ABSTRACT
The development of effective and eco-friendly insecticides is a demand. Green nanoparticles synthesis is an important field in pest control strategy, which has cost effective and faster than physical and chemical methods. In this study, silver nanoparticles (AgNPs) were synthesized using Artemisia herba-alba plant extract to be tested against an important pest of non-edible plants such as cotton. UV-vis spectrophotometry, TEM, XRD and FTIR were used to confirm the biophysical characterization of AgNP formation, which appeared as spherical particles with size ranging from 9.68 to 36.7 nm. Feeding and contact application methods were used to evaluate the larvicidal activity of green-synthesized AgNPs against the cotton pest Spodoptera littoralis larvae. LC50 and LC90 values of synthesized AgNPs were calculated. After 5 days of treatment, the calculated LC50 of applied AgNPs by feeding application was 74.569 ml/ml (soln./ H2O), whereas contact application recorded LC50 of 27.47 ml/ml after 30 h of treatment. Morphological and histopathological abnormalities after AgNPs treatment were detected as demelanization and mummified shape of larvae, the destruction of the cuticle layer, disintegration of gut epithelia and gonad deformation. Our results proved the success of green-synthesized NPs application against S. littoralis larva, which open new avenues in pest control.
Introduction
The cotton leafworm Spodoptera littoralis is one of the most destructive agricultural pests in the subtropical and tropical ranges of Africa and the Middle East [Citation1]. The insect is considered a devastating pest in its native range. Larvae defoliate ~40 plant families with economically important crops, such as cotton and tomatoes [Citation2]. Insecticides used to control such pests pose many hazards [Citation3], and the need for new ecofriendly bioinsecticides is a must [Citation4–6].
Nanotechnology is a new branch of science with a wide range of applications in the pharmaceutical, medicinal, biotechnological, industrial and agricultural fields [Citation7]. Silver nanoparticles (AgNPs) have been proven to have potential antibacterial, antifungal and larvicidal properties [Citation8,Citation9]. The possibility of using nanotechnology for the development of biopesticides was detected by Scarfato et al. [Citation10] and Debnath et al. [Citation11]. Due to their large surface areas, nanoparticles can bond and adsorb other compounds easily and circulate more easily in lepidopteran and other insect systems [Citation12–14].
The conventional methods of AgNPs synthesis have many limitations and require high energy, pressure and temperature. These processes are usually slow, have high costs and use chemical reducing agents, such as sodium borohydride and dimethylformamide, which have environmental encumbrances [Citation15]. In contrast, using plants for nanoparticle synthesis can be advantageous over other traditional and biological processes. This process is relatively fast as there is no need to maintain specific media and culture conditions [Citation16]. Green nanoparticles are cost-effective, safe and environment-friendly [Citation17]; thus, they can be an economically efficient alternative to chemical insecticides. The green nanoparticle synthesis method uses various plants and their products, such as enzymes, proteins, flavonoids, terpenoids and cofactors, which act as both reducing and capping agents [Citation18].
Recent studies on the biosynthesis of AgNPs have revealed that there was a significant shift in interest toward the use of medicinal flora for nanoparticle synthesis, where the green leaves of medicinal plants have the best capacity to reduce and stabilize AgNPs due to the abundance of reducing components (H+) [Citation19,Citation20]. Artemisia species are widely used to treat certain diseases, such as malaria, hepatitis, cancer, stomach ulcers and wound healing, due to their antibacterial, antifungal, and disinfectant properties [Citation21]. They possess several compounds with insecticidal and anti-feeding activities against many insect pests, such as 3-methyl,3-phenyl-1,4-pentadiyne found in Artemisia monosperma essential oil [Citation22]. Artemisia belongs to Family Asteraceae (Compositae). There are ~250 species of Artemisia worldwide, found in Europe, Asia, Africa and North America [Citation23]. However, few studies have dealt with nanoparticle synthesis from Artemisia species and its cytotoxic and apoptosis effects [Citation19]. Vijayakumar et al. [Citation24], Basavegowda et al. [Citation25] and Mousavi et al. [Citation19] have synthesized nanoparticles from three different species of Artemisia and investigated their antimicrobial activity. Aziz et al. [Citation26] demonstrated that AgNPs synthesized from Artemisia herba-alba showed high larvicidal toxicity against mosquito vectors.
A better understanding of the cytotoxicity, histopathology and safety issues associated with the utilization of nanoparticles toward the target pest could be the limits of this technology and expand their horizon beyond laboratory use [Citation27]. Notably, over 200 studies on the toxicity of nanoparticles to various arthropod species of economic importance have been published in the last 3 years [Citation28,Citation29]. Histopathological effect of AgNPs on midgut region of S. litura and Helicoverpa armigera were detected also by Manimegalai et al. [Citation30].
This study aimed to use endogenous species of Artemisia plants in preparing AgNPs. The potential activity of prepared Artemisia herba-alba AgNPs (Ar-AgNPs) as a new nano-insecticide against S. littoralis (Noctuidae-Lepidoptera) was conducted. Feeding and contact were compared as methods of applications. The morphological and histopathological abnormalities that followed the applications were investigated.
Materials and methods
Preparation of artemisia extract
Artemisia herba-alba was purchased from a local market. Dried leaf powder (10 g) was stirred in a 1:1 ratio of 50 ml deionized water and 50 ml ethanol and boiled for 10 min [Citation24]. The crude extract was filtered and kept at 4°C for further investigation.
AgNP synthesis
For AgNP synthesis, 0.1 M aqueous solution of silver nitrate (AgNO3; 90 ml) was mixed with 10 ml of the aqueous plant extract, and the mixture was continuously stirred for 2 h at 30°C [Citation31]. The mixture must be kept away from light until the colorless solution turns darkish brown, revealing the reduction process of Ag+ to Ag0 nanoparticles. The prepared AgNP solution was kept at 4°C until further investigation.
