Abstract
We present the complete mitochondrial genomes of the Critically Endangered whitespotted wedgefish, Rhynchobatus djiddensis (Forsskål, 1775), and bottlenose wedgefish, Rhynchobatus australiae (Whitley, 1939), with the R. djiddensis mitogenome documented for the first time. The genomes for R. djiddensis and R. australiae are 16,799 and 16,805 bp in length, respectively. Both comprise 13 protein-coding regions, 22 tRNA genes, two rRNA genes, and a non-coding control region. All protein-coding regions consistently start with the ATG start codon; however, the alternative start codon GTG is observed at the start of the COX1 gene. NADH2, COX2, and NADH4 have incomplete stop codons: T or TA, and tRNALeu and tRNASer, have atypical codons: UAA, UGA, GCU, and UAG. The phylogenetic analysis places R. djiddensis and R. australiae within the Rhynchobatus genus, separate from other families in the order Rhinopristiformes. We also highlight the most variable gene regions to expedite future primer design, of which NADH2 was the most variable (4.5%) when taking gene length into account. These molecular resources could promote the taxonomic resolution of the whitespotted wedgefish species complex and aid in the genetic characterization of populations of these and related species.
Introduction
Rhino rays (wedgefishes and giant guitarfishes) from the subclass Elasmobranchii are the most imperiled marine taxa in the world, with all but one of the 16 described species classified as Critically Endangered according to the International Union for Conservation of Nature (IUCN) Red List of Threatened Species, and all are listed in Appendix II of the Convention on International Trade in Endangered Species (CITES) (Choo et al. Citation2021; IUCN Citation2022). This is due to a combination of their K-selected life history traits, presence in shallow waters which intersect with coastal fisheries, and overexploitation through targeted and incidental catch, driven by the need for animal protein and the trade in their high-value fins (Kyne et al. Citation2020). Furthermore, significant taxonomic uncertainty is associated with the Rhynchobatus genus; thus, individuals recorded in e.g. fisheries landings are often synonymized as a single species complex referred to as the whitespotted wedgefish complex. Molecular taxonomic studies of rhino rays are mostly limited in scope to single genetic markers (Aschliman et al. Citation2012) which are not always accessible for the relevant species and do not enable fine-scale population genetic analyses. The lack of clear evolutionary significant units and molecular resources has compromised species-specific fishery and demographic data and further impedes assessments of conservation status, enforcement of laws and management of these highly threatened species (Henderson et al. Citation2016; Kyne et al. Citation2020). In particular, the whitespotted wedgefish, Rhynchobatus djiddensis (Forsskål, 1775), and the bottlenose wedgefish, Rhynchobatus australiae (Whitley, 1939), found across the Southwest Indian Ocean region have an extremely high risk of extinction and lack of baseline information (White et al. Citation2014; Kyne et al. Citation2020; Daly et al. Citation2021).
As such, the aim was to assemble and annotate the complete mitogenomes of R. djiddensis and R. australiae from high-throughput sequencing data and to infer the phylogenetic placement of these two species within the order Rhinopristiformes. We also highlight the most variable gene regions to aid with primer design and the amplification of alternative mitochondrial markers. Future studies can utilize the genomic resources developed here to refine species identification and genetic characterization in these and related species.
Materials and methods
Samples, DNA extraction, and high-throughput sequencing
In this study, the R. djiddensis specimen was collected in Sodwana Bay, KwaZulu-Natal, South Africa (27.5565° S, 32.6673° E; sample ID: SALS-050.2) and the R. australiae specimenin Unguja, Zanzibar, Tanzania (6.1357° S, 39.3621° E; sample ID: FID7731). The specimens were morphologically identified (please see for references images) and confirmed by molecular species identification based on the cytochrome oxidase c subunit 1 (COX1) and nicotinamide adenine dinucleotide hydride dehydrogenase subunit 2 (NADH2). Fin-clip samples and DNA are stored at the Genetics Department of Stellenbosch University, South Africa (http://www.sun.ac.za/english/faculty/agri/genetics, Aletta Bester-van der Merwe, [email protected]). Total genomic DNA was extracted from fin-clip samples using a standard cetyltrimethylammonium bromide extraction protocol (Sambrook and Russell Citation2001). The quality and quantity were assessed using a NanoDrop™ ND 2000 spectrophotometer (Thermo Fisher Scientific, Waltham, MA). Low-coverage whole-genome sequencing was performed on an Ion Torrent S5™ System at the Central Analytical Facility at Stellenbosch University, South Africa. All sequencing reads were quality filtered using Torrent Suite™ Software.
