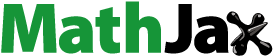
Abstract
The study was aimed at investigating the synergistic inhibitory effect of unique combinational regimen of nanocapsulated Metformin (Met) and Curcumin (Cur) against T47D breast cancer cells. For this purpose, Met and Cur were co-encapsulated in PEGylated PLGA nanoparticles (NPs) and evaluated for their therapeutic efficacy. The morphology and dynamic light scattering (DLS) analyses were carried out to optimize the nanoformulations. Drug release study was performed using dialysis method and then the cytotoxic and inhibitory effect of individual and combined drugs on expression level of hTERT in T47D breast cell line were evaluated using MTT assay and qPCR, respectively. The results showed that free drugs and formulations exhibited a dose-dependent cytotoxicity against T47D cells and especially, Met–Cur–PLGA/PEG NPs had more synergistic antiproliferative effect and significantly arrested the growth of cancer cells than the other groups (p < .05). Real-time PCR results revealed that Cur, Met and combination of Met–Cur in free and encapsulated forms inhibited hTERT gene expression. It was found that Met–Cur–PLGA/PEG NPs in relative to free combination could further decline hTERT expression in all concentration (p < .05). Taken together, our study demonstrated that Met–Cur–PLGA/PEG NPs based combinational therapy holds promising potential towards the treatment of breast cancer.
Introduction
Breast cancer is now the most common cancer among women [Citation1]. Even though several chemotherapeutics such as doxorubicin, etoposide and paclitaxel have been developed to treat this type of cancer, issues remain such as high reoccurrence, low survival rates and a significant decrease in quality of life after conventional chemotherapy and radiation treatment [Citation2]. Accordingly, development of efficient anti-cancer drugs with minimal side effects is necessary and substances such as Curcumin (Cur) and Metformin (Met) are promising products for such therapeutic applications [Citation3,Citation4].
Telomeres are present at end of all eukaryotic chromosomes and play significant functions in genome stability and human aging [Citation2]. Telomerase is a specialized DNA–protein structure responsible for preserving the integrity and length of chromosome ends [Citation5]. Telomerase enzyme from all species minimally consists of two crucial constituents: a catalytic subunit human telomerase reverse transcriptase (hTERT) and a human telomerase RNA component (hTER), which serves as the template for adding of new telomeric repeats to the ends of replicated chromosomes [Citation6]. hTERT is the target for telomerase regulation in humans. Considerably increased hTERT expression level and activity has been detected in almost 90% of cancer cells, proposing its potential as an anticancer drug target [Citation7].
More recent human cancer therapies are based on an efficient combination of different therapeutic agents with different mode of actions. A combination strategy may improve the anti-cancer effects or even leads to a synergic inhibitory effect on a causal and contributing factor in carcinogenesis [Citation8]. Also, the shutdown of a single target occasionally does not certainly eliminate cancer and therefore utilizing a mix of molecular-targeted drugs targeting separate features or pathways has been suggested. This approach, particularly in the removal of hindrances such as tumour resistance and low drug efficiency, can be beneficial to improve the overall therapeutic efficiency of the single drug [Citation9,Citation10].
At present, approaches toward more efficient combinational therapies including accurate and controlled drug delivery are planning and employing [Citation9]. Although the combinational therapy in cancer requires to be more examined, applying inhibitors from chemotherapeutic agents and phytochemicals or applying a combination of various natural substances for cancer therapy has been accompanied with meaningful successes [Citation11,Citation12]. Telomerase, due to its structure and function, can be a favourable candidate for targeting by concurrent and combinational therapy of anti-cancer drugs together to make different inhibitory mechanisms on cancer growth [Citation13].
In spite of proven effectiveness of Met and Cur in cancer treatment, there are major challenges limiting their clinical utilizes. Met is a moderately safe drug, though given in high doses and proper formulating it may improve chemotherapy by decreasing adverse effects and improving patient compliance [Citation14,Citation15]. However, Met is highly soluble and lowly permeable, making its controlled release formulation a possible challenge. Cur has unfavourable properties such as poor solubility in water, low physicochemical stability, rapid intestinal and hepatic metabolism, genotoxicity and low cellular uptake which can limit the therapeutic applications of its beneficial effects [Citation16,Citation17]. To mitigate these hurdles, use of nanotechnological approaches that allow slow, sustained, and controlled release of the encapsulated agents can be a promising approach to improve delivery compound to cancer cells with helping in enhanced bioavailability and reduced degradation rate in vivo [Citation18–20].