Characterization of the prepared AgNPs
Ultraviolet-visible (UV-vis) spectroscopy
UV-vis characterization of synthesized AgNPs using A. herba-alba extract was performed using UV-vis spectroscopy (ChromeTech UV-100 spectrophotometer, USA). For UV-vis analysis, 0.1 ml was diluted to 2 ml using deionized water. Silver ions were monitored from 200 to 800 nm [Citation31].
Transmission electron microscopy (TEM)
The morphology and size of synthesized AgNPs were investigated by TEM using a JEM 1400 instrument at an accelerating voltage of 80 kV. For TEM analysis, the sample was prepared by placing one drop of AgNP solution onto a carbon film supported on a copper grid, followed by water evaporation in air at room temperature [Citation32].
X-ray diffraction (XRD)
XRD was used to identify crystalline phases using an X-ray diffractometer (D8 Advance; Bruker, Germany PANalytical X’Pert Pro X-ray machine). The sample was deposited on the sample holder for scanning at the range of 10°–80° [Citation33].
Fourier transform infrared spectroscopy (FTIR)
To examine the presence of functional groups on the surface of synthesized AgNPs, both aqueous extracts of A. herba-alba and synthesized AgNPs were performed by using the FTIR analysis. The measurement was carried out using the KBr pellet method in the wavelength scanning range of 500–4000 cm−1 (4100 Jasco- Japan). The samples were prepared by mixing thoroughly with KBr and then compressed to form an ultimate thin homogenous and transparent disc with thickness of 0.5 mm [Citation34].
Insect rearing
S. littoralis larvae were reared under laboratory conditions of Plant Protection Research Institute, Agriculture Research Center, Egypt [Citation35].
Bioassay tests
A. herba-alba plant extract, an aqueous solution of AgNO3 (0.1 M), and synthesized AgNPs were tested for their activity against S. littoralis 4th instar larvae. The larvae were starved for 3 h [Citation36].
Feeding application
Five concentrations of the plant extract, aqueous solution of AgNO3 (0.1 M) and green-synthesized AgNP solution (Ar-AgNPs) were prepared (0.1, 0.25, 0.5, 0.75 and 0.9) ml/ml. Leaf discs of castor plants were dipped in each preparation for 1 min. Every treatment had three replicates with 15 larvae/replicate kept at 25°C. Mortality readings were recorded every 24 h for 5 days [Citation37]. The regression lines were plotted, and the lethal concentrations, LC50 and LC90, were calculated.
Contact application
For both AgNO3 (0.1 M) and Ar-AgNPs, five concentrations were prepared (0.1, 0.25, 0.5, 0.75 and 0.9 ml/ml (soln./ H2O)) and then 1 ml was sprayed over the plastic cup and allowed to air dry aseptically [Citation38]. Fifteen S. littoralis 4th instar larvae were allowed to crawl over it. Every treatment had three replicates. Mortality readings were taken after 6, 12, 18, 24, 30 and 48 h, and the regression lines were plotted to calculate LC50 and LC90 values. In control experiments, the leaves of castor plant were dipped in distilled water.
Histopathological effects of Ar- AgNPs on S. littoralis larvae
After treatment of S. littoralis 4th instar larvae with LC50 values of Ar-AgNPs, moribund larvae were collected and processed to prepare histological slides from the whole larvae. Microscopic preparations were performed following the method of [Citation39]. Histopathological signs that appeared after feeding or contact applications of AgNPs were recorded and photographed.
Statistical analyses
Probit analysis was done to calculate median lethal concentration (LC50 and LC90) using the Ldp3 and SPSS 21.0 version software package. Significant differences were established at P ≤ 0.05 and P ≤ 0.001 levels [Citation40,Citation41].
Results
AgNP biosynthesis using A. herba-alba plant extract
The color change of the solution from clear to yellowish-brown and then to darkish brown indicates the conversion of silver ions into AgNPs. The yellowish-brown color was remarkable after 20 min of addition of leaf extract to aqueous AgNO3 solution due to AgNP formation. The color intensity increased with reaction time, thus confirming AgNP formation. After 2 h, the intensity of the darkish brown color did not increase, indicating that the reaction was ended ().
UV-vis spectroscopic analysis of AgNPs
UV-vis spectroscopy is considered one of the useful techniques for the preliminary characterization of metal nanoparticles. The maximum absorption peak of synthesized AgNPs was found at 430 nm (). The appearance of a 430 nm peak in UV-vis spectroscopic analysis represents the complete synthesis of AgNPs.
TEM analysis
The TEM image of synthesized AgNPs using A. herba-alba extract (Ar-AgNPs) is shown in . The average particle size ranged from 9.68 to 38 nm, illustrating that AgNPs are predominantly spherical. This result also indicated that synthesized AgNPs are monodispersed without aggregation.
XRD analysis
The XRD pattern of synthesized AgNPs using A. herba-alba extract (Ar-AgNPs) is shown in . The XRD pattern showed diffraction peaks at scattering angles, with 2θ values of 38.19°, 44.55°, 64.44° and 77.42° corresponding to (111), (200), (220) and (311) lattice planes of the silver crystal, respectively ().
Fourier transform infrared spectroscopy (FTIR)
FTIR analysis was used in the present study to identify functional groups of A. herba-alba extract that have roles in reducing, capping and stabilizing synthesized AgNPs. The FTIR spectrum of plant extract recorded a lot of peaks. These spectra were shifted out to fewer peaks with slightly different values after being used in the reduction of silver nitrate to silver nanoparticles (). Some peaks were recorded in AgNPs spectrum only, and these peaks were: 3778, 1884 and 1825 cm−1 bands. In the case of plant extract spectra, there was a broad band with different peaks between 3000 and 3600 cm−1 that indicated the presence of hydroxyl group.
