Abstract
Cancer is a main public health problem that is known as a malignant tumor and out-of-control cell growth, with the potential to assault or spread to other parts of the body. Recently, remarkable efforts have been devoted to develop nanotechnology to improve the delivery of anticancer drug to tumor tissue as minimizing its distribution and toxicity in healthy tissue. Nanotechnology has been extensively used in the advance of new strategies for drug delivery and cancer therapy. Compared to customary drug delivery systems, nano-based drug delivery method has greater potential in different areas, like multiple targeting functionalization, in vivo imaging, extended circulation time, systemic control release, and combined drug delivery. Nanofibers are used for different medical applications such as drug delivery systems.
Introduction
Cancer is a main public health problem and one of the world’s most disturbing diseases. Systemic chemotherapy along with surgical resection or radiotherapy is the most generally used therapeutic strategy for cancer. A combination of radiation therapy and chemotherapy is commonly applied to raise survival rates of patients. However, the absence of selectivity for tumor tissues often leads to some adverse effect for the patients who undergo a chemotherapeutic method, including kidney malfunction, nausea and vomiting, nerve injury, impairment of sight, and bone marrow suppression (Saravanabhavan and Dharmalingam Citation2013, Zong et al. Citation2015). Although some progress has been made in cancer therapy, there are still numerous limitations including severe toxicity in normal cells, death caused by the systematic administration of anticancer drugs at extreme endurable doses (Piccolo and Kolesar Citation2014), limited distribution of chemotherapy drugs from the blood vessels into the solid tumors, (Postma et al. Citation2013) low resection rates and poor overall patient survival in surgery, (Langer Citation1998) and prominent clinical toxicities in radiotherapy (Minchinton and Tannock Citation2006). Recently, remarkable efforts have been devoted to develop nanotechnology to improve the delivery of anticancer drug to tumor tissue as minimizing its distribution and toxicity in healthy tissue (Panyam and Labhasetwar Citation2003). Nanotechnology has been extensively used in the advance of new strategies for drug delivery and cancer therapy. Compared to customary drug delivery systems, nano-based drug delivery method have greater potential in different areas, like multiple targeting functionalization, in vivo imaging, extended circulation time, systemic control release, and combined drug delivery (Liu et al. Citation2014). Polymeric nanofibers refers to fibers with diameters from 1 nm to 1 μm, closely matching the size scale of extracellular matrix (ECM) fibers (Dahlin et al. Citation2011, Ma and Zhang Citation1999). These nanomatertials are made of inorganic (i.e., titanium, silicon or aluminum oxides) or organic (polyvinyl alcohol, gelatin, poly(N-isopropylacrylamide, polycaprolactone, or polyurethane) materials. Nanofibers consist of low density, large surface area, tight pore size, and high pore volume (Díaz and Vivas-Mejia Citation2013). Nanofibers are used for different medical applications such as drug delivery systems. For instance, in one research Tseng and coworkers used biodegradable nanofibers to successfully deliver vancomicyn, an antibiotic, to the brain tissue of rats and decrease the toxicity related with parenteral antibiotic treatment (Tseng et al. Citation2013). Drug or other therapeutic agents may be integrated into electrospun nanofibers during blend electrospinning, chemical immobilization, co-axial electrospinning, physical adsorption, and emulsion electrospinning () (Huang et al. Citation2003).
Figure 1. Drug incorporation techniques (Goonoo et al. Citation2014).
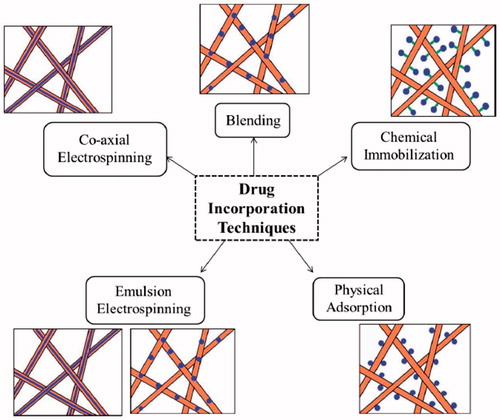
Nanofiber in cancer therapy
More recently, nano carrier-based drug delivery systems have attracted more and more attention in overwhelming these limitations (Ferrari Citation2005, Jemal et al. Citation2006, Moses et al. Citation2003, O'Gorman et al. Citation2014, Peer et al. Citation2007). Newly, drug-eluting electrospun nanofibers as a novel dosage form have excited much exploration interest because they exhibited following benefits: high loading and encapsulation efficiency for physico-chemically diverse drugs; relatively prolonged residence time; desirable distribution and delivery of the active substance for an extended period at a predictable percentage; typical softness, flexibility, non-abrasion, and lack of sharp corners which enable them to realize various geometries (sheets, tubes, coatings) to fit the lesions; and finally low cost and easy application. These innovative systems have the capability to selectively carry drugs into the cancer tissues or into the targeted sites, which is in contrast to systematic treatment with free drugs. However, the efficacy of drug delivery and subsequently the specific selective effects are still main challenges of these intravenously delivered chemotherapeutics. Among these nanocarriers, polymeric micelles with distinct core/shell architecture which are self-assembled from amphiphilic copolymers have been extensively reported (Farokhzad and Langer Citation2009, Machado et al. Citation2013, Malcolm et al. Citation2010, Newland et al. Citation2012). They are in the center of attention because their exceptional advantages, such as the ability to improve the solubility of water insoluble drugs, prolonged blood circulation, and the easiness of functionalization (Blakney et al. Citation2013, Farokhzad and Langer Citation2009, Liu et al. Citation2013, Machado et al. Citation2013, Zhang et al. Citation2014). However, these nanocarriers will encounter various obstacles in route from the injection location to the target cell, such as mucosal barriers and nonspecific uptake (Kazunori et al. Citation1993, Zamani et al. Citation2013). Thus, the intravenously delivered micelles still show some defects, including fast clearance from the bloodstream, over gathering in non-target organs (Fox et al. Citation2009, Wang et al. Citation2012). Overreliance on the enhanced permeability and retention effect to deliver the nanocarrier into the tumor, and a modest increase in tumor accumulation (Nicolas et al. Citation2013). Unfortunately, the micelles’ advantage-prolonging blood circulation, occasionally turns into a disadvantage that may result in extravasation of the encapsulated containing in unexpected locations due to the low stability of the micellar system (Moses et al. Citation2003).
To address some of these difficulties, localized drug delivery to the solid tumors is a worthy approach. By comparison to the systemic administration, the localized system has some gains such as ensuring prolonged therapeutic drug levels at the tumor location. Additionally, maintaining low systemic drug exposure, (Allen and Cullis Citation2004, Basarkar and Singh Citation2009) not only results in greater therapeutic efficacy and a lower toxicity, (Wang et al. Citation2014) but also reduces the requirement for repeating chemotherapeutic administrations and subsequently improving the quality of life and patient compliance (Allen and Cullis Citation2004).
In one efficacious previous study, implantable wafers found was on a polyanhydride polymer used to locally deliver chemotherapeutic drugs such as carmustine (BCNU) to treat brain cancer (Peer et al. Citation2007). The implantable bulk materials (like blocks, films, and wafers) and the conventional injectable hydrogel system are the most common forms for conventional localized drug delivery systems. For the implantable bulk materials, it is difficult to tune degradation rate. Conventional injectable hydrogel systems, which may improve patient compliance and ease, can be generally divided into two classifications including particle drug depots and semisolid drug depots (Couvreur and Vauthier Citation2006). The particle drug depots, (including emulsions, liposomes, biodegradable microspheres, and micelles) are relatively unstable and easy to migrate away from the tumor location. For the semisolid drug depots, the in vivo solidification of liquid hydrogel is inconvenient occasionally, and primary burst of drug may happen during the lag time between the injection and the formation of the solid hydrogel (Couvreur and Vauthier Citation2006).
Currently, the challenges of this localized drug delivery via polymers are the absence of control in drug release and distribution (Allen and Cullis Citation2004). And particularly targeting at tumor cells. Hence, the combination of the active targeting micellar system with the implantable “controllable” matrix may be a good choice to reach a high chemotherapy efficacy against tumors along with low side effects in normal tissues, and also to overcome the weaknesses of the conventional localized drug delivery systems.
In previous studies, researchers innovated a newly implantable active-targeting micelle-in-nanofiber device for efficient and harmless cancer therapy (Yang et al. Citation2015). This device can be prepared as illustrated in .
Figure 2. Schematic illustrations of the fabrication of the implantable active-targeting micelle-in-nanofiber device (FM-Nanofiber) and the delivery process of these Dox-loaded micelles (FM) from nanofiber matrix to tumor tissues and finally to tumor cells (Yang et al. Citation2015).