In the present study, we co-encapsulated Cur and Met in PEGylated PLGA nanoparticles (NPs) and evaluated their anticancer effect against human breast cancer cell line T47D. This is the first study to depict the therapeutic efficacy of unique combination of Cur and Met.
Materials and materials
Materials
T47D cell line (breast cancer epithelial like cell line) was provided from Cell Bank of Pasteur Institute of Iran (code: C203). RPMI1640 and Fetal Bovine Serum (FBS) were purchased from Gibco, Invitrogen, UK. Penicillin G, Streptomycin, DL-Lactide, glycolide, Polyethylene glycol (PEG, molecular weight: 4000) Stannous octoate [Sn(Oct)2], polyvinyl alcohol (PVA), Dichloromethane (DCM), MTT (3,4,5-dimethylthiazol-2-yl)-2,5-diphenyltetrazolium bromide) and dimethyl sulphoxide were supplied by Sigma-Aldrich (St Louis, MO, USA). First Strand cDNA synthesis Kit and SYBR Green PCR Master Mix were purchased from Fermentas (Vilnius, Lithuania).
Synthesis of PLGA–PEG
Synthesis of PLGA/PEG was done via ring open polymerization of glycolide and DL-lactide followed by addition of PEG4000. As mentioned in melt polymerization protocol [Citation3], PEG4000 and PLGA were copolymerized in presence of Sn(Oct)2 as the catalyst. DL-lactide (2.882 g), PEG4000 (1.54 g) and glycolide (0.570 g) were melted in bottleneck flask in 140 °C under a nitrogen atmosphere. Reaction mixture comprising a 3:1 proportion of d,l-lactide to glycolide and 0.05% (w/w) Sn(Oct)2 was produced and heated to 175 °C and maintained for 4 h.
Preparation of NPs
Met-NPs were prepared by the double emulsion (W/O/W) method with a minor modification. Briefly, 20 mg of PLGA/PEG dissolved in 5 ml of DCM and 0.2 ml of Met solution were transferred to a centrifuge tube, and the mixture was emulsified using sonication for 5 min. Subsequently, the emulsion and 2 ml of 1% polyvinyl alcohol (PVA) were emulsified using sonication for 5 min. The emulsion was then gently dropped into 10 ml of 0.5% PVA and stirred for 15 min at room temperature. After vacuum evaporation of the solvent, the NPs were collected by centrifugation at 14,000 rpm for 10 min at room temperature and washed twice using distilled water.
Met–Cur NPs were produced by the improved W/O/W method. After the first emulsification, the mix and 2 ml of 1% PVA were stirred for 3 min at room temperature. At the same time, 0.2 ml of Cur-dissolved DCM was added gently, and then emulsified again. The following stages were similar with the preparation of Met NPs.
Cur NPs were prepared using an emulsion/solvent evaporation method. 20 mg of copolymer and 1 mg of Cur were dissolved in 5 ml of DCM. The solution was stirred for 15 min at room temperature and emulsified using sonication in 10 ml of aqueous solution with 1% PVA. After vacuum evaporation of the solvent, the NPs were collected by centrifugation at 14,000 rpm for 10 min at room temperature and washed twice using distilled water.
Characterization of the NPs
Measurements of particle size distribution and zeta potential
The NPs size (diameter, nm), polydispersity index and surface charge (zeta potential, mV) were determined using a Dynamic Light Scattering (DLS) Zetasizer Nano ZS (Malvern Instruments Ltd., Malvern, UK) equipped with a helium–neon laser beam at a wavelength of 633 nm and a fixed scattering angle of 90°. NPs were prepared by directly dissolving copolymers or drug-loaded NPs in distilled water at a concentration of 0.5 mg/ml followed using 10 min sonication at room temperature. Results were means ± SD of triplicates.
NPs morphology
Field emission scanning electron microscopy (FE-SEM) system (Hitachi S-4800 FE-SEM) was applied to determine the shape and surface morphology of prepared NPs. NPs were coated with gold under vacuum before SEM.
FTIR spectroscopy
A Perkin-Elmer Spectrum One model FT-IR was applied to record the IR spectra of free drugs, free copolymer (PLGA/PEG) Met NPs, Cur NPs and Met–Cur NPs prepared in KBr disks in the region of 4000–400 cm−1.
