-catalyst for the synthesis of biodiesel using non-edible seed oil of Malabar ebony. Fuel. 2023;333:126492. doi: 10.1016/j.fuel.2022.126492.
- Jabeen M, Munir M, Abbas MM, et al. Sustainable production of biodiesel from novel and non-edible Ailanthus altissima (mill.) seed oil from green and recyclable potassium hydroxide activated ailanthus cake and cadmium sulfide catalyst. Sustainability. 2022;14(17):10962. doi: 10.3390/su141710962.
- Abbasi TU, Ahmad M, Asma M, et al. High efficient conversion of Cannabis sativa L. biomass into bioenergy by using green tungsten oxide nano-catalyst towards carbon neutrality. Fuel. 2023;336:126796. doi: 10.1016/j.fuel.2022.126796.
- Chia SR, Ahmad M, Sultana S, et al. Reen synthesis of biodiesel from citrus medica seed oil using green nanoparticles of copper oxide. Fuel. 2022;323:124285. doi: 10.1016/j.fuel.2022.124285.
- Munir M, Ahmad M, Rehan M, et al. Production of high quality biodiesel from novel non-edible Raphnus raphanistrum L. seed oil using copper modified montmorillonite clay catalyst. Environ Res. 2021;193:110398. doi: 10.1016/j.envres.2020.110398.
- Munir M, Saeed M, Ahmad M, et al. Optimization of novel Lepidium perfoliatum Linn. biodiesel using zirconium-modified montmorillonite clay catalyst. Energy Sources Part A Recov Util Environ Eff. 2022;44(3):6632–6647. doi: 10.1080/15567036.2019.1691289.
- Sukoco BM, Lestari YD, Susanto E, et al. Middle manager capabilities and organisational performance: the mediating effect of organisational capacity for change. Int J Product Perform Manag. 2022;71(4):1365–1384. doi: 10.1108/IJPPM-07-2019-0364.
- Munir M, Ahmad M, Saeed M, et al. Sustainable production of bioenergy from novel non-edible seed oil (Prunus cerasoides) using bimetallic impregnated montmorillonite clay catalyst. Renew Sustain Energy Rev. 2019;109:321–332. doi: 10.1016/j.rser.2019.04.029.
- Naghmash MA, El-Molla SA, Mahmoud HR. Synthesis and characterization of novel chlorinated SnO2 nanomaterials for biodiesel production via stearic acid esterification with methanol. Adv Powder Technol. 2022;33(4):103532. doi: 10.1016/j.apt.2022.103532.
- Prestigiacomo C, Biondo M, Galia A, et al. Interesterification of triglycerides with methyl acetate for biodiesel production using a cyclodextrin-derived SnO@ γ-Al2O3 composite as heterogeneous catalyst. Fuel. 2022;321:124026. doi: 10.1016/j.fuel.2022.124026.
- Al-Mawali KS, Osman AI, Al-Muhtaseb AH, et al. Life cycle assessment of biodiesel production utilising waste date seed oil and a novel magnetic catalyst: a circular bioeconomy approach. Renew Energy. 2021;170:832–846. doi: 10.1016/j.renene.2021.02.027.
- Hanif M, Bhatti IA, Zahid M, et al. Production of biodiesel from non-edible feedstocks using environment friendly nano-magnetic Fe/SnO catalyst. Sci Rep. 2022;12(1):16705. doi: 10.1038/s41598-022-20856-7.
- Kouzu M, Hidaka JS. Purification to remove leached CaO catalyst from biodiesel with the help of cation-exchange resin. Fuel. 2013;105:318–324. doi: 10.1016/j.fuel.2012.06.019.
- Kaur N, Ali A. Kinetics and reusability of Zr/CaO as heterogeneous catalyst for the ethanolysis and methanolysis of Jatropha curcas oil. Fuel Process Technol. 2014;119:173–184. doi: 10.1016/j.fuproc.2013.11.002.
- Molaei Dehkordi A, Ghasemi M. Transesterification of waste cooking oil to biodiesel using Ca and Zr mixed oxides as heterogeneous base catalysts. Fuel Process Technol. 2012;97:45–51. doi: 10.1016/j.fuproc.2012.01.010.