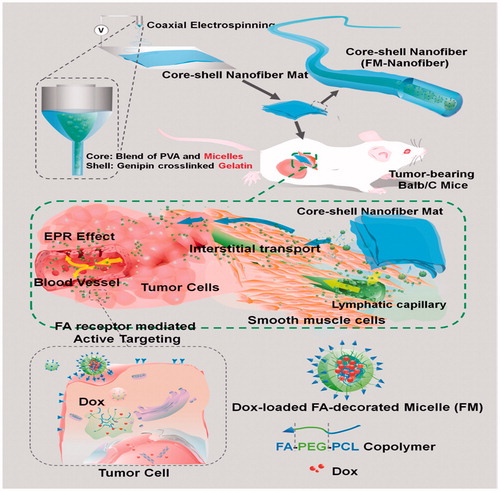
First, the folate (FA)-conjugated polyɛ-caprolactone-polyethylene glycol (FA_PCL-PEG) copolymer was used to encapsulate doxorubicin (Dox), the anticancer drug model, by self-assembling into active-targeting micelles (FM). FA ligands can directly bind to the folate receptors (FR) that are over expressed on the surface of a majority of solid tumors (Low and Kularatne Citation2009). Then, these micelles are trapped in the core area of the core_shell polymeric nanofibers by coaxial electrospinning in which the inner phase is a mixture water solution of poly(vinyl alcohol) (PVA) and the micelles, and the outer phase is a gelatin solution. Electrospun fibers are identified to be excellent drug carriers with a higher surface area per mass, high drug loading, and encapsulation efficiency (Chakraborty et al. Citation2009, Joshi et al. Citation2014, Szentivanyi et al. Citation2011). They also have the potential as an implantable device for cancer chemotherapy of solid tumors either in the tumor or at the surgical resection margins (Kim et al. Citation2013, Ranganath and Wang Citation2008, Ranganath et al. Citation2010).
In summary, they have effectively developed a new localized drug delivery device by combination of active-targeting micellar system with implantable polymeric nanofibers (Madan et al. Citation2009). To date, many approaches have been proposed for the delivery of drugs, lysozymes, growth factors, and genes and siRNAs using forms of grapheme, polymers, and blends of these. It has been reported that these delivery systems have been used in diverse applications and post-surgical cancer therapies. Numerous drug delivery methods such as hydrogels, nanoparticles, polymer-drug conjugates, and microspheres have been advanced, which each of possesses its own advantages and disadvantages (Arnold and Ulbrich-Hofmann Citation2006, Charernsriwilaiwat et al. Citation2012, Chaturvedi et al. Citation2011, Citation2013, Kawashima et al. Citation2011, Neves et al. Citation2012, Rudzinski and Aminabhavi Citation2010). Of the diverse drug delivery systems, polymer nanofiber-drug conjugates have drawn interest between the scientists, as it could be delivered to targeted locations by passive or active targeting (Letchford and Burt Citation2007, Mora-Huertas et al. Citation2010).
A lot of systems have been developed for the administration of the drug, in which the polymeric drug-delivery systems have various merits compared to other conventional methods. Some of these advantages include dosage forms, therapeutic influence, non-toxicity, degradability, and so on. Despite these benefits, there are also some demerits, such as the low effectiveness of the drug delivery and burst release of drug at the beginning (Sokolsky-Papkov et al. Citation2007). The limitations associated with the existing treatment models and the requirement to overcome them acted as the catalyst for operating further investigation in these areas. In current years, electro spinning has gathered a lot of interest in the invention of ultrafine polymer fibers of diameters ranging from 20 to 2000 nm, as their fundamental properties provide an excellent environment for cell adhesion, proliferation, and differentiation. Some researchers have proposed the use of the fibers as an alternative for fabricating vascular scaffolds because of its low cost productivity, facile control of fiber diameter, and easiness in the setup. Furthermore, it mimics the natural ECM at nanoscale while possessing brilliant mechanical properties (Lin et al. Citation2013, Zeng et al. Citation2003).
It was obvious from the works of numerous researchers that the nanofiber scan be applied as typical carriers of the drug as they possess higher surface to the volume ratio along with improved antitumor efficacy and antimicrobial activity. Apart from drug delivery, much attention has been concentrated on the field of tissue engineering for maintenance and stimulation of tissue growth. This has always remained a very challenging application since the materials that are selected play a vital role. The materials used for tissue engineering must be biocompatible, biodegradable, non-toxic, and less immunogenic; moreover, it should be safe to implant or deliver the drug. Besides, it should also possess appropriate mechanical properties and various other features that are required for drug delivery and tissue engineering applications (Peter et al. Citation2010, Zheng et al. Citation2013a, Citation2013b).
In a study conducted by Saravanabhavan and Dharmalingam (Citation2013), scientists concluded that PSunanofibers were successfully fabricated with the incorporation of nHA into them by using the electro spinning technique as post-surgical implants in cancer therapy. The system exhibited a high potential in delivering the anticancer drugs to the target site, and it was also demonstrated that it could be used as a postsurgical implant.
In vitro studies on the drug release showed the prolonged pattern of release which allowed the spread of the drugs inside the target location wherever the tumor may exist. The results from the MTT assay showed that the metabolizing activity of the normal cells was not affected by the PSu; but the drug loaded composites decreased the metabolic activity of the cancerous cells. Thus, the prepared model could potentially be a carrier for anticancer drug, and it could also serve as a postsurgical implant (Ahmadi et al. Citation2014, Ebrahimnezhad et al. Citation2013, Mollazade et al. Citation2013, Nejati-Koshki et al. Citation2013, Pourhassan-Moghaddam et al. Citation2013, Saravanabhavan and Dharmalingam Citation2013, Zong et al. Citation2015).
In a study conducted by Zong et al. (Citation2015), cisplatin-loaded poly (ethylene oxide)/polylactide composite electrospun nanofibers were prepared to seek the possibility and feasibility of nanofibers-based vaginal drug delivery system for local chemotherapy against the cervical cancer (Abbasi et al. Citation2014a, Alimirzalu et al. Citation2014, Alizadeh et al. Citation2014, Davoudi et al. Citation2014, Eatemadi et al. Citation2014, Ghasemali et al. Citation2013, Hosseininasab et al. Citation2014, Kouhi et al. Citation2014). The results showed that drug-loaded nanofibers is a hopeful dosage form for local therapy of cervical/vaginal cancers. It is appropriate for the treatment of incurable cervical/vaginal cancers, or even better as an auxiliary selection to surgical resection of these cancers (Abbasi et al. Citation2014b, Alizadeh et al. Citation2016, Chung et al. Citation2016, Daraee et al. Citation2014, Davoudi et al. Citation2014, Eatemadi et al. Citation2016, Effat et al. Citation2016, Elham et al. Citation2014, Eommolbanin et al. Citation2014, Fekri Aval et al. Citation2016, Hadis et al. Citation2016, Jin-Hwan et al. Citation2014, Mohammad et al. Citation2014, Nasrabadi et al. Citation2016, Tabatabaei Mirakabad et al. Citation2016).