Drug loading
The drug loading efficiency (DLE) and drug loading capacity (DLC) were determined using HPLC technique. The drug nanoformulations were filtered applying Amicon centrifugal tube (molecular weight cut-off 3000 Da, Millipore, USA). The filtrate was collected to determine the quantity of the free drug (unentrapped) remains of the total amount of drug added. Few microliter of filtrate was introduced into the HPLC column. In advance, separate calibration curve has been made for Cur and Met. The calibration equations were applied to determine the quantity of drug in the filtrate:
In vitro drug release
For in vitro drugs release assay, drug-loaded NPs (20–30 mg) were dispersed in PBS (5 ml, pH = 7.4) and transferred to dialysis membrane tubing (MW cut-off: 3000) placed in 20 ml of PBS with stirring at 120 rpm at 37 °C. At selected time intervals, the environmental buffer solution was replaced with fresh PBS, and the concentration of the released Met and Cur in the removed PBS was calculated applying a calibration curve at the wavelength where Met and Cur showed their maximum absorbance (237 and 427 nm, respectively), quantitatively monitored using Lambda 950 Visible-UV spectrophotometer (PerkineElmer Fremont, CA, USA). Subsequently, the accumulative ratios of the released Met and Cur were determined as a function of time.
Cytotoxicity assay
The cytotoxicity assay was determined by 3-(4,5-dimethythiazol-2-yl)-2,5-diphenyl tetrazolium bromide (MTT) assay. It is based on the capacity of mitochondrial succinate dehydrogenase in living cells to reduce the yellow water-soluble tetrazolium salt into an insoluble, coloured formazan complex which is measured spectrophotometrically. Briefly, T47D breast cancer cells were seeded into 96-well plates (2 × 104 cells/well) and allowed to attach for 24 h before treatment. After 24 h, cells were exposed to blank copolymer, free Met, free Cur, Met–Cur, Met NPs, Cur NPs and Met–Cur NPs at different dosing level. The cells were incubated for 72 h accordingly. After 72 h exposure time, the cell culture medium was replaced and treated with 200 μl of MTT solution (2 mg/ml) and incubated for 4 h. The formed formazan crystals were extracted by adding 200 μl of pure DMSO and incubated for additional 20 min. The absorbance was record at 570 nm using an EL× 800 microplate absorbance reader (Bio Tek Instruments, Winooski, VT) with a reference wavelength of 630 nm. All experiments were repeated three times.
Real-time PCR
T47D cells were treated with different concentrations of free and drug loaded NPs for 72 h. After drug exposure time, total RNA was extracted using the Trizol reagent (Invitrogen, Carlsbad, CA) according to manufacturer’s instructions. Nanodrop was proved the purity and quantity of total RNA. After the integrity of total RNA was examined by using electrophoresis, complementary DNA (cDNA) was synthesis by tow-step RT-PCR kit according to the instructions of the manufacturer. Next, Hot Taq EvaGreen qPCR Mix was used following the instructions of the manufacturer to determine hTERT expression levels by quantitative real-time PCR technique. Sequence of forward and reverse primers for hTERT were, F: 5′-GGAGCAAGTTGCAAAGCATTG-3′, R: TCCCACGACGTAGTCCATGTT-3′ and primers for β-actin, F: 5′-TCCCTGGAGAAGAGCTACG-3′, R: 5′-GTAGTTTCGTGGATGCCACA-3′. Relative hTERT expression levels were normalized by housekeeping gene (β-actin) and relative expression of hTERT was calculated by this formula: Normalized relative ratio =2 − ΔΔCt.
Results and discussion
Preparation of met and cur loaded PLGA/PEG NPs
Synergistic combination of two or more therapeutic agents attracts increasingly attentions as a hopeful approach to overcome the unfavourable toxicity and other side effects that restrict the efficacy of many drugs. Hence, it is important to discovery a nanocarrier-mediated co-delivery system which can load two or more therapeutic agents in the same carrier and transfer them to the same cancer cells at the same time. Particularly, co-delivery of Cur and Met with different water solubility characteristics was a fundamental challenge in the development of a co-delivery system for their applications simultaneously. Double emulsion approach utilizes a two-step process of preparing a water-in-oil (W/O) emulsion for encapsulation of loads, followed by producing water-in-oil-in-water (W/O/W) emulsion in water employing a combination of surfactants to construct NPs [Citation21]. This process allows formation of NPs with the capability to encapsulate both hydrophilic and hydrophobic therapeutic agents [Citation22]. Wang and colleagues used this method to nanoencapsulation of two anticancer drugs, doxorubicin and paclitaxel, with distinct solubility characteristics into amphiphilic methoxy PEG/PLGA copolymer [Citation23]. In this work, the improved w/o/w method had been applied to achieve the Met–Cur-loaded PLGA/PEG NPs.