Figure 1. Species reference images of (A) Rhynchobatus djiddensis (Photo by the South African Institute for Aquatic Biodiversity), the whitespotted wedgefish, with prominent black markings between eyes, large number of white spots and black pectoral marking surrounded by four or more white spots and (B) Rhynchobatus australiae (Photo by John Nevill), the bottlenose wedgefish, with bottle-shaped snout slightly constricted near tip, three white spots aligned over the pectoral marking (usually two spots below), a short line of well-demarcated white spots on the mid dorsal surface and no spots on the tail (TL = 137 cm).
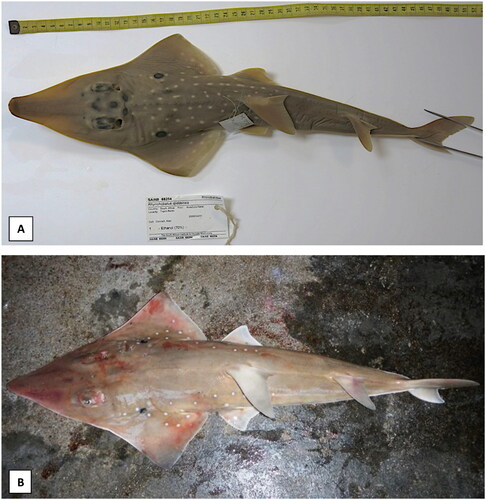
Mitogenome assembly, phylogenetic reconstruction, and variation analysis
Ion Torrent reads were mapped to the previously published mitogenome of R. australiae (KU746924) using the Geneious Read Mapper algorithm with default parameters (Kearse et al. Citation2012). The consensus sequences were annotated with the web-based tool MitoAnnotator (Iwasaki et al. Citation2013) and the Sequence Manipulation Suite (Stothard Citation2000) was used to check for translation errors. The annotated mitogenome sequences can be accessed via GenBank under accession numbers ON065568 for R. djiddensis and ON065567 for R. australiae. The mitogenomes were drawn into full circular genome with Proksee (https://proksee.ca) which uses CGView.js as its genome drawing engine (Grant and Stothard Citation2008). Strand asymmetry was measured based on the following formulas: AT skew = (A – T)/(A + T) and GC skew = (G – C)/(G + C) (Perna and Kocher Citation1995).
We inferred the phylogenetic placement of R. djiddensis and R. australiae by aligning the mitogenomes against 13 whole mitogenome sequences from the order Rhinopristiformes (), using MAFFT (Katoh and Standley Citation2013) with the L-INS-i algorithm on Geneious (Kearse et al. Citation2012). Two mitogenomes from Arhynchobatidae and three from Rajidae were included as outgroups. The alignment was based on 13 concatenated protein-coding genes of each mitogenome. The nucleotide substitution models that best fitted the alignment were determined in PhyloSuite using PartitionFinder2 (Lanfear et al. Citation2016) according to the Akaike’s information criterion with correction for small sample size (AICc) as recommended by Lanfear et al. (Citation2016). Sequences were partitioned into coding domain sequences (CDS) to account for the divergent phylogenies of sequence regions undergoing unique evolution. Maximum-likelihood (ML) inference of the phylogenetic relationships among mitogenomes was performed using IQ-TREE (Nguyen et al. Citation2015) with 1000 bootstrap replicates and Bayesian inference (BI) was performed in MRBAYES v3.2.7a (Ronquist et al. Citation2012) with 2,000,000 MCMC generations and the first 500,000 generations discarded as burn-in. The consensus tree was visualized with The Interactive Tree Of Life (iTOL) v5 (Letunic and Bork Citation2021). Additionally, the mitogenomes from this study and the online R. australiae sequence (KU746924) were aligned in Geneious as previously described, whereafter Mega 11 (Tamura et al. Citation2021) was used to identify the most variable regions across all protein-coding genes and the control region between the species.