Figure 5. FTIR spectra of A) A. herba-alba plant extract and B) synthesized Ar-AgNPs using A. herba-alba plant extract.
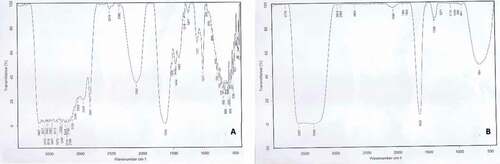
The absorption peaks for plant extract at 3413, 3080 and 2948 cm−1 belonged to the stretching vibrations of N–H, C–H of benzene rings and carboxylic acids, respectively, while the peak at 2841 cm−1 correspond to C-C stretching vibrations of aromatic amines. There was a sharp peak at 2082 cm-1 only in the plant extract and disappeared in AgNPs spectra that likely indicated the presence of C ≡ C bond. There is a huge and sharp peak at 1633 cm−1 for plant extract which slightly shifted to about 1635 cm−1 in AgNPs spectra informed some carbon double bonds which could be the amide I group. A sharp peak at 1017 cm−1 in plant extract spectra was due to C–O for alcohol or ether.
Determination of lethal concentrations
Feeding application
The larvicidal activities of A. herba-alba plant extract, aqueous solution of AgNO3, and synthesized AgNPs were studied against S. littoralis 4th instar larvae. . reveals that the mortality rate increased with increasing concentrations of tested preparations. Mortality percentages ranged from 2.3% to 27.9% with 0.25 to 0.9 ml/ml of the plant extract after 5 days, whereas mortality percentages ranged from 2.33% to 53.49% and from 0% to 74.4% using the same concentrations for AgNO3 solution and synthesized Ar-AgNPs, respectively. LC50 values were 185.51, 94.516 and 74.569 ml/ml for the plant extract, AgNO3 solution and Ar-AgNPs, respectively.
Table 1. Larvicidal activity of the tested solutions against S. littoralis during feeding application
Contact application
Mortality readings were positively correlated to increased concentrations of tested solutions after 30 and 48 h treatment (, respectively). The mortality percentages of Ar-AgNPs reached 100% after 30 h at a concentration of 0.9 ml/ml. LC50 values were 27.466 and 9.419 ml/ml for Ar-AgNPs after 30 and 48 h, respectively.
Table 2. Larvicidal activity of the tested solutions against S. littoralis larvae after 30 h using contact application
Table 3. Larvicidal activity of the tested solutions against S. littoralis larvae after 48 h of contact application
Morphological and histopathological changes after treatment of S. littoralis larvae with AgNPs
Ar-AgNP-fed larvae showed cuticle decolorization in the thoracic region with stop feeding. Larvae appeared like a mummy in contact application . In stop feeding with knockdown after a few hours of application, treated larvae could not perform its curled posture and appeared with relaxed legs .
Figure 6. Morphological effects of Ar-AgNPs on S. littoralis larvae: (A) control, (B) treatment through feeding and (C) treatment through contact.
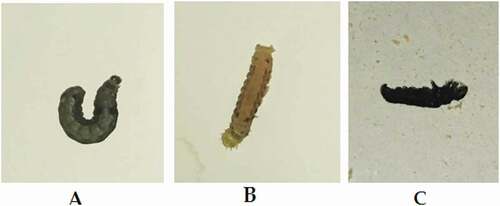
These investigations revealed histological structure alterations in different larval tissues due to treatment with Ar-AgNPs. Midgut epithelial cells and the cuticle were the most affected tissues. shows the deformations after the application of Ar-AgNPs by contact and formation of channel passage I.(c)). These zones seemed as the entrance passage of the nanoparticles. Decolorization in parts of the chitin layer, which lost its integrity with undifferentiated hypodermal cells, was detected.
Figure 7. Histopathological changes after treatment S. littoralis larvae with Ar-AgNPs I. (A) Longitudinal section (L.S.) in normal integument showing a normal cuticle layer. (B) L.S. in treated larvae through feeding showing the treated cuticle layer. (C) L.S. in treated larvae through contact showing an irregular cuticle formation, with decolorization of some parts. Red arrows show the destruction of cuticle and hypodermal layers. CL, cuticle layer; HD, hypodermal layer; DHD, destructed hypodermal layer. II. (A) L.S. in normal midgut showing normal gut epithelia (B) T.S. in treated larvae through feeding showing elongation of epithelial cells, nonhomogeneous cytoplasm, and appearance of vacuoles between cells with destructed muscular layer around the gut epithelia. (C) T.S. in treated larvae through contact showing detachment of epithelial cells from the basement membrane, irregular shape of the epithelial cells, and cytoplasmic particles appeared in the lumen. EPC, epithelial cells; GC, goblet cells; v, vacuoles. III. (A) L.S. in normal tissue showing normal muscle tissue (B) L.S. in treated larvae through feeding showing irregular sarcolemma sheath. (C) L.S. in treated larvae through contact showing distortion of muscle. M, muscles.IV. (A) L.S. in normal fat tissue showing normal fat body full of lipid granules (B) L.S. in treated larvae through feeding showing highly affected fat tissue. (C) T.S. in treated larvae through contact showing vacuoles within the fat tissue and the absence of lipid droplets. Ft, fat body. V. (A) L.S. in normal larvae showing normal trachea. (B) L.S. in treated larvae through feeding showing treated trachea. (C) T.S. in treated larvae through contact showing the treated trachea. The cuticle sheath is ruptured and appeared irregular in shape. T, trachea. VI. (A) L.S. in normal gonads. (B) L.S. in treated larvae through feeding showing highly affected gonads. (C) L.S. in treated larvae through contact showing gonads deformation *Gon, gonads; Sp, spermatogonia.