Characterization of drug-loaded PLGA/PEG NPs
Size, morphology and drug loading
Like other amphiphilic copolymers, PLGA/PEG could be emulsified in an aqueous solution to form core-shell NPs. The NPs should be small enough to evade detection and destruction by the reticuloendothelial system, to achieve longevity during systemic circulation [Citation24]. The particle size and size distribution of NPs were characterized by a zeta potential analyzer with DLS technique. summarized the average particles size of blank and drug-loaded NPs. PLGA/PEG NPs showed an average size of 202.83 ± 9.23 nm with uniform dispersion of particles and a polydispersity index of 0.176, and its zeta potential was −7.23 ± 0.43 mV. Cur- and Met-loaded PLGA/PEG NPs were larger than blank NPs, indicating that the presence of Cur and Met in the core of the NPs increased the volume. Met–Cur NPs showed the largest diameter with a smaller polydispersity, which might be attributed to insertion of drugs making the amphiphilic copolymers form a well-knit structure.
Table 1. Characterization of PLGA/PEG amphiphilic copolymers and drug-loaded NPs.
The morphology of Met–Cur NPs was further confirmed by Transmission electron microscopy (TEM) imaging (). The TEM image showed that Met–Cur NPs was dispersed as individual particles with a distinct spherical core-shell structure homogeneously distributed around a size of around 170 nm. This spherical shapes and uniform distributions of Met–Cur NPs were further confirmed using FE-SEM image (). As the narrow size distribution measured by DLS, the average hydrodynamic diameter of the drug-loaded NPs was around 220 nm ().
Figure 1. Dynamic light scattering (DLS), Transmission electron microscopy (TEM) and Field emission scanning electron microscopy (FESEM) characterization of Met–Cur–PLGA/PEG NPs showing the core-shell structure of NPs. (A) DLS histogram of Met–Cur NPs, (B) TEM and (C) FE-SEM images.
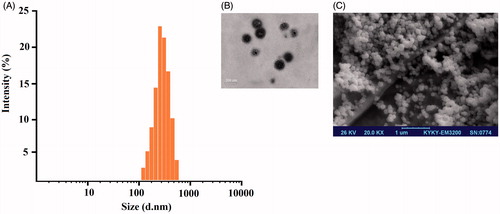
The particle size from TEM (170 nm) was slightly smaller than observed from DLS analysis (202.83 ± 9.23 nm). The discrepancies in sizes were due to that fact that TEM image was acquired on dry samples under vacuum whereas the DLS profile was obtained in a solvated state.
The encapsulation efficiencies of Met and Cur in the NPs were 75.15 and 80.5% at a copolymer/drug with a loading capacity of 12.5 ± 2.1 and 10.1 ± 2.2%, respectively. The high entrapment efficiency of Cur in comparison with Met was attributed to the hydrophobic nature of the anticancer drug.
FTIR spectrum is consistent with the structure of the supposed copolymer. FTIR spectroscopy was applied to demonstrate the structure of PLGA/PEG NPs and drugs loaded PLGA/PEG NPs (). In the case of Cur-loaded PLGA/PEG NPs, the broad peak around 3300–3600 cm−1 indicates the stretching of hydrogen bonded -OH groups. The sharp peaks at 1759 and 1094–1186 cm−1 related to ester group (O-C = O) (C-O) and C-O-C ether group and C-C bonds of PLGA/PEG NPs. The major peaks of Cur were observed at 1625, 1590 and 1513 cm−1 which related to carbonyl group (C = O), and aliphatic and aromatic ethylene C = C bounds, respectively. The observed shift at Cur characteristic peaks may be attributed to probable interaction between PLGA/PEG NPs and Cur. The absorption around 1500–1400 cm−1 indicated the –C–O elongation frequency of –OH groups in Cur and Cur-loaded PLGA/PEG NPs. Our results are in agreement with the reports of Athira et al. [Citation25], and Mohan et al. [Citation26]. The results indicated the successful incorporation and interaction of Cur with PLGA/PEG NPs.
In the case of Met-loaded PLGA/PEG NPs, all the characteristic bands of Met and PLGA/PEG were observed. The broad peak at 3300–3600 cm−1 was attributed to O–H and N–H bands of polymer and drug. The peak at 2362 cm−1 was related to Met C = N of imines stretching vibration. An intense peak at 1759 cm−1 was the characteristic esoteric (O–C = O) bands of PLGA section. The peaks at 1508–16,369 cm−1 are characteristic bands of NH primary bending, NH secondary bending and C–N stretching vibrations of Met. Other characteristic peaks of PLGA/PEG which indicated C–C, C–O and C–O–C were appeared at 1059–1187 cm−1. Therefore, it can be concluded the interaction and loading of Met in PLGA/PEG NPs. In the FTIR spectra of Met–Cur-loaded PLGA/PEG NPs all the characteristic bands related to Met, Cur and PLGA/PEG copolymer was appeared, indicating the accurate preparation of dual Met–Cur encapsulation into PLGA/PEG NPs.