Prevention of lung cancer recurrence by nanoparticle (nanofiber) technology
Lung cancer is the chief reason of cancer deaths in North America, with above 200,000 new cases identified every year and a gloomy five-year survival percentage of ∼18% (Mollazade et al. Citation2013, Zong et al. Citation2015). One of the factors causative to poor survival is the lack of ability of a lot of patients to bear a “wide” local cutting out of their tumor, i.e., lobectomy, since elimination of the predictable 25% of whole lung function additional compromises previously restricted pulmonary function. Lesser, i.e., wedge, resections save lung parenchyma but are connected with a two-fold rise in local cancer return as an outcome of the microscopic disease remaining at the surgical resection border (Ebrahimnezhad et al. Citation2013, Nejati-Koshki et al. Citation2013). This is a serious choice since recent two-year survival in patients that grow recurrence drops to ∼20% (Pourhassan-Moghaddam et al. Citation2013) for instance the mainstream of these patients are not candidates for added surgery, and radiation and/or chemotherapy are largely palliative (Ahmadi et al. Citation2014). Platinum based DNA-adducting agents, for instance cisplatin, are the in progress standard-of-care chemotherapy for lung cancer (Ghasemali et al. Citation2013, Kouhi et al. Citation2014). Even though these agents have dose-limiting side-effects such as nephrotoxicity (Abbasi et al. Citation2014a) and neurotoxicity (Hosseininasab et al. Citation2014) with systemic management, the expenditure of cisplatin has reached some enhancement in overall survival of lung cancer patients with metastatic disease. Cisplatin has furthermore been used in mixture therapy (Alimirzalu et al. Citation2014, Davoudi et al. Citation2014, Ghasemali et al. Citation2013, Kouhi et al. Citation2014) to attain a wider therapeutic window and therefore enhanced clinical results. Additional methods to increase cisplatin efficacy in vivo are also being explored. Nanoparticles and local drug delivery approaches such as chemotherapy-loaded films, foams, and gels are altogether being advanced to develop drug uptake while reducing systemic side effects (Eatemadi et al. Citation2014). In specific, cisplatin-loaded nanoparticles have been assessed in numerous clinical trials with hopeful outcomes, (Alizadeh et al. Citation2014, Citation2016) and other cisplatin drug delivery materials such as gels (Eatemadi et al. Citation2016), films (Davoudi et al. Citation2014), and glues (Effat et al. Citation2016) intended for local administration are gaining traction in the fight against lung and associated thoracic cancers. Though, a lot of local and systemic drug delivery systems possess burst release kinetics, which exposes drugs to tumors for merely a short period and highpoints the requirement for developed designs for sustained-release chemotherapy depots. In recent times, informed the fabrication of three-dimensional superhydrophobic microfiber meshes that make use of the metastable air barrier within these porous materials to drastically slow wetting and thus tolerate the release of encapsulated 7-ethyl-10-hydroxycamptothecin (HCPT) (Elham et al. Citation2014), an investigational lipophilic anticancer agent, for some weeks. Knowing the central character of cisplatin therapy in the treatment of lung cancer, this report concentrates on efforts using superhydrophobic materials to deliver this hydrophilic drug. Exactly, the current report defines the fabrication of cisplatin-loaded, three dimensional nanofiber meshes; reveals the sustained release of cisplatin in vitro; and applies the satisfactory physical and mechanical stuffs of these biodegradable meshes to an in vivo surgical model of destructive, initial-stage lung cancer, and local post-surgical cancer recurrence (Eommolbanin et al. Citation2014). Given the requirement to reduce the amount of lung removed and yet accomplish histologically negative margins, a local drug delivery tactic that supplements cytoreductive surgery with localized, sustained-release chemotherapy possibly will hold capacity by removing residual, microscopic tumor cells – mainly in patients impotent to endure more destructive resection. Such a local drug-delivery method is presently clinically implemented in the treatment of single one cancer: high-grade malignant glioma (Hadis et al. Citation2016, Tabatabaei Mirakabad et al. Citation2016). Afterward cytoreductive surgery, rigid, brittle carmustine (BCNU)-loaded polyanhydride (Daraee et al. Citation2014) wafers are located in the resected tumor beds, with BCNU release happening over 2–3 weeks (Nasrabadi et al. Citation2016). Aimed at patients with lately identified malignant glioma, this treatment improved their median survival to 64.1 weeks, in comparison to 49.4 weeks with placebo (Chung et al. Citation2016, Nasrabadi et al. Citation2014). Additional success has been limited by the short time of drug release in this delivery system. Unlike static tissues, the practical difference of revocable tissue extension and contraction present on the lung surface requires the use of compliant materials for drug delivery. Hence, the design features for drug delivery to the lung requires a compliant, flexible material with a prolonged release profile. Therefore, formerly advanced paclitaxel-loaded PGC-C18 films for preventing local cancer recurrence in a parallel Lewis Lung Carcinoma (LLC) tumor resection model (Abbasi et al. Citation2014b). These films depend on their hydrophobicity to arrange for sustained drug release over ∼90 days for lipophilic drugs (such as paclitaxel (Elham et al. Citation2014b)). Though, these films lacked the mechanical integrity to serve as buttressing materials themselves and were thus cast atop secondary scaffolds composed of de-cellularized bovine pericardium strips. In order to fabricate an all-in-one drug-device system that may well be stapled into the lung tissue by standard surgical staplers, as was designated the method of electrospinning. Electrospinning is a polymer processing procedure (Fekri Aval et al. Citation2016, Mohammad et al. Citation2014, Sill and von Recum Citation2008) that generates non-woven fiber meshes and involves the application of high voltage to a polymer solution at the tip of a syringe pump and needle assembly.
The communal chloroform/methanol solvent system for electrospinning PCL-based meshes was evaded for the reason that cisplatin is unsolvable in such nonpolar solvents, and solvent-drug compatibility has been exposed to affect drug release degrees and/or outcome in poor encapsulation (Zeng et al. Citation2005).
Nanofiber and tumor of central nervous system
Such as in other organs, malignant tumors are one of the greatest regularly studied target diseases of nanotherapeutics amongst brain diseases. Particularly, numerous pathological mechanisms as well as reactive oxygen species (ROS), biological actions of growth factors, and signaling pathways of proliferative potentials could be addressed with nanoparticle-based therapeutics (Arvizo et al. Citation2013, Kudgus et al. Citation2013). Furthermore, double functions of nanoparticles in together imaging and therapy, so called theranostics, are still attractive notions (Muthu et al. Citation2014). A prominent features of nanoparticles is that it is possible to increase bioavailability of therapeutic agents in brain tumors by way of conjugation of specific ligands on the surface of nanoparticles which are loaded with therapeutic materials. BBB could be a difficulty to therapeutic agents along with toxic materials (Kim et al. Citation2006). Bioavailability can be improved by ligand revision with peptides targeting cell surface receptors which are abundant in endothelial cells lining brain vasculatures, such as transferrin receptor and low-density lipoprotein receptor (Kuang et al. Citation2013, Zhang et al. Citation2013). Therapeutic materials as well as conventional chemotherapeutic agents and minor interfering RNA could be loaded in nanoparticles. Furthermore, ligand adjusted nanoparticles develops cellular uptake of therapeutic materials into malignant tumor cells by conjugation with ligands which fix to surface molecules specific to glioma cells (Gao et al. Citation2013). Packing with two or extra therapeutic materials into nanoparticles is also a plausible approach in the treatment of brain tumor (Lei et al. Citation2013).
Glioblastoma multiforme (GBM) accounts for approximately 50% of Reported malignant brain tumors (Wen and Kesari Citation2008). GBM is a prime tumor of astrocytes that, in spite of years of investigation, remains resistant to treatment even with improvements in surgical methods, neuroimaging, and adjuvant modalities for instance chemotherapy and radiation (Bao et al. Citation2006, Sarkar and Chiocca Citation2011). Clinical observations recommend that these tumors migrate by way of single cells, chiefly along wihite matter tracts (Bellail et al. Citation2004, Louis Citation2006). Conventionally, cancer cell migration has been evaluated using a number of two-dimensional (2D) assays, such as the micro liter migration evaluate (Giese et al. Citation1995, Valster et al. Citation2005) or the wound healing assay (Valster et al. Citation2005). Particular to GBM migration, some brain mimetic hydrogels, as well as hyaluronic acid, have been working by ourselves (Rao et al. Citation2011) and others (Ananthanarayanan et al. Citation2011, Coquerel et al. Citation2009, David et al. Citation2008, Yang et al. Citation2011). Polymeric electrospun nanofibers are substitute neural tissue engineering substrates (Corey et al. Citation2008, Gerardo-Nava et al. Citation2009, Prabhakaran et al. Citation2009) that have been used as conductors for neural reparation and regeneration (Corey et al. Citation2008, Schnell et al. Citation2007, Wang et al. Citation2009) and substrates for Schwann cell maturation (Chew et al. Citation2008) and neural stem cell variation (Christopherson et al. Citation2009). Ranged electrospun nanofibers are mostly fascinating as neural guides because of their topographical resemblance to white matter (Jovanov-Milosevic et al. Citation2006). Moreover, aligned electrospun nanofibers (i.e., poly(ɛ-caprolactone) [PCL]) reproduce the morphological and molecular signs of glioma migration ex vivo (Agudelo-Garcia et al. Citation2011, Johnson et al. Citation2009). These tunable materials have not been employed formerly to survey the character of microenvironment, specifically mechanics and chemistry, on GBM behaviors (Rao et al. Citation2013).