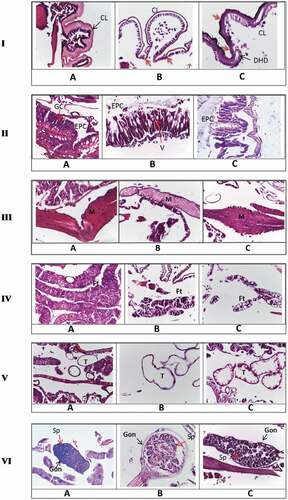
Sections in the gut region of treated larvae through feeding II.(b)) demonstrated elongation of epithelial cells, appearance of vacuoles, absence of brush border, and empty goblet cells. Genetic materials could not be detected within the nucleus. Destruction of the musculature of the gut was also detected. Epithelial cells lost their uniform shape with the appearance of projections toward the lumen. The larval gut region treated with Ar-AgNPs through contact appeared detached from the basement membrane, with the explosion of some epithelial cells into the lumen. Also, no chromatin materials could be seen within the nuclei II.(c)).
The muscular system was also affected III), with appearance of fissures and loss of its band arrangement with irregular sarcolemma sheath, after contact or feeding application. The fat bodies and trachea were also affected IV and V). Treatment with contact application had obvious effects on the respiratory system V.(c)). The cuticle lining of the trachea lost its integrity, explaining the quick death after a few hours of application. The gonads were obviously deformed VI. (b, c)).
Discussion
Plant-mediated AgNPs have been widely examined as alternatives to synthesized chemicals used against insect pests because they are safe, costless and have a simple biosynthesis process [Citation42,Citation43]. The interface between nanotechnology and the field of pest control opens promising avenues in improving agricultural products and controlling disease transmission [Citation44–46]. A few years ago, the green synthesis of nanoparticles was achieved using extracts from plants, fungi, or bacteria [Citation47,Citation48].
The green synthesis of nanoparticles has advantages over chemical methods; it does not cause any hazardous effects to the environment [Citation6]. Recent investigations showed that green-synthesized AgNPs had larvicidal and pupicidal toxicity against a number of insects [Citation49,Citation50]. This study used Artemisia plant to synthesize AgNPs. Different metabolites have been identified from Artemisia spp., such as sesquiterpene lactones, flavonoids, and essential oils [Citation51]. These compounds possess insecticidal and anti-feeding activity against some insects [Citation19,Citation21].
The reduction of silver ions in watery solution to AgNPs is accompanied by the color change from yellow to yellowish-brown or darkish-brown due to the excitation of the vibrating surface plasmon within AgNPs [Citation52,Citation53]. The color change as a result of agglomeration is a well-understood phenomenon [Citation54].
The first confirmation of the successful preparation of AgNPs was the appearance of a 430 nm peak in . The obtained (AgNPs) peak was due to the excitation of surface plasmon resonance SPR of silver atoms. This assured the excitation of conduction electrons in silver [Citation55]. This study agreed with Ga’al et al. [Citation56] and Basu et al. [Citation57].
The spherical shape and very small size of TEM micrographs confirmed the morphological features of AgNPs [Citation58,Citation59]. The high-resolution TEM technique was previously reported as the best method for accurately determining the morphological characteristics of nanoparticles [Citation60].
XRD analysis showed four obvious peaks corresponding to 111, 200, 220 and 311, which were more intense than the other planes [Citation61]. Thus, XRD highlighted that AgNPs formed by the reduction of AgNO3 with Artemisia extract were face-centered cubic and crystalline. Two unpredicted peaks appeared at 32.02° and 36.12°. These peaks might be due to the organic compounds in the leaf extract of Artimisia [Citation62,Citation63].
Based on our observations, we can conclude that functional groups such as hydroxyl, amide and carbonyl ( present in the A. herba-alba extract might be responsible for the bio-reduction of Ag+ to AgNPs and act as reductant, capping and stabilizing agent of AgNPs, these explainations were supported by [Citation64]. FTIR spectra indicate that the plant bio-molecules are active in synthetizing and stabilizing AgNP formation [Citation65,Citation66]. Aziz, et al [Citation26], isolated similar peaks when synthetizing AgNPs using A. herba-albe.
Bioassay results revealed that contact application was highly effective compared to the feeding application. Contact application of Ar-AgNPs induced mortality after 30 h, proving the potential of nanoparticles to deliver insecticides to insects hiding in difficult habitats, such as tree bark or under the skin of animals.
The detected morphological changes could be explained by the reduction in acetylcholine-choline esterase reaction, resulting in paralysis and knockdown of treated larvae. Destruction of the muscular system made larvae unable to take its normal C-shape (). Demelanization I.(b)) was due to the absence of copper tyrosinase, which convert tyrosine to dopaquinone forming melanin pigment. Competition between Ag and Cu ions made Ag ions to replace copper leading to Cu2+ starvation resulting in demelanization [Citation67].
Histopathological preparations assured the destructive effect and structural alterations in different tissues of Ar-AgNP-treated larvae. Contact application of nanoparticles had quick and high toxicity against Spodoptera spp. binds to insect cuticle, followed by wax abrasion, resulting in insect dehydration and mummification I.(c)). Dewaxing and corrosion of the cuticle layer led to the appearance of Ar-AgNP passages in this layer .