Stability and in vitro drug release
In view of the potential utility of NPs formulation for pharmaceutical applications, stability is significant for long-term storage, transportation and scalable processing. Stability study was carried out by re-dissolving Met–Cur NPs in PBS (pH 4.4, 5.4 and 7.4) at 37 °C for 3 days (conditions for accumulated release testing) to assess the size change. As shown in DLS results of , there was no significant change in the average diameter of Met–Cur NPs during the study, and this stable character made the NPs shows important potentials for clinical implications.
Met and Cur were encapsulated into PLGA/PEG NPs in 1000:1 molar ratio. As shown in , no initial burst release phenomenon was observed for both the drugs. The drugs (Met and Cur) released in a sustained manner from the nanoparticulate systems up to 120 h study period. It has to be noted that Met and Cur has different release pattern with latter released relatively faster than that of Cur which was releasing slowly until the end of release study. For example, at the end of 24 h, approximately 20 ± 2.6% of Cur released comparing to that of 25 ± 3.3% Met from PLGA/PEG NPs. By 120 h, 92 ± 3.43% of Met released from the NPs, whereas only 65 ± 4.2% of Cur released during the same time period. The significant difference (p < .05) in release pattern among two drugs might be due to different hydrophobicity and position of drugs in the PLGA/PEG core. In theory speaking, Met was released at first when the outermost copolymer layer broke off, after that, the core structure with hydrophobic surface disassembled quickly, accompanied by the release of Cur. A sustained release profile for both the drugs would be beneficial for the efficient cancer therapy. Furthermore, a sequential release of drugs would activate the cell apoptosis in cancer tissues.
In vitro cytotoxicity analysis
Here, Met and Cur were used as a pair to demonstrate the synergistic effect of co-delivery of two natural chemotherapeutic agents in the same vehicle. T47D cells were first separately incubated in 96-well plates. After 24 h, the cells were treated for 72 h with the free Met, free Cur, Met NPs, Cur NPs and Met–Cur NPs in different drug concentrations. To assess the combined effects of Met and Cur as a free and nanoformulated mix on T47D cells, we treated T47D cells with Met and Cur in a constant ratio to one another (1000:1) based on their IC50s.
As shown in , free drugs and formulations exhibited a dose-dependent cytotoxicity against T47D cells. Especially, Cur showed higher cytotoxic effect than Met across all the concentrations. As expected, combination of Met–Cur substantially reduced the viability of the cancer cells. Importantly, Met–Cur–PLGA/PEG NPs significantly arrested the growth of cancer cells. The enhanced anticancer effect of Met–Cur–PLGA/PEG NPs was due to the controlled release of therapeutic drugs and high intracellular concentrations. It is well known that free drug easily diffuses into the cell membrane whereas micellar drug takes a specific cellular internalization pathway and releases the drug in a systemized manner [Citation27].
Figure 5. In vitro cytotoxicity of free Met, free Cur, free Met–Cur and Met–Cur–PLGA/PEG NPs against T47D cells incubated for 72 h. The cytotoxicity of formulations was evaluated by MTT assay. The data are presented as mean ± SD (n = 3).
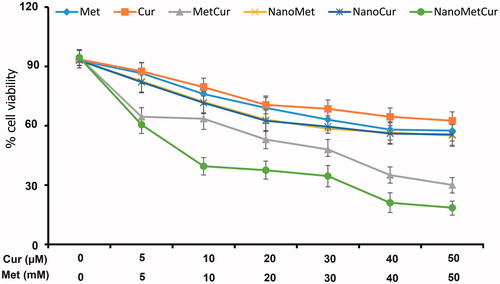
Similar trend IC50 values were calculated to quantitate the amount of drug required to kill 50% of cancer cells. The IC50 values were calculated from GraphPad prism (version 6) software. The IC50 values of Met, NanoMet, Cur, NanoCur, Met–Cur and NanoMet–Cur were 11.54 mM, 8.71 mM, 11.68 μM, 8.87 μM, 6.67 μM and 4.76 μM, respectively, in T47D cells after 72 h incubation. The IC50 values clearly showed the superior performance of combinational NPs. The precise nature of this interaction was further analysed by the median-effect method, where the combination indices (CI) of less than, equal to, and more than 1 indicate synergistic, additive and antagonistic effects, respectively [Citation28]. As shown in , the CIs value were <1 for the free combination and nano-combination treatments, indicating a synergistic effect between Met and Cur on T47D cell proliferation in both free and nanoformulated forms. Also, the combinational nanoparticulate formulations displayed more synergistic effects and induced greater cancer cell killing potency than the cocktail free drugs.