- Taufiq-Yap YH, Hwa S, Rashid U, et al. Transesterification of Jatropha curcas crude oil to biodiesel on calcium lanthanum mixed oxide catalyst: effect of stoichiometric composition. Energy Convers Manag. 2014;88:1290–1296. doi: 10.1016/j.enconman.2013.12.075.
- Kouzu M, Kasuno T, Tajika M, et al. Calcium oxide as a solid base catalyst for transesterification of soybean oil and its application to biodiesel production. Fuel. 2008;87(12):2798–2806. doi: 10.1016/j.fuel.2007.10.019.
- Al-Muhtaseb AH, Osman AI, Jamil F, et al. Integrating life cycle assessment and characterisation techniques: a case study of biodiesel production utilising waste Prunus armeniaca seeds (PAS) and a novel catalyst. J Environ Manage. 2022;304:114319. doi: 10.1016/j.jenvman.2021.114319.
- D'Cruz A, Kulkarni MG, Meher LC, et al. Synthesis of biodiesel from canola oil using heterogeneous base catalyst. J Am Oil Chem Soc. 2007;84(10):937–943. doi: 10.1007/s11746-007-1121-x.
- Hanif S, Alsaiari M, Ahmad M, et al. Membrane reactor based synthesis of biodiesel from Toona ciliata seed oil using barium oxide nano catalyst. Chemosphere. 2022;308(Pt 3):136458. doi: 10.1016/j.chemosphere.2022.136458.
- Jeon H, Kim DJ, Kim SJ, et al. Synthesis of mesoporous MgO catalyst templated by a PDMS–PEO comb-like copolymer for biodiesel production. Fuel Process Technol. 2013;116:325–331. doi: 10.1016/j.fuproc.2013.07.013.
- Saman S, Balouch A, Talpur FN, et al. Green synthesis of MgO nanocatalyst by using Ziziphus mauritiana leaves and seeds for biodiesel production. Verpoort App Organomet Chem. 2021;35:1–10. doi: 10.1002/aoc.6199.
- Hassan HMA, Alhumaimess MS, Alsohaimi IH, et al. Aldosari, biogenic-mediated synthesis of the Cs2O–MgO/MPC nanocomposite for biodiesel production from olive oil. ACS Omega. 2020;5(43):27811–27822. doi: 10.1021/acsomega.0c02814.
- Munir M, Saeed M, Ahmad M, et al. Cleaner production of biodiesel from novel non-edible seed oil (Carthamus lanatus L.) via highly reactive and recyclable green nano CoWO3@ rGO composite in context of green energy adaptation. Fuel. 2023;332:126265. doi: 10.1016/j.fuel.2022.126265.
- Yang W. Effect of nitrogen, phosphorus and potassium fertilizer on growth and seed germination of Capsella bursa-pastoris (L.) Medikus. J Plant Nutr. 2018;41(5):636–644. doi: 10.1080/01904167.2017.1415350.
- Satar S, Kavallieratos NG, Tüfekli M, et al. Capsella bursa-pastoris is a key overwintering plant for aphids in the mediterranean region. Insects. 2021;12(8):744. doi: 10.3390/insects12080744.
- Ahmed HT, Francis A, Clements DR, et al. The biology of Canadian weeds. 159. Capsella bursa-pastoris (L.) Medik. Can J Plant Sci. 2021;102(3):529–552.
- Wahab R, Ansari SG, Dar MA, et al. Synthesis of magnesium oxide nanoparticles by sol-gel process. Mater Sci Forum. 2007;558–559:983–986. doi: 10.4028/www.scientific.net/MSF.558-559.983.
- Ashok A, Kennedy LJ, Vijaya JJ, et al. Optimization of biodiesel production from waste cooking oil by magnesium oxide nanocatalyst synthesized using coprecipitation method. Clean Techn Environ Policy. 2018;20(6):1219–1231. doi: 10.1007/s10098-018-1547-x.