Application of Ar-AgNPs through feeding demonstrated various degrees of deformations in the gut region as shown in (II). These observations were compatible with Kalimuthu, et al. [Citation68], who noted that midgut cells of Aedes aegypti 4th instar larvae showed degeneration of nuclei and brush border after exposure to AgNPs. Sundararajan et al. [Citation69] observed histological alterations in the midgut and digestive tract of Ae. aegypti 3rd and 4th instar larvae after treatment with gold nanoparticles. Yasur and Usha [Citation70], in their study on nanoparticle effects on plants and insects, confirmed penetration into cell organelles and localization of nanoparticles at mitochondria or nucleolus in both plant and insect tissues. They suggested the possible use of nanoparticles to deliver pesticides or fertilizers.
The explanation about the mode of action of nanoparticles against insects is still a matter of discussion, and many hypotheses have been made [Citation71–73]. Hazaa et al. [Citation74] reported a substantial elongation in the larval period of S. littoralis larvae after treating with Borago officinalis – AgNPs. The possible overall toxicity of Ar-AgNPs might be due to the presence of a wide range of phytochemicals and volatile compounds in such plant preparations, such as oxygenated sesquiterpenes, phenyls and carboxylic and carbonyl hydrocarbons [Citation75].
This study proved that prepared Ar-AgNPs have significant toxicity against the 4th instar larvae of the cotton leafworm. Green AgNPs appeared to significantly impact Spodoptera antioxidants, leading to oxidative stress and cell death II). Empty nuclei in (II.(c)) may be due to the binding of Ar-AgNPs to S and P molecules in proteins and nucleic acids. Rai et al. [Citation76] assumed that nanoparticle toxicity was through its penetration of the exoskeleton. Destruction of midgut epithelial cells might lead to changes in membrane permeability, distributing the proton motive force and leading to cellular dysfunction and cell death [Citation77,Citation78].
Conclusion and recommendations
Intergraded pest management should include the approaches of nanotechnology instead of traditional strategies based on chemical insecticides. Nanotechnology decreases the use of chemicals insecticide and makes agriculture eco-friendly and profitable. Green synthesis of AgNPs is an environmentally friend method, could be carried out in many applications.
We focused on A. herba-alba, a popular medicinal plant used in folk medicine, as a source of green nano-insecticides against the destructive cotton pest S. littoralis. The color of the aqueous silver nitrate shifted from colorless to darkish brown with the addition of Artemisia extract, which proved the first confirmation of the synthesis of AgNPs. UV-Vis spectroscopy, TEM, XRD and FTIR analyses were used to specify the characteres of the prepared AgNPs. Our findings revealed the potential effects of very low doses of green synthesized nanoparticles on controlling S. littoralis. These findings also suggest that Artemisia AgNPs could play a prospective role in protecting many agricultural products attacked by pests. Histopathological results proved that the mechanism of Ar-AgNPs action is not limited to the gut region. The cuticle, muscles, fat tissue and gonads were also affected. The actual mechanism of AgNPs inside the insect’s body requires more detailed studies and our knowledge is still limited about this field.
Acknowledgments
The authors are grateful to Entomology Department, Faculty of Science, Ain Shams University, for providing the necessary lab facilities to do this work and Dr. Tahany, G.M. Mohammad (Central Agricultural Pesticides Laboratory, Pesticide Formulations Department, Agriculture Research Center, Giza, Egypt) for her kind help in preparation of silver nanoparticles.
Disclosure statement
No potential conflict of interest was reported by the author(s).
References
- Saleh H, Abd El-Rahman S, El-Gably A, et al. Biological and histological effects of certain insecticides on Spodoptera littoralis (bosid). J Plant Protection Pathol. 2021;12(2):111–115. DOI:10.21608/jppp.2021.154391.
- European and Mediterranean Plant Protection Organization (EPPO). European and Mediterranean Plant Protection Organization. Spodoptera litura Fabricious. EPPO Bulletin. 2008;9:142–146.
- Yadav SK. Pesticide applications-threat to ecosystems. J Human Ecol. 2010;32(1):37–45.
- Bulmer MS, Bachelet I, Raman R, et al. Targeting an antimicrobial effector function in insect immunity as a pest control strategy. PNAS. 2009;106(31):12652–12657.
- Zhang S, Xiao-zhen YE. Impacts of chemical insecticides on extracellular protease and chitinase activities of Metarhizium anisopliae. J Coll For. 2010;4:289–292.
- Cloyd RA, Bethke JA. Impact of neonicotinoid insecticides on natural enemies in greenhouse and interiors cape environments. Pest Manag Sci. 2011;67(1):3–9.
- Bhattachayya A, Bhaumik A, Usha Rani P, et al. Nano-particles: a recent approach to insect pest control. Afr J Biotechnol. 2010;9(24):3489–3493.
- Saxena A, Tripathi RM, Singh RP. Biological synthesis of silver nanoparticles by using onion (Allium cepa) extract and their antibacterial activity. Digest J Nanomater Bio Structures. 2010;5:427–432.
- Elumalai EK, Prasad TN, Hemachandran J, et al. Extracellular synthesis of silver nanoparticles using leaves of Euphorbia hirta and their antibacterial activities. J Pharmacol Sci Res. 2010;2(9):549–554.
- Scarfato P, Avallone E, Iannelli P, et al. Synthesis and characterization of polyurea microcapsules containing essential oils with antigerminative activity. J Appl Polym Sci. 2007;105(6):3568–3577. DOI:10.1002/app.26420.
- Debnath N, Das S, Seth D, et al. Entomotoxic effect of silica nanoparticles against Sitophilus oryzae (L.). J Pest Sci. 2011;84(1):99–105. DOI:10.1007/s10340-010-0332-3.