Real-time PCR
The maintenance of telomere length through the expression of telomerase is fundamental to the capability of cancer cells to remain proliferative. hTERT expression is the rate-limiting element of the enzymatic activity of telomerase and is supposed to be a sensitive indicator of telomerase function [Citation29,Citation30]. Real-time PCR was used to quantify hTERT mRNA expression in T47D cells treated with free and encapsulated forms of Met and Cur in different concentration after 72 h incubation (). Real-time PCR results revealed that Cur, Met and combination of Met–Cur in free and encapsulated forms inhibited hTERT gene expression. As shown in , nanoCur and nanoMet inhibited hTERT expression only in higher concentration (8 and 8000 μM, respectively) compared to free components (p < .05), whereas treatment with combination forms decreased hTERT mRNA expression in a dose-dependent manner. Also, it has been found that nano-combination in relative to free combination could further decline in hTERT expression in all concentrations (p < .05). Our results demonstrated that the nano-encapsulated combination form of these components had grater synergistic effect on inhibition of hTERT expression in T47D cells. This data suggests that the nano-combination of Met and Cur inhibits telomerase activity by rapidly decreasing hTERT mRNA levels. However, further studies are needed to provide insight into the other mechanisms involved in the elicited anticancer effects of the combination treatment of Met and Cur.
Figure 7. Real-time PCR analysis. Cur–PLGA/PEG (A) and Met–PLGA/PEG (B) inhibited hTERT expression in higher concentration (8 and 8000 μm) compared to pure components (p < .05). Whereas Met–Cur and Met–Cur–PLGA/PEG NPs decreased hTERT mRNA expression in in a dose dependent manner (C). Nano-combination in relative to free combination could further decline in hTERT expression in all concentration (p < .05).
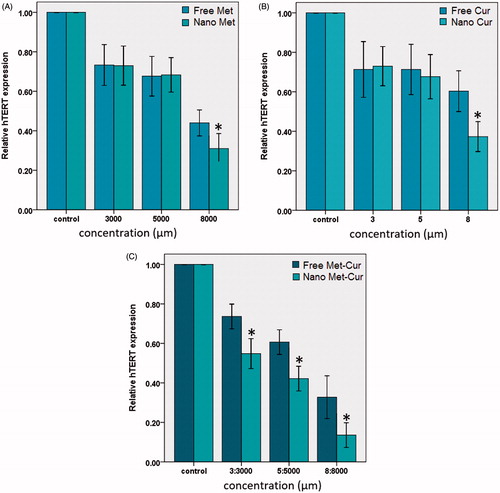
It has been reported that Met can regulate the telomerase activity in cancer cells. In a study by Leigh and colleagues [Citation30], the effect of Met on proliferation and expression of key targets of Met cell signalling especially hTERT in endometrial cancer cell lines was evaluated. Findings revealed that Met potently inhibits the growth in a dose dependent manner in the cell lines. Also, treatment with Met resulted in G1 arrest, induction of apoptosis and decreased hTERT expression. Western immunoblot analysis confirmed that Met induces phosphorylation of AMPK (5' AMP-activated protein kinase), its immediate downstream mediator, within 24 h of exposure. In parallel, treatment with Met decreased phosphorylation of S6 protein, a vital target of the mTOR pathway. Authors concluded that the effect of Met on hTERT expression may potentially occur as an indirect consequence of cell cycle arrest rather than a direct effect on hTERT transcription.
The effect of Cur in decreasing telomerase activity has been detected in several cancer cell lines by a rising number of reports [Citation16,Citation31–33]. This reduction has been realized that is because of the blocking of nuclear localization of telomerase through disconnection chaperone Hsp90–p23 complex from TERT and therefore inhibition from translocation of TERT to the nucleus. Interaction of the Hsp90 and p23 with hTERT is essential for regulating the nuclear transport of telomerase [Citation34]. Furthermore, Cur via down-regulation of TERT expression results in suppressing of telomerase activity in which increasing concentrations of Cur cause a reduced level of TERT mRNA with an unchanged TR mRNA level [Citation35].
Studies revealed that suppression of cancer cells growth by telomerase inhibition did not occur instantly. However, it is a long-term response. Some reports propose that telomerase inhibition merged with other chemotherapeutic agents could decrease this lag period and encourage tumour cell death more efficiently [Citation36].