- Ullah K, Jan HA, Ahmad M, et al. Synthesis and structural characterization of biofuel from Cocklebur sp., using zinc oxide nano-particle: a novel energy crop for bioenergy industry. Front Bioeng Biotechnol. 2020;8:756. doi: 10.3389/fbioe.2020.00756.
- Birla A, Singh B, Upadhyay SN, et al. Kinetics studies of synthesis of biodiesel from waste frying oil using a heterogeneous catalyst derived from snail shell. Bioresour Technol. 2012;106:95–100. doi: 10.1016/j.biortech.2011.11.065.
- Jan HA, Surina I, Saqib NU, et al. Application of recycled nickel-oxide nanoparticles for biodiesel synthesis from the non-edible seed oil of Acacia farnesiana. Biofuels. 2023:1–11. doi: 10.1080/17597269.2023.2294225.
- Uğuz G, Atabani AE, Mohammed MN, et al. Fuel stability of biodiesel from waste cooking oil: a comparative evaluation with various antioxidants using FT-IR and DSC techniques. Biocatal Agric Biotechnol. 2019;21:101283. doi: 10.1016/j.bcab.2019.101283.
- Zhang Y, Ma M, Zhang X, et al. Synthesis, characterization, and catalytic property of nanosized MgO flakes with different shapes. J Alloys Compd. 2014;590:373–379. doi: 10.1016/j.jallcom.2013.12.113.
- Al Zubi MA, Jayaganthan A, Alimuddin, et al. Reduction of carbon-based emissions using MgO nanoparticle with waste cooking oil biodiesel in diesel engine. Adsorp Sci Tech. 2022;2022. doi: 10.1155/2022/9259173.
- Wilson K, Hardacre C, Lee AF, et al. The application of calcined natural dolomitic rock as a solid base catalyst in triglyceride transesterification for biodiesel synthesis. Green Chem. 2008;10(6):654–665. doi: 10.1039/b800455b.
- Jan HA, Saqib NU, Aamir A, et al. Aleurites moluccana as a potential non-edible feedstock for industrial-scale biodiesel synthesis using homemade zinc oxide nanoparticles as a catalyst. Waste Biom Valor. 2023;15:1081–1095. doi: 10.1007/s12649-023-02238-w.
- Meher LC, Vidya Sagar D, Naik SN. Technical aspects of biodiesel production by transesterification—a review. Renew Sustain Energy Rev. 2006;10(3):248–268. doi: 10.1016/j.rser.2004.09.002.
- Seenuvasan M, Kalai Selvi P, Anil Kumar M, et al. Standardization of non-edible Pongamia pinnata oil methyl ester conversion using hydroxyl content and GC–MS analysis. J Taiwan Inst Chem Eng. 2014;45(4):1485–1489. doi: 10.1016/j.jtice.2013.11.002.
- Miao X, Wu Q. Biodiesel production from heterotrophic microalgal oil. Bioresour Technol. 2006;97(6):841–846. doi: 10.1016/j.biortech.2005.04.008.
- Jan HA, Osman AI, Šurina I, et al. Recycling calcium oxide nanoparticles for sustainable biodiesel production from nonedible feedstock Argemone mexicana L. Biofuels. 2023:1–10. doi: 10.1080/17597269.2023.2277989.
- Jan HA, Al-Fatesh AS, Osman AI, et al. Assessment of Ailanthus altissima seed oil as a potential source for biodiesel production using nickel oxide nanoparticles catalyst. JKSUS. 2024;36(3):103084. doi: 10.1016/j.jksus.2023.103084.
- Dhawane SH, Karmakar B, Ghosh S, et al. Parametric optimisation of biodiesel synthesis from waste cooking oil via Taguchi approach. J Environ Chem Eng. 2018;6(4):3971–3980. doi: 10.1016/j.jece.2018.05.053.
- De S, Pessoa Junior WAG, Sá ISC, et al. Pineapple (Ananás comosus) leaves ash as a solid base catalyst for biodiesel synthesis. Bioresour Technol. 2020;312:123569. doi: 10.1016/j.biortech.2020.123569.
- Niju S, Meera Sheriffa Begum KM, Anantharaman N. Enhancement of biodiesel synthesis over highly active CaO derived from natural white bivalve clam shell. Arab J Chem. 2016;9(5):633–639. doi: 10.1016/j.arabjc.2014.06.006.