- Valentina Y. Optimization of reactant concentration in biosynthesis of silver nanoparticles using pathogenic bacteria isolated from clinical sources and their characterization. Indian J Microbiol Res. 2020;7(1):63–69.
- Hammer KA, Carson CF, Riley TV, et al. A review of the toxicity of Melaleuca alternifolia (tea tree) oil. Food Chem Toxicol. 2006;44(5):616–625. DOI:10.1016/j.fct.2005.09.001.
- Barik TK, Sahu B, Swain V. Nanosilica from medicine to pest control. Parasitol Res. 2008;103(2):253–258.
- Raja S, Ramesh V, Thivaharan V. Green biosynthesis of silver nanoparticles using Calliandra haematocephala leaf extract, their antibacterial activity and hydrogen peroxide sensing capability. Arabian J Chem. 2017;10(2):253–261.
- Shankar SS, Rai A, Ahmad A, et al. Rapid synthesis of Au, Ag, and bimetallic Au core-Ag shell nanoparticles using neem (Azadirachta indica) leaf broth. J Colloid Interface Sci. 2004;275(2):496–502. DOI:10.1016/j.jcis.2004.03.003.
- Casida JE, Quistad GB. Insecticide targets: learning to keep up with resistance and changing concepts of safety. Agric Chem Biotechnol. 2005;43:185–191.
- Tavakoli F, Salavati-Niasari M, Badiei A, et al. Green synthesis and characterization of graphene nanosheets. Mater Res Bull. 2015;63:51–57.
- Mousavi B, Tafvizi F, Bostanabad SZ. Green synthesis of silver nanoparticles using artemisia turcomanica leaf extract and the study of anti-cancer effect and apoptosis induction on gastric cancer cell line (AGS). Artif Cells Nanomed Biotechnol. 2018;46(sup1):499–510.
- Almasoud N, Alhaik H, Almutairi M, et al. Green nanotechnology synthesized silver nanoparticles: characterization and testing its antibacterial activity. Green Process Synth. 2021;10(1):510–528. DOI:10.1515/gps-2021-0048.
- Carvalho IS, Cavaco T, Brodelius M. Phenolic composition and antioxidant capacity of six Artemisia species. Ind Crops Prod. 2011;33(2):382–388.
- Khan M, Mousa AA, Syamasundar KV, et al. Determination of chemical constituents of leaf and stem essential oils of Artemisia monosperma from central Saudi Arabia. Nat Prod Commun. 2012;7(8):1079–1082. DOI:10.1177/1934578X1200700829.
- Seddiek SA, Ali MM, Khater HF, et al. Anthelmintic activity of the white wormwood, Artemisia herba-alba against heterakis gallinarum infecting Turkey poults. J Med Plant Res. 2011;5:3946–3957.
- Vijayakumar M, Priya K, Nancy FT, et al. Biosynthesis, characterization and anti-bacterial effect of plant-mediated silver nanoparticles using Artemisia nilagirica. Ind Crops Prod. 2013;41:235–240.
- Basavegowda N, Idhayadhulla A, Lee YR. Preparation of Au and Ag nanoparticles using Artemisia annua and their in vitro antibacterial and tyrosinase inhibitory activities. Mater Sci Eng. 2014;43:58–64.
- Aziz AT, Alshehri MA, Panneerselvam C, et al. The desert wormwood (Artemisia herba-alba) – from Arabian folk medicine to a source of green and effective nanoinsecticides against mosquito vectors. J Photochem Photobiol B Biol. 2018;180:225–234.
- Bamal D, Singh A, Chaudhary G, et al. Silver nanoparticles biosynthesis, characterization, antimicrobial activities, applications, cytotoxicity and safety issues: an updated review. Nanomaterials. 2021;11(8):2086. DOI:10.3390/nano11082086.
- Athanassiou CG, Kavallieratos NG, Benelli G, et al. Nanoparticles for pest control: current status and future perspectives. J Pest Sci. 2018;91(1):1–15. DOI:10.1007/s10340-017-0898-0.
- Maroufpour N, Mousavi M, Asgari Lajayer B, Ghorbanpour, M. Biogenic Nanoparticles in the Insect World: Challenges and Constraints . Biogenic Nano-Particles and Their Use in Agro-Ecosystems. 2020;173–185.
- Manimegalai T, Raguvaran K, Kalpana M, et al. Green synthesis of silver nanoparticle using Leonotis nepetifolia and their toxicity against vector mosquitoes of Aedes aegypti and culex quinquefasciatus and agricultural pests of Spodoptera litura and Helicoverpa armigera. Environ Sci Pollut Res. 2020;27(34):43103–43116. DOI:10.1007/s11356-020-10127-1.
- El-Rahman AF A, Tahany GM. Green synthesis of silver nanoparticle using eucalyptus globulus leaf extract and its antibacterial activity. J Appl Sci Res. 2013;9(10):6437–6440.
- Asoro MA, Kovar D, Ferreira PJ. In situ transmission electron microscopy observations of sublimation in silver nanoparticles. ACS Nano. 2013;7(9):7844–7852.
- Das R, Nath SS, Chakdar D, et al. Preparation of silver nanoparticles and their characterization. J Nanotechnol. 2009;5:1–6.
- Alamdari S, Ghamsari MS, Lee C, et al. Preparation and characterization of zinc oxide nanoparticles using leaf extract of Sambucus ebulus. Appl Sci. 2020;10(10):3620. DOI:10.3390/app10103620.
- Hatem A, Azazy A, Abd El-Samad S, et al. Toxicity and bioactivity of feeding cotton leaf worm, Spodoptera littoralis (boisduval) (lepidoptera: noctuidae) larvae on fresh leaves of selected weeds. J Plant Prot Pathol. 2011;2(3):257–273. DOI:10.21608/jppp.2011.86418.