Table 1. Whole mitogenomes from the order Rhinopristiformes used to infer the phylogenetic placement of R. djiddensis (ON065568) and R. australiae (ON065567).
Results
The obtained mitogenomes for R. djiddensis and R. australiae comprised 16,799 and 16,805 nucleotides, respectively (). The overall base composition of the R. djiddensis genome was A: 32.3%, T: 27.7%, C: 26.7%, and G: 13.3%; and of the R. australiae genome was A: 32.3%, T: 27.6%, C: 26.9%, and G: 13.2%. Protein-coding gene regions were highly conserved between species, as commonly seen in elasmobranchs (Díaz-Jaimes et al. Citation2016).
Figure 2. Gene maps of the Rhynchobatus djiddensis (ON065568) and Rhynchobatus australiae (ON065567) mitogenomes. The outermost circle genes are transcribed clockwise and the rest counter clockwise.
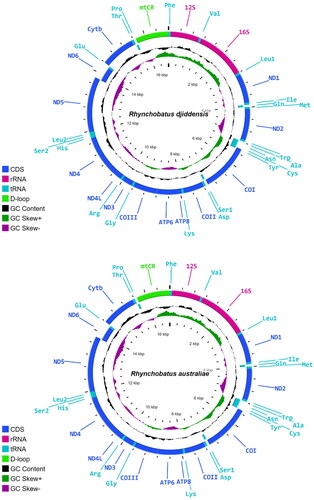
The phylogenetic reconstruction places R. djiddensis and R. australiae within the genus Rhynchobatus, with the closest relationship to the other two wedgefish species, R. laevis and R. australiae (). In addition to the placement of our specimens, the previously reported sample of R. laevis (Johri, Tiwari, et al. Citation2020) clusters within R. australiae with strong support in our ML analysis. Johri, Tiwari, et al. (Citation2020), however, used NADH2 genes to assess the phylogenetic placement of R. laevis where it also resided within the clade representing Rhynchobatus, but separate to other sister species. In our BI analysis, R. laevis clusters the same as in Johri, Tiwari, et al. (Citation2020), with slightly less statistical support than our ML analysis (Figure S1).
Figure 3. Maximum-likelihood phylogeny of five families from the order Rhinopristiformes based on the concatenated dataset of 13 protein-coding genes, with members of the families Rajidae and Arhynchobatidae included as outgroups. Numbers at nodes indicate bootstrap values. GenBank accession numbers are given adjacent to the species name, scale bar indicates groupings of species into families and the taxa in bold are the mitogenomes from this study.
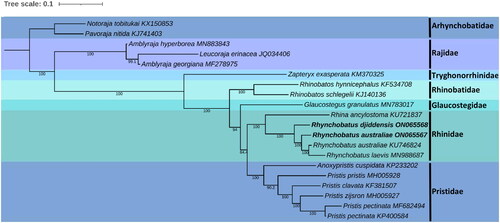
The number of single nucleotide polymorphisms (SNPs) per gene region, and relative variation accounting for gene length, are listed in . A total of 481 SNPs were detected between the species (ambiguous sites were not included). NADH5 was the most variable in terms of total number of SNPs (75), followed by COX1 (53) and NADH2 (47). Taking gene length into account, NADH2 was most variable (4.493%), followed by NADH6 (4.239%) and NADH5 (4.052%), with the control region ranking 7th (3.7%).
Table 2. Single nucleotide polymorphisms (SNPs) found in 16 regions of the mitogenomes from this study; Rhynchobatus djiddensis (ON065568) and Rhynchobatus australiae (ON065567), and the online-available R. australiae sequence (KU746924).