Magnetic nanoparticle-based drug delivery for cancer therapy
Magnetic drug delivery systems
Greatly power has been made to address the diverse locations where tumors can happen and, therefore, numerous methods have been advanced. Magnetic implants are utilized to guide various magnetic drug-delivery applications, generally exploiting blood vessels, but with a little exceptions: One notion is created on intra the cal drug delivery and involves direct drug infusion into the spinal canal. It has become a standard training in order to treat various central nervous system diseases and in major tumors, also, due to the condensed systemic toxicity from the drug bypassing the blood–brain barrier. In order to overcome residual detriments, for example insufficient drug deposition at particular locations, ferric steel implants were located in the subarachnoid space in an in vitro human spine model previous to nanoparticle administration into the spine. The consequences show that this method can improve the targeting abilities for intrathecal drug delivery (Lueshen et al. Citation2015). For treating lung cancer, the expiratory pathway appears to be the logical access for an sufficient targeting approach. Magnetizable aerosols can be used for inhalative magnetic drug targeting in order to improve the drug focus at a certain target place within the lung. Commercially accessible nebulizers are capable at making magnetic nanoparticle suspensions as aerosols for inhalation (Baumann et al. Citation2012). Joining both an implanted stable magnet in terms of dilute micro ferrio magnetic wires inserted within blood vessels and outside useful magnetic fields could provide specific enrichment of concurrently administered ferromagnetic nanoparticles (Hournkumnuard and Natenapit Citation2013). Within this setting, more than 60% of the injected concentration can be gathered in the area of interest. It has been, and still is, problematic to enrich magnetic nanoparticles three-dimensionally in the intersection of dissimilar magnetic fields. Enduring magnets are gaining attention for use in magnetic drug-targeting devices and can display convincing magnetic properties even at great depths deploying Halbach-like magnet arrays (He et al. Citation2014). Additional probability considers fast magnetic pulses on ferromagnetic rods that lead to reversing the indication of the potential energy term in Earnshaw’s theorem, thus enabling a quasi-static, stable trap between magnets. In vitro investigating determine the possibility of making inward-pointing magnetic forces which cause the concentration of ferromagnetic rods on a central target (Nacev et al. Citation2015). An entirely different concept of magnetic drug targeting was done by Taherkani et al. using directional magneto taxis as an alternative to magnetic attraction. Bacteria can perform as self-propelling vehicles for reaching hard-to-treat hypoxic areas in solid tumors (Taherkhani et al. Citation2014). Drug-carrying liposomes were hence attached to the bacterium magnetococcus marinus MC-1 lacking countering the propelling properties. The total goal of the targeting plans should be their translation to clinics, and that depends on the possibility of Implementing both the synthesis of drug-carrying nanoparticles in a quality-controlled environment (GMP manufacturing) and the equipment essential for applying the system that can easily be performed in a clinical environment. The road to clinical implementation can be difficult and lengthy and several sophisticated methods providing very promising in-vitro and in-vivo results may fail under these presuppositions. A convincing concept that is close to clinical implementation is magnetic drug targeting based on intra-arterial administration of drug-loaded SPIONs and enrichment by externally applied magnetic fields that are generated by a tough electromagnet. The resistant of principle was proved in the worldwide major study concerning the positive pre-clinical application of SPIONs for cancer treatment (Tietze et al. Citation2013). In feature, instantaneous MTO-SPIONs injection into the tumor-supplying vessel in rabbits and application of a strong external magnetic field over a V2 squamous cell carcinoma located at the hind leg led to complete tumor remissions without side effects. Through applying only 5e10% of the conservative chemotherapeutic dose, complete tumor remissions were achieved. The distribution profile after MDT displayed 57.2% of the overall recovery of administered drug, with 66.3% of the particles localized in the tumor area, as compared to fewer than 1% of drug and particles reaching the tumor area during conventional intravenous application without magnetic targeting (Tietze et al. Citation2015).
Tumor targeting approaches
In this section, we will argue diverse (active) targeting plans that force the tumor-selective enrichment of active agents. Numerous tumor properties are exploited to improve the buildup of drugs in tumor cells. Generally, they are attributed to particular components on the surface of tumor cells, but there are numerous extra mechanisms. One pattern of particular binding on tumor cells is the tactic of Shvetsov et al. using heat shock protein HSP 70 connected to the surface of SPIONs that are talented to attach to the CD 40 receptor that is expressed on glioma cells (Shevtsov et al. Citation2014). The particular overexpression of Endoglin (CD105) receptors in actively proliferating single-walled carbon nanotube (CNT) that is moreover attributed with Dox as a chemotherapeutic agent. The application in a murine breast cancer model yielded significantly increased cell death (Al Faraj et al. Citation2015). The FA receptor is a very frequently addressed tumor target, as the application of FA-functionalized copolymers of poly(ethylene glycol), subsequent self-assembly into nanoscaled micelles and encapsulation with a hydrophobic model drug and SPIONs indicate (Zhang et al. Citation2014). Many cancer cells overexpress avb3/5 integrins, which can specially identify the short peptide motif Arg–Gly–Asp (RGD). Based on this biological feature of cancer, particle surfaces were spread with this peptide and thus a formulation of siRNA/RGD gold nanoparticles was able to target tumor cells in a lung cancer syngeneic orthotopic murine model (Conde et al. Citation2013). A dissimilar notion exploits the distinct microenvironment in the tumor region as it refers to dynamic cellular and extra-cellular components surrounding tumor cells at each phase of carcinogenesis. Many investigators assume that this aspect is a better mechanism than antibody recognition because of its relative genetic stability with lesser probability for the development of drug resistance. LyP-1, a nine residue peptide, has been exposed to target tumor-associated macrophages. As a result, LyP-1 was spread on hollow mesoporous silica nanoparticles and confirmed improved uptake into MDA-MB-231 cells (Liu et al. Citation2015). Hypoxia is a significant factor of the tumor microenvironment and has been the target of drug discovery efforts for nearly half an era. An remarkable study considers the uptake of charged nanoparticles that were far enlarged for positively charged nanoparticles in comparison to negatively charged ones. Similar phenomena were detected in both iron oxide nanoparticles and gold nanoparticles (Chen et al. Citation2013). These results will be significant for adjusting nanodrug carriers for well uptake efficacy. A novel pH and redox dual-responsive targeting nanoparticle is planned as a drug carrier. The peptide RGDFFFFC is anchored on the surface of mesoporous silica nanoparticles via disulfide bonds, that are redox-responsive, as a gatekeeper as well as a tumor targeting ligand. The peptide and monomethoxypolyethylene glycol (MPEG) with a benzoic-imine bond, which is pH-sensitive, are then connected via “click” chemistry. In vitro cell research shows that the targeting possessions is switched off in typical tissues with a neutral pH, and switched on in tumor tissues with an acidic pH after elimination of the MPEG segment by hydrolysis of the benzoic-imine bond under acidic conditions (Xiao et al. Citation2014).
Use of CNT and lipid nanoparticles based delivery system
Recently in order to prepare novel drug delivery devices, the use of, polymers, silicon materials, carbon materials, and metals has been proposed. The carbon-nanotubes composites and hybrid materials that couple the benefits of polymers (biodegradability and biocompatibility) with those of CNTs (cellular uptake, stability, electromagnatic, and magnetic behavior) are one of the furthermost talented materials in this field.
There are three pre-requisites for a perfect transporter for target drug delivery systems functions: (1) they themselves have target effects; (2) they have adequately strong adsorptive special effects for anticancer drugs to make sure they can transfer the drugs to the effect-relevant locations; and (3) they can release the drugs from them in the effect-relevant locations, and only in this way can the treatment sound effects grow. The transferring abilities of CNTs combined with suitable surface modifications and their unique physicochemical properties demonstrate great potential to meet the three pre-requisites (Zhang et al. Citation2011).
CNTs are lengthy carbon-based tubes which can be either single or multiwalled and have the potential to perform as bio persistent fibers. Nanotubes for single-walled carbon nanotubes (SWCNT) and multiwalled carbon nanotubes (MWCNT) have feature ratios >100, with measurements of numerous mm and widths of 0.7–1.5 nm and 2–50 nm, respectively (Shvedova et al. Citation2003). Based on current investigation with carbon derived nanomaterials revealed that both single and multi-wall CNTs induced platelet aggregation, but not by the C60-fullerenes that are used as building blocks for these CNT (Radomski et al. Citation2005). Recently study informed that cisplatin, a small molecule, can be loaded into SWCNTs with a diameter of 1.3–1.6 nm (Tripisciano et al. Citation2009). The cisplatin incorporated into the tubes was demonstrated with, infrared spectroscopy, Raman spectroscopy and high-resolution communication electron microscopy (TEM). Drug-release study using dialysis membrane technique has shown that cisplatin was frequently released for almost a week, with maximum release during 72 h and up to one week. Wu et al. effectively created a new MWCNT based drug delivery system by tethering anticancer agent HCPT onto the surface of MWCNTs. CNTs were surface-functionalized, which was followed by amidation with a hydrophilic diaminotriethylene glycol, and subsequent conjugation of by carboxyl enhancement via heightened oxidization treatment, succinylated HCPT to hydroxyl derivatized MWCNTs was attained via a cleavable ester linkage (Wu et al. Citation2009). Of specific attention here are lipid-based nanoparticles (LNPs) that are genuine particles (about 100 nm in dimension) accumulated from diversities of lipid and other chemical modules that perform cooperatively to overcome biological barriers for LNPs in order to specially accumulate in or around disease-target cells for the functional delivery of therapeutic agents in order to cure or of imaging agents for diagnosis. The abilities of these LNPs will obviously vary depending on practical necessities, but the nanoscale permits for an impressive level of variety in abilities to permit corresponding LNPs to address a similarly varied range of functional necessities. Consequently, LNPs should be considered as suitable vehicles to make available an integrated, personalized method to cancer diagnosis and therapy in future cancer disease management. There is one aspect that is very much in favor of multifunctional LNP use in terms of diagnosis of cancer and therapy. LNPs ordered in the blood stream (i.v. administration) regularly gather in tumors anyway due to the improved penetrability and retention (EPR) effect, a performance that was recognized by Matsumura and Maeda as a means to target anticancer therapeutic agents to tumors (Matsumura and Maeda Citation1986). LNP accumulation in tumors takes place by reason of the attendance of highly penetrable blood vessels in tumors with great fenestrations (>100 nm in size), a consequence of quick, faulty angiogenesis. Furthermore, tumors are considered by dysfunctional lymphatic drainage that assistances the retention of LNPs in tumor for long sufficient to permit local nanoparticle to disintegrate in the locality of tumor cells (Thanou and Duncan Citation2003).