- Zhang Y, Dubé MA, McLean DD, et al. Biodiesel production from waste cooking oil: 2. Economic assessment and sensitivity analysis. Bioresour Technol. 2003;90(3):229–240. doi: 10.1016/S0960-8524(03)00150-0.
- Gebremariam SN, Marchetti JM. Economics of biodiesel production. Energy Convers Manag. 2018;168:74–84. doi: 10.1016/j.enconman.2018.05.002.
- Ávila Vázquez V, Díaz Estrada RA, Aguilera Flores MM, et al. Transesterification of non-edible castor oil (Ricinus communis L.) from Mexico for biodiesel production: a physicochemical characterization. Biofuels. 2020;11:1–10. doi: 10.1080/17597269.2020.1787700.
- Ma Y, Wang Q, Sun X, et al. Kinetics studies of biodiesel production from waste cooking oil using FeCl3-modified resin as heterogeneous catalyst. Renew Energy. 2017;107:522–530. doi: 10.1016/j.renene.2017.02.007.
- Al-Samaraae RR, Atabani AE, Uguz G, et al. Perspective of safflower (Carthamus tinctorius) as a potential biodiesel feedstock in Turkey: characterization, engine performance and emissions analyses of butanol–biodiesel–diesel blends. Biofuels. 2020;11(6):715–731. doi: 10.1080/17597269.2017.1398956.
- Kaisan MU, Anafi FO, Nuszkowski J, et al. Calorific value, flash point and cetane number of biodiesel from cotton, jatropha and neem binary and multi-blends with diesel. Biofuels. 2020;11(3):321–327. doi: 10.1080/17597269.2017.1358944.
- Khan MA, Blackshaw RE, Marwat KB. Biology of milk thistle (Silybum marianum) and the management options for growers in north-western Pakistan. Weed Biol Manag. 2009;9(2):99–105. doi: 10.1111/j.1445-6664.2009.00326.x.
- Knothe G. Improving biodiesel fuel properties by modifying fatty ester composition. Energy Environ Sci. 2009;2(7):759–766. doi: 10.1039/b903941d.
- Mofijur M, Masjuki HH, Kalam MA, et al. Effect of biodiesel-diesel blending on physico-chemical properties of biodiesel produced from moringa oleifera. Proced Eng. 2015;105:665–669. doi: 10.1016/j.proeng.2015.05.046.
- Pullen J, Saeed K. An overview of biodiesel oxidation stability. Renew Sustain Energy Rev. 2012;16(8):5924–5950. doi: 10.1016/j.rser.2012.06.024.
- Sia CB, Kansedo J, Tan YH, et al. Evaluation on biodiesel cold flow properties, oxidative stability and enhancement strategies: a review. Biocatal Agric Biotechnol. 2020;24:101514. doi: 10.1016/j.bcab.2020.101514.
- Bannister CD, Chuck CJ, Bounds M, et al. Oxidative stability of biodiesel fuel. Proceed Inst Mech Eng Part D J Auto Eng. 2011;225(1):99–114. doi: 10.1243/09544070JAUTO1549.
- Kumar N. Oxidative stability of biodiesel: causes, effects and prevention. Fuel. 2017;190:328–350. doi: 10.1016/j.fuel.2016.11.001.
- Fregolente PBL, Fregolente LV, Wolf MacIel MR. Water content in biodiesel, diesel, and biodiesel–diesel blends. J Chem Eng Data. 2012;57(6):1817–1821. doi: 10.1021/je300279c.
- Cardoso LC, Almeida FNC, Souza GK, et al. Synthesis and optimization of ethyl esters from fish oil waste for biodiesel production. Renew Energy. 2019;133:743–748. doi: 10.1016/j.renene.2018.10.081.
- Lugo-Méndez H, Sánchez-Domínguez M, Sales-Cruz M, et al. Synthesis of biodiesel from coconut oil and characterization of its blends. Fuel. 2021;295:120595. doi: 10.1016/j.fuel.2021.120595.