- WHO. Guidelines for Laboratory and field testing of mosquito larvicides. World Health Organization Document WHO. 2005;13:39. cds /WHO-pes/gcdpp/.
- Kasmara H, Melanie NDA, Hermawan, W, et al. The toxicity evaluation of prepared lantana camara nano extract against Spodoptera litura (Lepidoptera: Noctuidae).AIP Conference Proceedings, Indonesia. 2018; 1927:1–7.
- Jafer FS, Annon MR. Larvicidal effect of pure and green-synthesized silver nanoparticles against Tribolium castaneum (herb.) and Callosobruchus maculatus (fab.). J Glob Pharm Technol. 2018;10(3):448–454.
- Kjanijou M, Jiraungkoo K, Kosai P, et al. Effect of Murraya paniculata leaf extract against Culex quinquefasciatus larva. Asian J Biol Sci. 2012;5(4):201–208. DOI:10.3923/ajbs.2012.201.208.
- SPSS. Statistical product and service solution, system user’s guide, version 17.0.0.0. (U.S.A: Polar Engineering and Consultin). 2008. http://www.winwrap.com.
- Finney DF. Probit analysis. J Pharm Sci. 1971;60:1432–1432.
- Kumar PM, Murugan K, Madhiyazhagan P, et al. Biosynthesis, characterization, and acute toxicity of Berberis tinctoria-fabricated silver nanoparticles against the Asian tiger mosquito, Aedes albopictus, and the mosquito predators Toxorhynchites splendens and Mesocyclops thermocyclopoides. Parasitol Res. 2016;115(2):751–759. DOI:10.1007/s00436-015-4799-y.
- Kumar D, Kumar G, Agrawal V. Green synthesis of silver nanoparticles using Holarrhena antidysenterica (L.) Wall.bark extract and their larvicidal activity against dengue and filariasis vectors. Parasitol Res. 2018;117(2):377–389.
- Roni M, Murugan K, Panneerselvam C, et al. Characterization and biotoxicity of Hypnea musciformis-synthesized silver nanoparticles as potential eco-friendly control tool against Aedes aegypti and Plutella xylostella. Ecotoxicol Environ Saf. 2015;121:31–38.
- Sujitha V, Murugan K, Paulpandi M, et al. Green-synthesized silver nanoparticles as a novel control tool against dengue virus (DEN-2) and its primary vector Aedes aegypti. Parasitol Res. 2015;114(9):3315–3325. DOI:10.1007/s00436-015-4556-2.
- Benelli G, Lukehart CM. Special issue: applications of green-synthesized nanoparticles in pharmacology, parasitology and entomology. J Cluster Sci. 2017;28(1):1–2.
- Rajan R, Chandran K, Harper SL, et al. Plant extract synthesized silver nanoparticles: an ongoing source of novel biocompatible materials. Ind Crops Prod. 2015;70:356–373.
- Benelli G, Pavela R, Maggi F, et al. Commentary: making green pesticides greener? The potential of plant products for nanosynthesis and pest control. J Cluster Sci. 2017;28(1):3–10. DOI:10.1007/s10876-016-1131-7.
- Govindarajan M, Kadaikunnan S, Alharbi NS, et al. Single-step biological fabrication of colloidal silver nanoparticles using Hugonia mystax: larvicidal potential against zika virus, dengue, and malaria vector mosquitoes. Artif Cells Nanomed Biotechnol. 2017;45(7):1317–1325. DOI:10.1080/21691401.2016.1228664.
- Azarudeen RMST, Govindarajan M, AlShebly MM, et al. Size-controlled biofabrication of silver nanoparticles using the Merremia emarginata leaf extract: toxicity on Anopheles stephensi, Aedes aegypti and Culex quinquefasciatus (diptera: culicidae) and non-target mosquito predators. J Asia-Pacific Entomol. 2017;20(2):359–366. DOI:10.1016/j.aspen.2017.02.007.
- Mohamed AEHH, El-Sayed MA, Hegazy ME, et al. Chemical constituents and biological activities of Artemisia herba-alba. Rec Nat Prod. 2010;4:1–25.
- Gao X, Wei L, Yan H, et al. Green synthesis and characteristic of core-shell structure silver/ starch nanoparticles. Mater Lett. 2011;65(19–20):2963–2965. DOI:10.1016/j.matlet.2011.06.020.
- Hamedi S, Masumeh S, Shojaosadati S, et al. Comparative study on silver nanoparticles properties produced by green methods. Iran J Biotechnol. 2012;10(3):191–197.
- Ihegwuagu N, Mundi S, Adama F, et al. Rapid synthesis of silver nano particles capped in starch and its anti–mold activity. Int J Innov Sci Res. 2014;9(1):16–25.
- Deepak P, Sowmiya R, Ramkumar R, et al. Structural characterization and evaluation of mosquito-larvicidal property of silver nanoparticles synthesized from the seaweed, Turbinaria ornata (turner) J. Agardh 1848. Artif Cells Nanomed Biotechnol. 2017;45(5):990–998. DOI:10.1080/21691401.2016.1198365.
- Ga’al H, Fouad H, Mao G, et al. Larvicidal and pupicidal evaluation of silver nanoparticles synthesized using Aquilaria sinensis and Pogostemon cablin essential oils against dengue and zika viruses vector Aedes albopictus mosquito and its histopathological analysis. Artif Cells Nanomed Biotechnol. 2018;46(6):1171–1179. DOI:10.1080/21691401.2017.1365723.