Micelles’ advantage, extending blood circulation, occasionally turns into a problematic that may result in extravasation of the encapsulated cargos in unexpected locations due to the little firmness of the micellar system (Peer et al. Citation2007). To address some of these problems, localized drug delivery to the solid tumors is a good approach. By comparison to the systemic administration mentioned to beyond, the localized system has several advantages such as confirming therapeutic drug levels at the tumor location for prolonged times of time while keeping low systemic drug exposure which not only consequences in advanced therapeutic effectiveness of the drugs to cancer and a lower toxicity (Ho et al. Citation2007). But also decreases the need for repeat chemotherapeutic administrations, improving the quality of life and enhancing patient compliance. The implantable bulk materials (like blocks, films, wafers and so on) and the conventional injectable hydrogel system for conventional localized drug delivery systems, are the furthermost communal methods. The degradation rate is hard to be tuned in order to the implantable bulk materials. For the conventional injectable hydrogel systems, which can improve patient compliance and ease, it can be approximately divided into two categories: particle drug depots and semisolid drug depots (Hatefi and Amsden Citation2002). The particle drug depots, including emulsions, liposomes, biodegradable microspheres and micelles, are comparatively unbalanced and easy to transfer away from the tumor location, while, for the semisolid drug depots, the solidification of liquid hydrogel in vivo is problematic sometimes, and an primary burst of drug may happen during the lag time between the injection and the formation of the solid hydrogel. Presently, the challenges of this localized drug delivery using polymers are the lack of control in drug release and distribution (Wolinsky et al. Citation2012) and specific targeting to tumor cells. Consequently, the incorporation of the active targeting micellar system into the implantable, “controllable” matrix may be a good optimal to attain a high chemotherapy effectiveness against tumors and low side effects in normal tissues and overcome the drawbacks of the conventional localized drug delivery systems.
Conclusion
In the past few years, a number of approaches have been demonstrated to obtain aligned polymer fibers. Numerous anticancer drugs include Dox, paclitaxel (PTX), platinum complexes, and dichloroacetate have been electrospun into fibers and used for postoperative local chemotherapy. For example, preparation of ultrafine Dox-containing PEG–PLLA fibers by electrospinning a water-in-oil emulsion, in which the aqueous phase contained the water-soluble drugs and the oily phase was a chloroform solution of PEG–PLLA. The consequences showed that the Dox was entirely encapsulated inside the electrospun fibers. Then; they successfully loaded hydrophobic PTX and hydrophilic Dox at the same time into PEG–PLLA nanofiber mats by the emulsion-electrospinning method, and recognized multi-drug delivery
Authors’ contributions
AA conceived of the study and participated in its design and coordination. SD, and YP participated in the sequence alignment and drafted the manuscript. All authors read and approved the fi nal manuscript.
Acknowledgements
The authors thank the Department of Medical Nanotechnology, Faculty of Advanced Medical Science of Tabriz University, for all support provided. This work is funded by the 2016 Drug Applied Research Center Tabriz University of Medical Sciences Grant.
Disclosure statement
The authors report no declarations of interest. The authors alone are responsible for the content and writing of the paper.
References
- Abbasi E, Aval SF, Akbarzadeh A, Milani M, Nasrabadi HT, Hanifepour Y, Nejati-Koshki K, Pashaei-Asl R. 2014a. Dendrimers: synthesis, applications, and properties. Nanoscale Res Lett. 9:247.
- Abbasi E, Milani M, Fekri Aval S, Kouhi M, Akbarzadeh A, Tayefi Nasrabadi H. H. 2014b. Silver nanoparticles: synthesis, properties, bio-applications and limitations. Crit Rev Microbiol. 1.
- Agudelo-Garcia PA, De Jesus JK, Williams SP, Nowicki MO, Chiocca EA, Liyanarachchi S, et al. 2011. Glioma cell migration on three-dimensional nanofiber scaffolds is regulated by substrate topography and abolished by inhibition of STAT3 signaling. Neoplasia. 13:831–840.
- Ahmadi A, Shirazi H, Pourbagher N, Akbarzadeh A, Omidfar K. 2014. An electrochemical immunosensor for digoxin using core-shell gold coated magnetic nanoparticles as labels. Mol Biol Rep. 41:1659–1668.
- Al Faraj A, Shaik AP, Shaik AS. 2015. Magnetic single-walled carbon nanotubes as efficient drug delivery nanocarriers in breast cancer murine model: noninvasivemonitoring using diffusion-weighted magnetic resonance imaging assensitive imaging biomarker. Int J Nanomed. 10:157–168.
- Alimirzalu S, Akbarzadeh A, Abbasian M, Alimohammadi S, Davaran S, Hanifehpour Y, Samiei M, Joo SW. 2014. Synthesis and study of physicochemical characteristics of Fe3O4 magnetic nanocomposites based on poly(Nisopropylacrylamide) for anti-cancer drugs delivery. Asian Pac J Cancer Prev. 15:049–054.
- Alizadeh E, Akbarzadeh A, Zarghami N, Eslaminejad MB, Hashemzadeh S, Nejati-Koshki K. 2014. Up-regulation of liver enriched transcription factors (HNF4a and HNF6) and liver specific MicroRNA (miR-122) by inhibition of Let-7b in mesenchymal stem cells. Chem Biol Drug Des. 85:600–608.
- Alizadeh E, Eslaminejad MB, Akbarzadeh A, Sadeghi Z, Abasi M, Herizchi R, Zarghami N. 2016. Upregulation of MiR-122 via trichostatin a treatments in hepatocyte-like cells derived from mesenchymal stem cells. Chem Biol Drug Des. 87:296–305. doi: 10.1111/cbdd.12664. Epub 2015 Oct 19.
- Alizadeh E, Zarghami N, Eslaminejad MB, Akbarzadeh A, Barzegar A, Mohammadi SA 2016. The effect of Dimethyl Sulfoxide (DMSO) on hepatic differentiation of mesenchymal stem cells. Artificial Cells Nanomed Biotechnol. 44:157–164.
- Allen TM, Cullis PR. 2004. Drug delivery systems: entering the mainstream. Science. 303:1818–1822.
- Ananthanarayanan B, Kim Y, Kumar S. 2011. Elucidating the mechanobiology of malignant brain tumors using a brain matrix-mimetic hyaluronic acid hydrogel platform. Biomaterials. 32:7913–7923.
- Arnold U, Ulbrich-Hofmann R. 2006. Natural and engineered ribonucleases as potential cancer therapeutics. Biotechnol Lett. 28:1615–1622.
- Arvizo RR, Saha S, Wang E, Robertson JD, Bhattacharya R, Mukherjee P. 2013. Inhibition of tumor growth and metastasis by a self-therapeutic nanoparticle. Proc Natl Acad Sci USA. 110:6700–6705.
- Bao SD, Wu QL, McLendon RE, Hao YL, Shi Q, Hjelmeland AB, et al. 2006. Glioma stem cells promote radioresistance by preferential activation of the DNA damage response. Nature. 444:756–760.
- Basarkar A, Singh J. 2009. Poly (lactide-co-glycolide)-polymethacrylate nanoparticles for intramuscular delivery of plasmid encoding interleukin-10 to prevent autoimmune diabetes in mice. Pharm Res. 26:72–81.
- Baumann R, et al. 2012. Preparation and characterization of magnetizable aerosols. Eur J Pharm Sci. 45:693–697.
- Bellail AC, Hunter SB, Brat DJ, Tan C, Van Meir EG. 2004. Microregional extracellular matrix heterogeneity in brain modulates glioma cell invasion. Int J Biochem Cell Biol. 36:1046–1069.
- Blakney AK, Ball C, Krogstad EA, Woodrow KA. 2013. Electrospun fibers for vaginal anti-HIV drug delivery. Antiviral Res. 100:S9–S16.
- Chakraborty S, Liao IC, Adler A, Leong KW. 2009. Electrohydrodynamics: a facile technique to fabricate drug delivery systems. Adv Drug Deliv Rev. 61:1043–1054.
- Charernsriwilaiwat N, et al. 2012. Lysozyme-loaded, electrospun chitosan-based nanofiber mats for wound healing. Int J Pharm. 427:379–384.
- Chaturvedi K, et al. 2011. “Cyclodextrin-based siRNA delivery nanocarriers: a state-of-the-art review”. Expert Opinion on Drug Delivery 8:1455–1468.
- Chaturvedi K, et al. 2013. “Polymeric hydrogels for oral insulin delivery”. J Control Release 165:129–138.
- Chen Z, Wang F, Zhu L. 2013. The effects of hypoxia on uptake of positively chargednanoparticles by tumor cells. J. Bionanosci. 7:601–605.