Discussion
There is a pronounced need for updated and accurate genomic resources, particularly in the marine environment. Advances in high-throughput sequencing technologies have led to the development of genomic resources with great potential for applications in marine conservation, yet large-scale sequence information for elasmobranchs remains relatively scarce with limited genetic resources especially for batoids (Pearce et al. Citation2021). In this study, the use of low-coverage whole-genome sequencing data enabled the development of molecular resources, namely the complete mitogenomes of R. djiddensis and R. australiae. While our phylogenetic reconstruction supports the monophyly of Rhynchobatus and finds similar relationships as previous studies (Si, Ding, et al. Citation2016; Johri, Fellows, et al. Citation2020; Kousteni et al. Citation2021), full resolution of the order is limited by the lack of available data. Future studies should focus on generating and analyzing whole mitochondrial genomes for batoids, which is more attainable than a few years ago due to recent technological advancements. Additionally, the previously reported sample of R. laevis (Johri, Tiwari, et al. Citation2020) grouped within R. australiae in our ML analysis but separately in our BI analysis. Phylogenetic uncertainty translates to taxonomic uncertainty (Chafin et al. Citation2021), and the latter is significantly associated with the Rhynchobatus genus. Our mitogenome analysis also highlights areas of variability to serve as a guideline for locating and designing primers to target the most informative mitochondrial regions in R. djiddensis and R. australiae, with NADH2 being the most variable. This challenges the suitability, at least within the Rhynchobatus genus, of the more conventional control region and COX1 as standard mitochondrial markers for population studies or barcoding of elasmobranch species (Feutry et al. Citation2014; Klein et al. Citation2019). Our findings can be used to resolve misidentification issues and taxonomic disputes within the whitespotted wedgefish complex, as it is a prerequisite to the efficient implementation of conservation efforts and associated management based on elasmobranch population structuring patterns (Dudgeon et al. Citation2012; Bester-van der Merwe and Gledhill Citation2015). The taxonomic uncertainty within the Rhynchobatus genus is highlighted; therefore, these genomic resources in combination with those already developed, can support the sustainable management of wedgefishes using a precautionary approach.
Ethical approval
Samples were collected with the necessary permits. Ethical clearance was provided by the Research Ethics (Animal Care and Use) committee in the form of an Animal Notification with reference number #ACU-2021-21616.
Author contributions
MJG: conceptualization, methodology, formal analysis, and writing – original draft. JDK: conceptualization, supervision, and writing – review and editing. RHB: conceptualization, sample collection, funding acquisition, and writing – review and editing. AEB: conceptualization, funding acquisition, project administration, supervision, and writing – review and editing.
Supplemental Material
Download PDF (366.2 KB)Supplemental Material
Download PDF (111.7 KB)Supplemental Material
Download PDF (148.8 KB)Acknowledgements
Special thanks are given to the Wildlife Conservation Society Tanzania for assisting with the collection of samples, and Michaela van Staden for help with the analyses. The authors acknowledge collaboration with the Ministry of Blue Economy and Fisheries of the Revolutionary Government of Zanzibar. This research complies with the International Union for Conservation of Nature (IUCN) and the Convention on the Trade in Endangered Species of Wild Fauna and Flora (CITES) policy statements.
Disclosure statement
There are no financial conflicts of interest to disclose.
Data availability statement
The genome sequence data that support the findings of this study are openly available in GenBank of NCBI (https://www.ncbi.nlm.nih.gov/) under the accession numbers ON065567–ON065568. The associated BioProject, SRA, and Bio-Sample numbers are PRJNA886704, SRX17782697–SRX17782698, and SAMN31139799–SAMN31139800, respectively.
Additional information
Funding
References
- Aschliman NC, Nishida M, Miya M, Inoue JG, Rosana KM, Naylor GJP. 2012. Body plan convergence in the evolution of skates and rays (Chondrichthyes: Batoidea). Mol Phylogenet Evol. 63(1):28–42.
- Bester-van der Merwe AE, Gledhill KS. 2015. Molecular species identification and population genetics of chondrichthyans in South Africa: current challenges, priorities and progress. Afr Zool. 50(3):205–217.