- Demirbaş A. Biodiesel fuels from vegetable oils via catalytic and non-catalytic supercritical alcohol transesterifications and other methods: a survey. Energy Convers Manag. 2003;44(13):2093–2109. doi: 10.1016/S0196-8904(02)00234-0.
- Anand K, Ranjan A, Mehta PS. Estimating the viscosity of vegetable oil and biodiesel fuels. Energy Fuels. 2010;24(1):664–672. doi: 10.1021/ef900818s.
- Demirbaş A. Production of biodiesel from algae oils. Energy Sources Part A Recov Util Environ Eff. 2009;31(2):163–168. doi: 10.1080/15567030701521775.
- Adewuyi A, Awolade PO, Oderinde RA. Hura crepitans seed oil: an alternative feedstock for biodiesel production. J Fuels. 2014;2014:1–8. doi: 10.1155/2014/464590.
- Knothe G. Monitoring a progressing transesterification reaction by fiber‐optic near infrared spectroscopy with correlation to 1H nuclear magnetic resonance spectroscopy. J Am Oil Chem Soc. 2000;77(5):489–493. doi: 10.1007/s11746-000-0078-5.
- Portela NA, Oliveira ECS, Neto AC, et al. Quantification of biodiesel in petroleum diesel by 1H NMR: evaluation of univariate and multivariate approaches. Fuel. 2016;166:12–18. doi: 10.1016/j.fuel.2015.10.091.
- Ullah K, Ahmad M, Qiu F, et al. Assessing the experimental investigation of milk thistle oil for biodiesel production using base catalyzed transesterification. Energy. 2015;89:887–895. doi: 10.1016/j.energy.2015.06.028.
- Miglio R, Palmery S, Salvalaggio M, et al. Microalgae triacylglycerols content by FT-IR spectroscopy. J Appl Phycol. 2013;25(6):1621–1631. doi: 10.1007/s10811-013-0007-6.
- Atabani AE, Shobana S, Mohammed MN, et al. Integrated valorization of waste cooking oil and spent coffee grounds for biodiesel production: blending with higher alcohols, FT–IR, TGA, DSC and NMR characterizations. Fuel. 2019;244:419–430. doi: 10.1016/j.fuel.2019.01.169.
- Soon LB, Rus AZM, Hasan S. Continuous biodiesel production using ultrasound clamp on tubular reactor. Int J Autom Mech Eng. 2013;8:1396–1405. doi: 10.15282/ijame.8.2013.27.0115.
- Nandiyanto ABD, Oktiani R, Ragadhita R. How to read and interpret FTIR spectroscope of organic material. Indo J Sci Technol. 2019;4(1):79–118. doi: 10.17509/Ijost.V4i1.15806.
- Siatis NG, Kimbaris AC, Pappas CS, et al. Improvement of biodiesel production based on the application of ultrasound: monitoring of the procedure by FTIR spectroscopy. J Am Oil Chem Soc. 2006;83(1):53–57. doi: 10.1007/s11746-006-1175-1.
- Araya F, Troncoso E, Mendonça RT, et al. Condensed lignin structures and re-localization achieved at high severities in autohydrolysis of Eucalyptus globulus wood and their relationship with cellulose accessibility. Biotechnol Bioeng. 2015;112(9):1783–1791. doi: 10.1002/bit.25604.
- Andrade TA, Errico M, Christensen KV. Transesterification of castor oil catalyzed by liquid enzymes: optimization of reaction conditions. Comput Aided Chem Eng. 2017;40:2863–2868. doi: 10.1016/B978-0-444-63965-3.50479-7.
- Elango RK, Sathiasivan K, Muthukumaran C, et al. Transesterification of castor oil for biodiesel production: process optimization and characterization. Microchem J. 2019;145:1162–1168. doi: 10.1016/j.microc.2018.12.039.
- Asci F, Aydin B, Akkus GU, et al. Fatty acid methyl ester analysis of Aspergillus fumigatus isolated from fruit pulps for biodiesel production using GC-MS spectrometry. Bioengineering. 2020;11(1):408–415. doi: 10.1080/21655979.2020.1739379.