- Chew SY, Mi R, Hoke A, Leong KW. 2008. The effect of the alignment of electrospun fibrous scaffolds on Schwann cell maturation. Biomaterials. 29:653–661.
- Christopherson GT, Song H, Mao HQ. 2009. The influence of fiber diameter of electrospun substrates on neural stem cell differentiation and proliferation. Biomaterials. 30:556–564.
- Conde J, et al. 2013. In vivo tumor targeting via nanoparticle-mediated therapeutic siRNA coupled to inflammatory response in lung cancer mouse models. Biomaterials. 34:7744–7753.
- Coquerel B, Poyer F, Torossian F, Dulong V, Bellon G, Dubus I, et al. 2009. Elastinderived peptides: matrikines critical for glioblastoma cell aggressiveness in a 3-D system. Glia. 57:1716–1726.
- Corey JM, Gertz CC, Wang BS, Birrell LK, Johnson SL, Martin DC, et al. 2008. The design of electrospun PLLA nanofiber scaffolds compatible with serum-free growth of primary motor and sensory neurons. Acta Biomater. 4:863–875.
- Couvreur P, Vauthier C. 2006. Nanotechnology: intelligent design to treat complex disease. Pharm Res. 23:1417–1450.
- Dahlin RL, Kurtis Kasper F, Mikos AG. 2011. Polymeric nanofibers in tissue engineering. Tissue Eng Part B Rev. 17:349–364.
- Daraee H, Etemadi A, Kouhi M, Alimirzalu S, Akbarzadeh A. 2016. Application of liposomes in medicine and drug delivery. Artif Cells Nanomed Biotechnol. 44:381–391.
- David L, Dulong V, Coquerel B, Le Cerf D, Cazin L, Lamacz M, et al. 2008. Collagens, stromal cell-derived factor-1alpha and basic fibroblast growth factor increase cancer cell invasiveness in a hyaluronan hydrogel. Cell Prolif. 41:348–364.
- Davoudi Z, Akbarzadeh A, Rahmatiyamchi M, Movassaghpour AA, Alipour M, Nejati-Koshki K, et al. 2014. Molecular target therapy of AKT and NF-kB signaling pathways and multidrug resistance by specific cell penetrating inhibitor peptides in HL-60 cells. Asian Pac J Cancer Prev. 15:4353.
- Díaz MR, Vivas-Mejia PE. 2013. Nanoparticles as drug delivery systems in cancer medicine: emphasis on RNAi-containing nanoliposomes. Pharmaceuticals. 6:1361–1380.
- Eatemadi A, Daraee H, Karimkhanloo H, Kouhi M, Zarghami N, Akbarzadeh A, et al. 2014. Carbon nanotubes: properties, synthesis, purification, and medical applications. Nanoscale Res Lett. 9:1–13.
- Eatemadi A, Daraee H, Zarghami N, Yar HM, Akbarzadeh A. 2016. Nanofiber: synthesis and biomedical applications. Artif Cells Nanomed Biotechnol. 44:111–121.
- Ebrahimnezhad Z, Zarghami N, Keyhani M, Amirsaadat S, Akbarzadeh A, Rahmati M, Taheri ZM, Nejati-Koshki K. 2013. Inhibition of hTERT gene expression by silibinin-loaded PLGA-PEG-Fe3O4 in T47D breast cancer cell line. BioImpacts. 3:67–74.
- Elham A, Abolfazl A, Mohammad K, Morteza M. 2014. Graphene: synthesis, bio-applications, and properties. Artif Cells Nanomed Biotechnol. 1–7.
- Eommolbanin E, Elham A, Abolfazl A, Amir Ahmad K, Soodabeh D. 2014. Novel drug delivery system based on doxorubicin-encapsulated magnetic nanoparticles modified with PLGA-PEG1000 copolymer. Artif Cells Nanomed Biotechnol. 1–8.
- Farokhzad OC, Langer R. 2009. Impact of nanotechnology on drug delivery. ACS Nano. 3:16–20.
- Fekri Aval S, Akbarzadeh A, Yamchi MR, Zarghami F, Nejati-Koshki K, Zarghami N 2016. Gene silencing effect of SiRNA-magnetic modified with biodegradable copolymer nanoparticles on hTERT gene expression in lung cancer cell line. Artif Cells Nanomed Biotechnol. 44:188–193.
- Ferrari M. 2005. Cancer nanotechnology: opportunities and challenges. Nat Rev Cancer. 5:161–171.
- Fox ME, Szoka FC, Fréchet JMJ. 2009. Soluble polymer carriers for the treatment of cancer: the importance of molecular architecture. Accounts Chem Res. 42:1141–1151.
- Gao H, Yang Z, Zhang S, Cao S, Shen S, Pang Z, et al. 2013. Ligand modified nanoparticles increases cell uptake, alters endocytosis and elevates glioma distribution and internalization. Sci Rep. 3:2534.
- Gerardo-Nava J, Fuhrmann T, Klinkhammer K, Seiler N, Mey J, Klee D, et al. 2009. Human neural cell interactions with orientated electrospun nanofibers in vitro. Nanomedicine (Lond). 4:11–30.
- Ghasemali S, Nejati-Koshki K, Akbarzadeh A, Tafsiri E, Zarghami N, Rahmati-Yamchi M, et al. 2013. Study of inhibitory effect of β-cyclodextrin-Helenalin complex on HTERT gene expression in T47D breast cancer cell line by real time quantitative PCR (q-PCR). Asian Pac J Cancer Prev. 14:6949–6953.
- Giese A, Loo MA, Rief MD, Tran N, Berens ME. 1995. Substrates for astrocytoma invasion. Neurosurgery. 37:294–301.
- Goonoo N, Bhaw-Luximon A, Jhurry D. 2014. Drug loading and release from electrospun biodegradable nanofibers. J Biomed Nanotechnol. 10:2173–2199.
- Daraee H, Eatemadi A, Abbasi E, Fekri Aval S, Kouhi M, Akbarzadeh A. 2016. Application of gold nanoparticles in biomedical and drug delivery. Artif Cells Nanomed Biotechnol. 44:410–422.
- Hatefi A, Amsden B. 2002. Biodegradable injectable in situ forming drug delivery systems. J Control Release. 80:9–28.
- He W, et al. 2014. Development of single-side magnet array for super paramagnetic nano-particle targeting. Res J Appl Sci Eng Technol. 7:3022–3029.
- Ho EA, Soo PL, Allen C, Piquette-Miller M. 2007. Impact of intraperitoneal, sustained delivery of paclitaxel on the expression of P-glycoprotein in ovarian tumors. J Control Release. 117:20–27.
- Hosseininasab S, Pashaei‐Asl R, Khandaghi AA, Nasrabadi HT, Nejati‐Koshki K, Akbarzadeh A, et al. 2014. Synthesis, characterization, and In vitro studies of PLGA‐PEG nanoparticles for oral Insulin delivery. Chem Biol Drug Des. 84:307–315.
- Hournkumnuard K, Natenapit M. 2013. Magnetic drug targeting by ferromagnetic microwires implanted within blood vessels. Med Phys. 40: 062302/1-062302/10.
- Huang ZM, Zhang YZ, Kotaki M, Ramakrishna S. 2003. A review on polymer nanofibers by electrospinning and their applications in nanocomposites. Compos Sci Technol. 63:2223–2253.
- Jemal A, Siegel R, Ward E. 2006. i wsp.: Statystyka nowotworów 2006 Onkol Dypl. 3:6–35.
- Chung JH, Kim YK, Kim KH, Kwon TY, Vaezmomeni SZ, Samiei M, et al. 2016. Synthesis, characterization, biocompatibility of hydroxyapatite -natural polymers nanocomposites for dentistry applications. Artif Cells Nanomed Biotechnol. 44:277–284.
- Johnson J, Nowicki MO, Lee CH, Chiocca EA, Viapiano MS, Lawler SE, et al. 2009. Quantitative analysis of complex glioma cell migration on electrospun polycaprolactone using time-lapse microscopy. Tissue Eng Part C Methods. 15:531–540.
- Joshi M, Butola BS, Saha K. 2014. Advances in topical drug delivery system: micro to nanofibrous structures. J Nanosci Nanotechnol. 14:853–867.
- Jovanov-Milosevic N, Benjak V, Kostovic I. 2006. Transient cellular structures in developing corpus callosum of the human brain. Coll Antropol. 30:375–381.
- Kawashima T, et al. 2011. “A scalable controlled-release device for transscleral drug delivery to the retina”. Biomaterials 32:1950–1956.
- Kazunori K, et al. 1993. Block copolymer micelles as vehicles for drug delivery. J Control Release. 24:119–132.
- Kim JH, Kim JH, Park JA, Lee SW, Kim WJ, Yu YS, et al. 2006. Blood-neural barrier: intercellular communication at glio-vascular interface. J Biochem Mol Biol. 39:339–345.