- Castillo-Páez A, del Río-Portilla MA, Oñate-González E, Rocha-Olivares A. 2014. The mitochondrial genome of the banded guitarfish, Zapteryx exasperata (Jordan and Gilbert, 1880), possesses a non-coding duplication remnant region. Mitochondrial DNA A DNA Mapp Seq Anal. 27(3):1–3.
- Chafin TK, Douglas MR, Bangs MR, Martin BT, Mussmann SM, Douglas ME. 2021. Taxonomic uncertainty and the anomaly zone: phylogenomics disentangle a rapid radiation to resolve contentious species (Gila robusta complex) in the Colorado River. Genome Biol Evol. 13(9).
- Chen X, Ai W, Shi X, Gao T. 2015. Mitochondrial genome of the ringstraked guitarfish Rhinobatos hynnicephalus (Elasmobranchii: Rajiformes). Mitochondrial DNA. 26(4):653–654.
- Chen X, Kyne PM, Pillans RD, Feutry P. 2016. Complete mitochondrial genome of the endangered narrow sawfish Anoxypristis cuspidata (Rajiformes: Pristidae). Mitochondrial DNA A DNA Mapp Seq Anal. 27(6):4172–4173.
- Chen X, Wiley T, Kyne PM, Feutry P. 2016. Complete mitochondrial genome of the Critically Endangered smalltooth sawfish Pristis pectinata (Rajiformes: Pristidae). Mitochondrial DNA A DNA Mapp Seq Anal. 27(5):3331–3332.
- Chen X, Ai W, Xiang D, Pan L, Shi X. 2016. Complete mitogenome of the brown guitarfish Rhinobatos schlegelii (Rajiformes, Rhinobatidae). Mitochondrial DNA A DNA Mapp Seq Anal. 27(1):310–311.
- Choo MY, Choy CPP, Ip YCA, Rao M, Huang D. 2021. Diversity and origins of giant guitarfish and wedgefish products in Singapore. Aquat Conserv Mar Freshwater Ecosyst. 31(7):1636–1649.
- Daly R, Parker D, Cliff G, Jordaan GL, Nomfundo N, Bennett RH, Mann BQ. 2021. Long‐term catch trends and risk assessment of the Critically Endangered white‐spotted wedgefish (Rhynchobatus djiddensis) from South Africa. Aquat Conserv Mar Freshwater Ecosyst. 31(4):777–788.
- Díaz-Jaimes P, Bayona-Vásquez NJ, Adams DH, Uribe-Alcocer M. 2016. Complete mitochondrial DNA genome of bonnethead shark, Sphyrna tiburo, and phylogenetic relationships among main superorders of modern elasmobranchs. Meta Gene. 7:48–55.
- Díaz-Jaimes P, Bonfil R, Palacios-Barreto P, Bolaño-Martinez N, Bayona-Vásquez NJ. 2018. Mitochondrial genome of the Critically Endangered smalltooth sawfish Pristis pectinata from Veracruz, Mexico. Conserv Genet Resour. 10(4):663–666.
- Dudgeon CL, Blower DC, Broderick D, Giles JL, Holmes BJ, Kashiwagi T, Krück NC, Morgan JAT, Tillett BJ, Ovenden JR, et al. 2012. A review of the application of molecular genetics for fisheries management and conservation of sharks and rays. J Fish Biol. 80(5):1789–1843.
- Feutry P, Kyne PM, Pillans RD, Chen X, Naylor GJ, Grewe PM. 2014. Mitogenomics of the speartooth shark challenges ten years of control region sequencing. BMC Evol Biol. 14(1):232.
- Feutry P, Kyne PM, Grewe PM, Chen X, Liu M. 2015. Whole mitogenome of the endangered dwarf sawfish Pristis clavata (Rajiformes: Pristidae). Mitochondrial DNA. 26(2):329–330.
- Grant JR, Stothard P. 2008. The CGView Server: a comparative genomics tool for circular genomes. Nucleic Acids Res. 36(Web Server Issue):W181–W184.