- Basu S, Maji P, Ganguly J. Rapid green synthesis of silver nanoparticles by aqueous extract of seeds of Nyctanthes arbor-tristis. Appl Nanosci. 2016;6(1):1–5.
- Akbari B, Pirhadi T, Zandrahimi M. Particle size characterization of nanoparticles a practical approach. Iran. Iran J Mater Sci Eng. 2011;8(2):48–56.
- Ihegwuagu NE, Sha’Ato R, Tor-Anyiin TA, et al. Facile formulation of starch–silver-nanoparticle encapsulated dichlorvos and chlorpyrifos for enhanced insecticide delivery. New J Chem. 2016;40(2):1777–1784. DOI:10.1039/C5NJ01831E.
- Thakore S, Rathore PS, Jadeja RN, et al. Sunflower oil mediated biomimetic synthesis and cytotoxicity of monodisperse hexagonal silver nanoparticles. Mater Sci Eng C Mater Biol Appl. 2014;44:209–215.
- Saifuddin N, Nian CY, Zhan LW, et al. Chitosan-silver nanoparticles composite as point-of-use drinking water filtration system for household to remove pesticides in water. Asian J Biochem. 2011;6(2):142–159. DOI:10.3923/ajb.2011.142.159.
- Suvith VS, Philip D. Catalytic degradation of methylene blue using biosynthesized gold and silver nanoparticles. Spectrochimica Acta Part A, Molecular & Biomolecular Spectroscopy. 2014;118:526–532.
- Jeeva K, Thiyagarajan M, Elangovan V, et al. Caesalpinia coriaria leaf extracts mediated biosynthesis of metallic silver nanoparticles and their antibacterial activity against clinically isolated pathogens. Industrial Crops & Products. 2014;52:714–720.
- Islam NU, Jalil K, Shahid M, et al. Green synthesis and biological activities of gold nanoparticles functionalized with Salix alba. Arab J Chem. 2019;12(8):2914–2925. DOI:10.1016/j.arabjc.2015.06.025.
- Saraswathi VS, Santhakumar K. Green synthesis of silver nano-particles mediated using lagerstroemia speciosa and photocatalytic activity against Azo dye. Mech Mater Sci Eng J. 2017;9. DOI:10.2412/mmse.72.63.602 .
- Jemal K, Sandeep BV, Pola S. Synthesis, characterization, and evaluation of the antibacterial activity of Allophylus serratus leaf and leaf derived callus extracts mediated silver nanoparticles. J Nanomater. 2017;2017:1–11.
- Armstrong N, Ramamoorthy M, Lyon D, et al. Mechanism of silver nanoparticles action on insect pigmentation reveals intervention of copper homeostasis. PLOS ONE. 2013;8(1):e53186. DOI:10.1371/journal.pone.0053186.
- Kalimuthu K, Panneerselvam C, Chou C, et al. Control of dengue and zika virus vector Aedes aegypti using the predatory copepod Megacyclops formosanus: synergy with Hedychium coronarium-synthesized silver nanoparticles and related histological changes in targeted mosquitoes. Process Saf Environ Prot. 2017;109:82–96.
- Sundararajan B, Ranjitha Kumari BD. Novel synthesis of gold nanoparticles using Artemisia vulgaris L. leaf extract and their efficacy of larvicidal activity against dengue fever vector aedes aegypti L. J Trace Elem Med Biol. 2017;43:187–196.
- Yasur J, Rani PU. Lepidopteran insect susceptibility to silver nanoparticles and measurement of changes in their growth, development and physiology. Chemosphere. 2015;124:92–102.
- Ahmed KS, Mikhail WZA, Sobhy HM, et al. Impact of nanosilver- profenofos on cotton leafworm, Spodoptera littoralis (boisd.) larvae. Bull National Res Centre. 2019;43(1):46. DOI:10.1186/s42269-019-0076-z.
- Hany AF, Hosam MK, Hammam EG, et al. Nanosilica and jasmonic acid as alternative methods for control Tuta absoluta (Meyrick) in tomato crop under field conditions. Arch Phytopathol Plant Prot. 2016;49(13):362–370. DOI:10.1080/03235408.2016.1219446.
- Tunçsoy BS. Toxicity of nanoparticles on insects: a review. Artibilim Adana Bilim ve Teknoloji Üniversitesi Fen Bilimleri Dergisi. 2018;1(2):49–61.
- Hazaa M, Alm-Eldin M, Ibrahim AE, et al. Biosynthesis of silver nanoparticles using borago officinslis leaf extract, characterization and larvicidal activity against cotton leaf worm, Spodoptera littoralis (bosid). Int J Trop Insect Sci. 2021;41(1):145–156. DOI:10.1007/s42690-020-00187-8.
- Zhang Z, Han XM, Wei JH, et al. Compositions and antifungal activities of essential oils from agarwood of Aquilaria sinensis (lour.) gilg induced by lasiodiplodia theobromae (pat.) griffon. and maubl. J Braz Chem Soc. 2014;25:20–26.
- Rai M, Kon K, Ingle A, et al. Broad-spectrum bioactivities of silver nanoparticles: the emerging trends and future prospects. Appl Microbiol Biotechnol. 2014;98(5):1951–1961. DOI:10.1007/s00253-013-5473-x.
- Jiang X, Miclăuş T, Wang L, et al. Fast intracellular dissolution and persistent cellular uptake of silver nanoparticles in CHO-K1 cells: implication for cytotoxicity. Nanotoxicology. 2015;9(2):181–189. DOI:10.3109/17435390.2014.907457.
- Benelli G. Plant-mediated biosynthesis of nanoparticles as an emerging tool against mosquitoes of medical and veterinary importance: a review. Parasitol Res. 2016;115(1):23–34.