- Kim Y‐J,Ebara M, Aoyagi T. 2013. A smart hyperthermia nanofiber with switchable drug release for inducing cancer apoptosis. Adv Funct Mater. 23:5753–5761.
- Kouhi M, Vahedi A, Akbarzadeh A, Hanifehpour Y, Joo SW. 2014. Investigation of quadratic electro-optic effects and electro absorption process in GaN/AlGaN spherical quantum dot. Nanoscale Res Lett. 9:131–136.
- Kuang Y, An S, Guo Y, Huang S, Shao K, Liu Y, et al. 2013. T7 peptide-functionalized nanoparticles utilizing RNA interference for glioma dual targeting . Int J Pharm. 454:11–20.
- Kudgus RA, Szabolcs A, Khan JA, Walden CA, Reid JM, Robertson JD, et al. 2013. Inhibiting the growth of pancreatic adenocarcinoma in vitro and in vivo through targeted treatment with designer gold nanotherapeutics. PLoS One. 8:e57522.
- Langer R. 1998. Drug delivery and targeting. Nature. 392:5–10.
- Lei C, Cui Y, Zheng L, Chow PK, Wang CH. 2013. Development of a gene/drug dual delivery system for brain tumor therapy: potent inhibition via RNA interference and synergistic effects. Biomaterials. 34:7483–7494.
- Letchford K, Burt H. 2007. A review of the formation and classification of amphiphilic block copolymer nanoparticulate structures: micelles, nanospheres, nanocapsules and polymersomes. Eur J Pharm Biopharm. 65:259–269.
- Lin X, Li Y, Chen Z, Zhang C, Luo X, Du X, Huang Y. 2013. Synthesis, characterization and electrospinning of new thermoplastic carboxymethyl cellulose (TCMC). Chem Eng J. 215:709–720.
- Liu J, Huang Y, Kumar A, Tan A, Jin S, Mozhi A, Liang XJ. 2014. pH-Sensitive nano-systems for drug delivery in cancer therapy. Biotechnol Adv. 32:693–710.
- Liu J, Zhou G, Liu D, Xie Z, Huang Y, Wang X, Wu W, Jing X. 2013. Inhibition of orthotopic secondary hepatic carcinoma in mice by doxorubicin-loaded electrospun polylactide nanofibers. J Mater Chem. 1:101–109.
- Liu S, Zhou G, Liu D, Xie Z, Huang Y, Wang X, Wu W, Jing X. 2015. Single peptide ligand-functionalized uniform hollow mesoporoussilica nanoparticles achieving dual-targeting drug delivery to tumor cells and angiogenic blood vessel cells. Int. J. Nanomed. 1855–1867.
- Louis DN. 2006. Molecular pathology of malignant gliomas. Annu Rev Pathol. 1:97–117.
- Low PS, Kularatne SA. 2009. Folate-targeted therapeutic and imaging agents for cancer. Curr Opinion Chem Biol. 13:256–262.
- Lueshen E, et al. 2015. Implant-assisted intrathecal magnetic drug targeting to aid in therapeutic nanoparticle localization for potential treatment of central nervous system disorders. J Biomed Nanotechnol. 11:253–261.
- Ma PX, Zhang R. 1999. Synthetic nano-scale fibrous extracellular matrix. J Biomed Mater Res. 46:60–72.
- Machado RM, Palmeira-De-Oliveira A, Martinez-De-Oliveira J, Palmeira-De-Oliveira R. 2013. Vaginal films for drug delivery. J Pharm Sci. 102:2069–2081.
- Madan M, Bajaj A, Lewis S, Udupa N, Baig JA. 2009. In situ forming polymeric drug delivery systems. Ind J Pharm Sci. 71:242.
- Malcolm RK, Edwards KL, Kiser P, Romano J, Smith TJ. 2010. Advances in microbicide vaginal rings. Antiviral Res. 88:S30–S39.
- Matsumura Y, Maeda H. 1986. A new concept for macromolecular therapeutics in cancer chemotherapy: mechanism of tumoritropic accumulation of proteins and the antitumor agent smancs. Cancer Res. 46: 6387–6392.
- Minchinton AI, Tannock IF. 2006. Drug penetration in solid tumours. Nat Rev Cancer. 6:583–592.
- Mohammad P-M, Nosratollah Z, Afshin M, Mohammad R-Y, Davud G, Abolfazl A, Miguel de l. G, Kazem N-K. 2014. Watercress-based gold nanoparticles: biosynthesis, mechanism of formation and study of their biocompatibility in vitro. IET Digital Library. 4:5.
- Mollazade M, Nejati-Koshki K, Akbarzadeh A, Hanifehpour Y, Zarghami N, Joo SW. 2013. PAMAM dendrimers arugment inhibitory effect of curcumin on cancer cell proliferation: possible inhibition of telomerase. Asian Pac J Cancer Prev. 14:6925–6928.
- Mora-Huertas CE, Fessi H, Elaissari A. 2010. Polymer-based nanocapsules for drug delivery. Int J Pharm. 385:113–142.
- Moses MA, Brem H, Langer R. 2003. Advancing the field of drug delivery: taking aim at cancer. Cancer Cell. 4:337–341.
- Muthu MS, Leong DT, Mei L, Feng SS. 2014. Nanotheranostics – application and further development of nanomedicine strategies for advanced theranostics. Theranostics. 4:660–677.
- Nacev A, et al. 2015. Dynamic inversion enables external magnets to concentrate ferromagnetic rods to a central target. Nano Lett. 15:359–364.
- Nasrabadi HT, Abbasi E, Davaran S, Kouhi M, Akbarzadeh A. 2016. Bimetallic nanoparticles: preparation, properties, and biomedical applications. Artif Cells Nanomed Biotechnol. 44:376–380.
- Nejati-Koshki K, Akbarzadeh A, pourhasan-Moghadam M, joo S. w. 2013. Inhibition of leptin and leptin receptor gene expression by silibinin. Curcumin combination. Asian Pac J Cancer Prev. 14:6595–6599.
- Neves V, Gerondopoulos A, Heister E, Tîlmaciu C, Flahaut E, Soula B, Silva SR, McFadden J, Coley HM. 2012. Cellular localization, accumulation and trafficking of double-walled carbon nanotubes in human prostate cancer cells. Nano Res. 5:223–234.
- Newland B, Zheng Y, Jin Y, Abu-Rub M, Cao H, Wang W, Pandit A. 2012. Single cyclized molecule versus single branched molecule: a simple and efficient 3D “knot” polymer structure for nonviral gene delivery. J Am Chem Soc. 134:4782–4789.
- Nicolas J, et al. 2013. Design, functionalization strategies and biomedical applications of targeted biodegradable/biocompatible polymer-based nanocarriers for drug delivery. Chem Soc Rev. 42:1147–1235.
- Panyam J, Labhasetwar V. 2003. Biodegradable nanoparticles for drug and gene delivery to cells and tissue. Adv Drug Deliv Rev. 55:329–347.
- Peer D, Karp JM, Hong S, Farokhzad OC, Margalit R, Langer R. 2007. Nanocarriers as an emerging platform for cancer therapy. Nat Nanotechnol. 2:751–760.
- Peer D, Karp JM, Hong S, FaroKhzad OC, Margalit R, Langer R. 2007. Nanocarriers as an emerging platform for cancer therapy. Nat Nanotechnol. 2:751–760.
- Peter M, Binulal NS, Nair SV, Selvamurugan N, Tamura H, Jayakumar R. 2010. Novel biodegradable chitosan–gelatin/nano-bioactive glass ceramic composite scaffolds for alveolar bone tissue engineering. Chem Eng J. 158:353–361.
- Piccolo J, Kolesar JM. 2014. “Prevention and treatment of chemotherapy-induced peripheral neuropathy”. Am J Health-Syst Pharm. 71:19–25.
- Postma TJ, Reijneveld JC, Heimans JJ. 2013. Prevention of chemotherapy-induced peripheral neuropathy: a matter of personalized treatment? Ann Oncol. 24:1424–1426.
- Pourhassan-Moghaddam M, Rahmati-Yamchi M, Akbarzadeh A, Daraee H, Nejati-Koshki K, Hanifehpour Y, Joo SW. 2013. Protein detection through different platforms of immuno-loop-mediated isothermal amplification. Nanoscale Res Lett. 8:485.
- Prabhakaran MP, Venugopal JR, Ramakrishna S. 2009. Mesenchymal stem cell differentiation to neuronal cells on electrospun nanofibrous substrates for nerve tissue engineering. Biomaterials. 30:4996–5003.
- Radomski A, Jurasz P, Alonso-Escolano D, et al. 2005. Nanoparticle-induced platelet aggregation and vascular thrombosis. Br J Pharmacol. 146:882–893.
- Ranganath SH, Fu Y, Arifin DY, Kee I, Zheng L, Lee HS, Chow PK, Wang CH. 2010. The use of submicron/nanoscale PLGA implants to deliver paclitaxel with enhanced pharmacokinetics and therapeutic efficacy in intracranial glioblastoma in mice. Biomaterials. 31:5199–5207.