Conclusions
In summary, nanoparticles prepared by a double emulsion method from copolymer PLGA/PEG were employed as carriers to co-deliver Met and Cur into T47D cells. Based on our findings nanoformulation of Met–Cur combination was able to kill breast cancer cells more rapidly than either treatment alone, thereby potentially this strategy can reduce cytotoxicity and patient side effects. Also, the co-delivery of nanodrugs suppresses hTERT expression more efficiently than the delivery of either Met or Cur at the same concentrations, indicating a synergistic effect. To the best of our knowledge, this is the first study to demonstrate synergy between Met and Cur in combination and nano-combination forms against breast cancer cells. We hope that this work will begin to provide the scientific foundation for future clinical trials of nano-combinational therapy using Met and Cur for breast cancer treatment and ultimately, prevention.
Acknowledgements
The authors thank the Department of Medical Biotechnology, and Medical Nanotechnology, Faculty of Advanced Medical Sciences, Tabriz University of Medical Sciences for all support provided.
Disclosure statement
The authors declare that they have no competing interests.
Additional information
Funding
References
- Eatemadi A, Darabi M, Afraidooni L, et al. Comparison, synthesis and evaluation of anticancer drug-loaded polymeric nanoparticles on breast cancer cell lines. Artif Cells Nanomed Biotechnol. 2016;44:1008–1017.
- Anari E, Akbarzadeh A, Zarghami N. Chrysin-loaded PLGA-PEG nanoparticles designed for enhanced effect on the breast cancer cell line. Artif Cells Nanomed Biotechnol. 2015;44:1410–1416.
- Ghalhar MG, Akbarzadeh A, Rahmati M, et al. Comparison of inhibitory effects of 17-AAG nanoparticles and free 17-AAG on HSP90 gene expression in breast cancer. Asian Pac J Cancer Prev. 2014;15:7113–7118.
- Zeighamian V, Darabi M, Akbarzadeh A, et al. PNIPAAm-MAA nanoparticles as delivery vehicles for curcumin against MCF-7 breast cancer cells. Artif Cells Nanomed Biotechnol. 2016;44:735–742.
- Rahmati-Yamchi M, Zarghami N, Rahbani M, Montazeri A. Plasma leptin, hTERT gene expression, and anthropometric measures in obese and non-obese women with breast cancer. Breast Cancer Basic Clin Res. 2011;5:27.
- Daniel M, Peek GW, Tollefsbol TO. Regulation of the human catalytic subunit of telomerase (hTERT). Gene. 2012;498:135–146.
- Cheng Y-B, Guo L-P, Yao P, et al. C. Telomerase and hTERT: can they serve as markers for gastric cancer diagnosis? World J Gastroenterol. 2014;20:6615.
- Javan-Maasomi Z, Pilehvar-Soltanahmadi Y, Dadashpour M, et al. Synergistic anticancer effects of silibinin and chrysin in T47D breast cancer cells. Asian Pac J Cancer Prev. 2017;18:1283–1287.
- Lv S, Tang Z, Li M, et al. Co-delivery of doxorubicin and paclitaxel by PEG-polypeptide nanovehicle for the treatment of non-small cell lung cancer. Biomaterials. 2014;35:6118–6129.
- Du Q, Hu B, An H-M, et al. Synergistic anticancer effects of curcumin and resveratrol in Hepa1-6 hepatocellular carcinoma cells. Oncol Rep. 2013;29:1851–1858.
- He Z, Huang J, Xu Y, et al. Co-delivery of cisplatin and paclitaxel by folic acid conjugated amphiphilic PEG-PLGA copolymer nanoparticles for the treatment of non-small lung cancer. Oncotarget. 2015;6:42150.
- Duong HHP, Yung L-YL. Synergistic co-delivery of doxorubicin and paclitaxel using multi-functional micelles for cancer treatment. Int J Pharm. 2013;454:486–495.
- Alibakhshi A, Ranjbari J, Pilehvar-Soltanahmadi Y, et al. An update on phytochemicals substances in molecular target therapy of cancer: potential inhibitory effect on telomerase activity. Curr Med Chem. 2016;23:2380–2393.
- Naksuriya O, Okonogi S, Schiffelers RM, et al. Curcumin nanoformulations: a review of pharmaceutical properties and preclinical studies and clinical data related to cancer treatment. Biomaterials. 2014;35:3365–3383.
- Kourelis TV, Siegel RD. Metformin and cancer: new applications for an old drug. Med Oncol. 2012;29:1314–1327.
- Kazemi-Lomedasht F, Rami A, Zarghami N. Comparison of inhibitory effect of curcumin nanoparticles and free curcumin in human telomerase reverse transcriptase gene expression in breast cancer. Adv Pharm Bull. 2013;3:127–130.