- Ranganath SH, Wang C-H. 2008. Biodegradable microfiber implants delivering paclitaxel for post-surgical chemotherapy against malignant glioma. Biomaterials. 29:2996–3003.
- Rao SS, Dejesus J, Sarkar A, Winter JO. Brain mimetic hydrogels for investigating migration of glioblastoma multiformes in 3D. In: Transactions of the 35th Annual Meeting of Society for Biomaterials. XXXIII; 2011. p. 671.
- Rao SS, Nelson MT, Xue R, DeJesus JK, Viapiano MS, Lannutti JJ, Sarkar A, Winter JO. 2013. Mimicking white matter tract topography using core-shell electrospun nanofibers to examine migration of malignant brain tumors. Biomaterials. 34:5181–5190.
- Rudzinski WE, Aminabhavi TM. 2010. “Chitosan as a carrier for targeted delivery of small interfering RNA”. International Journal of Pharm. 399:1–11.
- Sasiadek W. 2014. Predicting radiotherapy-related clinical toxicities in cancer: a literature review. Clin J Oncol Nurs. 18:E37–E44.
- Saravanabhavan SS, Dharmalingam S. 2013. Fabrication of polysulphone/hydroxyapatite nanofiber composite implant and evaluation of their in vitro bioactivity and biocompatibility towards the post-surgical therapy of gastric cancer. Chem Eng J. 234:380–388.
- Sarkar A, Chiocca EA. Glioblastoma and malignant astrocytoma. In: Laws Ka, editor. Brain Tumors: An Encyclopedic Approach, 3rd ed. Edinburgh, New York, USA: Churchill Livingstone; 2011.
- Schnell E, Klinkhammer K, Balzer S, Brook G, Klee D, Dalton P, et al. 2007. Guidance of glial cell migration and axonal growth on electrospun nanofibers of polyepsilon caprolactone and a collagen/poly-epsilon-caprolactone blend. Biomaterials. 28:3012–3025.
- Shevtsov MA, et al. 2014. Tumor targeting using magnetic nanoparticle Hsp70 conjugate in a model of C6 glioma. Neuro-oncology 16:38–49.
- Shvedova AA, Castranova V, Kisin ER, et al. 2003. Exposure to carbon nanotube material: assessment of nanotube cytotoxicity using human keratinocyte cells. J Toxicol Environm Health Part A. 66:1909–1926.
- Sill TJ, von Recum HA. 2008. Electrospinning: applications in drug delivery and tissue engineering. Biomaterials. 29:1989–2006.
- Sokolsky-Papkov M, Agashi K, Olaye A, Shakesheff K, Domb AJ. 2007. Polymer carriers for drug delivery in tissue engineering. Adv Drug Deliv Rev. 59:187–206.
- Szentivanyi A, Chakradeo T, Zernetsch H, Glasmacher B. 2011. Electrospun cellular microenvironments: understanding controlled release and scaffold structure. Adv Drug Deliv Rev. 63:209–220.
- Tabatabaei Mirakabad FS, Akbarzadeh A, Milani M, Zarghami N, Taheri-Anganeh M, Zeighamian V, Badrzadeh F, Rahmati-Yamchi M. 2016. A Comparison between the cytotoxic effects of pure curcumin and curcumin-loaded PLGA-PEG nanoparticles on the MCF-7 human breast cancer cell line. Artif Cells Nanomed Biotechnol. 44:423–430.
- Taherkhani S, et al. 2014. Covalent binding of nanoliposomes to the surface of magnetotactic bacteria for the synthesis of self-propelled therapeutic agents. ACS Nano. 8:5049–5060.
- Thanou M, Duncan R. 2003. Polymer-protein and polymer-drug conjugates in cancer therapy. Curr Opinion Investigat Drugs. 4:701–709.
- Tietze R, et al. 2013. Efficient drug-delivery using magnetic nanoparticles-biodistribution and therapeutic effects in tumour bearing rabbits. Nanomedicine. 9:961–971.
- Tietze R, Zaloga J, Unterweger H, Lyer S, Friedrich RP, Janko C, Pöttler M, Dürr S, Alexiou C. 2015. Magnetic nanoparticle-based drug delivery for cancer therapy. Biochem Biophys Res Commun. 468:463–470.
- Tripisciano C, Kraemer K, Taylor A, Borowiak-Palen E. 2009. Single-wall carbon nanotubes based anticancer drug delivery system. Chem Phys Lett. 478:200–205.
- Tseng YY, Kao YC, Liao JY, Chen WA, Liu SJ. 2013. Biodegradable drug-eluting poly[lactic-co-glycol acid] nanofibers for the sustainable delivery of vancomycin to brain tissue: in vitro and in vivo studies ACS Chem Neurosci. 4:1314–1321.
- Valster A, Tran NL, Nakada M, Berens ME, Chan AY, Symons M. 2005. Cell migration and invasion assays. Methods. 37:208–215.
- Wang C,Wang Z, Zhang X. 2012. Amphiphilic building blocks for self-assembly: from amphiphiles to supra-amphiphiles. Acc Chem Res. 45:608–618.
- Wang HB, Mullins ME, Cregg JM, Hurtado A, Oudega M, Trombley MT, et al. 2009. Creation of highly aligned electrospun poly-L-lactic acid fibers for nerve regeneration applications. J Neural Eng. 6:016001.
- Wang J, Yang G, Guo X, Tang Z, Zhong Z, Zhou S. 2014. Redox-responsive polyanhydride micelles for cancer therapy. Biomaterials. 35:3080–3090.
- Wen PY, Kesari S. 2008. Malignant gliomas in adults. N Engl J Med. 359:492–507.
- Wolinsky JB, Colson YL, Grinstaff MW. 2012. Local drug delivery strategies for cancer treatment: gels, nanoparticles, polymeric films, rods, and wafers. J Control Release. 159:14–26.
- Wu W, Li R, Bian X, Zhu Z, Ding D, Li X, et al. 2009. Covalently combining carbon nanotubes with anticancer agent: preparation and antitumor activity. ACS Nano. 9:2740–2750.
- Xiao D, et al. 2014. A dual-responsive mesoporous silica nanoparticle for tumor-triggered targeting drug delivery. Small. 10:591–598.
- Yang G, et al. 2015. An implantable active-targeting micelle-in-nanofiber device for efficient and safe cancer therapy. ACS Nano. 9:1161–1174.
- Yang YL, Sun C, Wilhelm ME, Fox LJ, Zhu JL, Kaufman LJ. 2011. Influence of chondroitin sulfate and hyaluronic acid on structure, mechanical properties, and glioma invasion of collagen I gels. Biomaterials. 32:7932–7940.
- Zamani M, Prabhakaran MP, Ramakrishna S. 2013. Advances in drug delivery via electrospun and electrosprayed nanomaterials. Int J Nanomed. 8:2997.
- Zeng J, Xu X, Chen X, Liang Q, Bian X, Yang L, Jing X. 2003. Biodegradable electrospun fibers for drug delivery. J Control Release. 92:227–231.
- Zeng J, Yang L, Liang Q, Zhang X, Guan H, Xu X, et al. 2005. Influence of the drug compatibility with polymer solution on the release kinetics of electrospun fiber formulation. J Control Release. 105:43–51.
- Zhang B, Sun X, Mei H, Wang Y, Liao Z, Chen J, et al. 2013. LDLR-mediated peptide-22-conjugated nanoparticles for dual-targeting therapy of brain glioma. Biomaterials. 34:9171–9182.
- Zhang W, Zhang Z, Zhang Y. 2011. The application of carbon nanotubes in target drug delivery systems for cancer therapies. Nanoscale Res Lett. 6:555.
- Zhang Y, et al. 2014. Prevention of local liver cancer recurrence after surgery using multilayered cisplatin-loaded polylactide electrospun nanofibers. Chinese J Polym Sci. 32:1111–1118.
- Zhang Z, et al. 2014. Magnetic resonance imaging-visible and pH-sensitive polymeric micelles for tumor targeted drug delivery. J Biomed Nanotechnol. 10:216–226.
- Zheng F, Wang S, Wen S, Shen M, Shi X. 2013a. Amoxicillin-loaded electrospun nano-hydroxyapatite/poly (lactic-co-glycolic acid) composite nanofibers: Preparation, characterization and antibacterial activity. J Control Release. 1:e30–31.
- Zheng F, Wang S, Wen S, Shen M, Zhu M, Shi X. 2013b. Characterization and antibacterial activity of amoxicillin-loaded electrospun nano-hydroxyapatite/poly (lactic-co-glycolic acid) composite nanofibers. Biomaterials. 34:1402–1412.
- Zong S, Wang X, Yang Y, Wu W, Li H, Ma Y, et al. 2015. The use of cisplatin-loaded mucoadhesive nanofibers for local chemotherapy of cervical cancers in mice. Eur J Pharm Biopharm. 93:127–135.