- Xiong Y, Zhao Y, Miao L, et al. Co-delivery of polymeric metformin and cisplatin by self-assembled core-membrane nanoparticles to treat non-small cell lung cancer. J Control Release. 2016;244:63–73.
- Badrzadeh F, Akbarzadeh A, Zarghami N, et al. Comparison between effects of free curcumin and curcumin loaded NIPAAm-MAA nanoparticles on telomerase and PinX1 gene expression in lung cancer cells. Asian Pac J Cancer Prev. 2014;15:8931–8936.
- Amirsaadat S, Pilehvar-Soltanahmadi Y, Zarghami F, et al. Silibinin-loaded magnetic nanoparticles inhibit hTERT gene expression and proliferation of lung cancer cells. Artif Cells Nanomed Biotechnol 2017. [Epub ahead of print]. doi: 10.1080/21691401.2016.1276922.
- Sadeghzadeh H, Pilehvar-Soltanahmadi Y, Akbarzadeh A, et al. The effects of nanoencapsulated curcumin-Fe3O4 on proliferation and hTERT gene expression in lung cancer cells. Anticancer Agents Med Chem. 2017;17:1–11
- Mohammadian F, Pilehvar-Soltanahmadi Y, Mofarrah M, et al. Down regulation of miR-18a, miR-21 and miR-221 genes in gastric cancer cell line by chrysin-loaded PLGA-PEG nanoparticles. Artif Cells Nanomed Biotechnol. 2016;44:1972–1978.
- Alimohammadi YH, Joo SW. PLGA-based nanoparticles as cancer drug delivery systems. Asian Pac J Cancer Prev. 2014;15:517–535.
- Wang B, Yu X-C, Xu S-F, et al. Paclitaxel and etoposide co-loaded polymeric nanoparticles for the effective combination therapy against human osteosarcoma. J Nanobiotechnol. 2015;13:1.
- Santander-Ortega MJ, Csaba N, González L, et al. Protein-loaded PLGA–PEO blend nanoparticles: encapsulation, release and degradation characteristics. Colloid Polym Sci. 2010;288:141–150.
- Athira GK, Jyothi AN. Preparation and characterization of curcumin loaded cassava starch nanoparticles with improved cellular absorption. Int J Pharm Pharm Sci. 2014;6:171–176.
- Mohan PK, Sreelakshmi G, Muraleedharan C, et al. Water soluble complexes of curcumin with cyclodextrins: characterization by FT-Raman spectroscopy. Vib Spectrosc. 2012;62:77–84.
- Aslan B, Ozpolat B, Sood AK, et al. Nanotechnology in cancer therapy. J Drug Target. 2013;21:904–913.
- Chou T-C. Drug combination studies and their synergy quantification using the Chou-Talalay method. Cancer Res. 2010;70:440–446.
- Nasiri M, Zarghami N, Koshki KN, et al. Curcumin and silibinin inhibit telomerase expression in T47D human breast cancer cells. Asian Pac J Cancer Prev. 2013;14:3449–3453.
- Holysz H, Lipinska N, Paszel-Jaworska A, et al. Telomerase as a useful target in cancer fighting – the breast cancer case. Tumor Biol. 2013;34:1371–1380.
- Lee JH, Chung IK. Curcumin inhibits nuclear localization of telomerase by dissociating the Hsp90 co-chaperone p23 from hTERT. Cancer Lett. 2010;290:76–86.
- Montazeri M, Pilehvar-Soltanahmadi Y, Mohaghegh M, et al. Antiproliferative and apoptotic effect of dendrosomal curcumin nanoformulation in P53 Mutant and wide-type cancer cell lines. Anticancer Agents Med Chem. 2017;17:662–673
- Montazeri M, Sadeghizadeh M, Pilehvar-Soltanahmadi Y, et al. Dendrosomal curcumin nanoformulation modulate apoptosis-related genes and protein expression in hepatocarcinoma cell lines. Int J Pharm. 2016;509:244–254.
- Hasima N, B Aggarwal B. Targeting proteasomal pathways by dietary curcumin for cancer prevention and treatment. Curr Med Chem. 2014;21:1583–1594.
- Alibakhshi A, Ranjbari J, Pilehvar-Soltanahmadi Y, et al. An update on phytochemicals in molecular target therapy of cancer: potential inhibitory effect on telomerase activity. Curr Med Chem. 2016;23:2380–2393.
- Cerone MA, Londoño-Vallejo JA, Autexier C. Telomerase inhibition enhances the response to anticancer drug treatment in human breast cancer cells. Mol Cancer Ther. 2006;5:1669–1675.