- Henderson AC, Reeve AJ, Jabado RW, Naylor GJP. 2016. Taxonomic assessment of sharks, rays and guitarfishes (Chondrichthyes: Elasmobranchii) from south-eastern Arabia, using the NADH dehydrogenase subunit 2 (NADH2) gene. Zool J Linn Soc. 176(2):399–442.
- IUCN. 2022. The Red List of Threatened Species. Version 2022-1; [accessed 2022 Jun 18]. https://www.iucnredlist.org.
- Iwasaki W, Fukunaga T, Isagozawa R, Yamada K, Maeda Y, Satoh TP, Sado T, Mabuchi K, Takeshima H, Miya M, et al. 2013. MitoFish and MitoAnnotator: a mitochondrial genome database of fish with an accurate and automatic annotation pipeline. Mol Biol Evol. 30(11):2531–2540.
- Johri S, Tiwari A, Kerr EN, Dinsdale EA. 2020. Mitochondrial genome of the smoothnose wedgefish Rhynchobatus laevis from the Western Indian Ocean. Mitochondrial DNA B Resour. 5(3):2083–2084.
- Johri S, Fellows SR, Solanki J, Busch A, Livingston I, Mora MF, Tiwari A, Cantu VA, Goodman A, Morris MM, et al. 2020. Mitochondrial genome to aid species delimitation and effective conservation of the Sharpnose Guitarfish (Glaucostegus granulatus). Meta Gene. 24:100648.
- Katoh K, Standley DM. 2013. MAFFT Multiple Sequence Alignment Software Version 7: improvements in performance and usability. Mol Biol Evol. 30(4):772–780.
- Kearse M, Moir R, Wilson A, Stones-Havas S, Cheung M, Sturrock S, Buxton S, Cooper A, Markowitz S, Duran C, et al. 2012. Geneious Basic: an integrated and extendable desktop software platform for the organization and analysis of sequence data. Bioinformatics. 28(12):1647–1649.
- Klein J, Bester-van der Merwe A, Dicken M, Emami-Khoyi A, Mmonwa K, Teske P. 2019. Genomic resources for the spotted ragged-tooth shark Carcharias taurus. Afr J Mar Sci. 41(1):115–118.
- Kousteni V, Mazzoleni S, Vasileiadou K, Rovatsos M. 2021. Complete mitochondrial DNA genome of nine species of sharks and rays and their phylogenetic placement among modern elasmobranchs. Genes. 12(3):324.
- Kyne PM, Wang J-J, Xiang D, Chen X, Feutry P. 2018. The phylogenomic position of the Critically Endangered largetooth sawfish Pristis pristis (Rhinopristiformes, Pristidae), inferred from the complete mitochondrial genome. Mitochondrial DNA B Resour. 3(2):970–971.
- Kyne PM, Jabado RW, Rigby CL, Dharmadi D, Gore MA, Pollock CM, Herman KB, Cheok J, Ebert DA, Simpfendorfer CA, et al. 2020. The thin edge of the wedge: extremely high extinction risk in wedgefishes and giant guitarfishes. Aquat Conserv Mar Freshwater Ecosyst. 30(7):1337–1361.
- Lanfear R, Frandsen PB, Wright AM, Senfeld T, Calcott B. 2016. PartitionFinder 2: new methods for selecting partitioned models of evolution for molecular and morphological phylogenetic analyses. Mol Biol Evol. 34(3):772–773.
- Letunic I, Bork P. 2021. Interactive Tree Of Life (iTOL) v5: an online tool for phylogenetic tree display and annotation. Nucleic Acids Res. 49(W1):W293–W296.
- Nguyen L-T, Schmidt HA, von Haeseler A, Minh BQ. 2015. IQ-TREE: a fast and effective stochastic algorithm for estimating maximum-likelihood phylogenies. Mol Biol Evol. 32(1):268–274.
- Pearce J, Fraser MW, Sequeira AMM, Kaur P. 2021. State of shark and ray genomics in an era of extinction. Front Mar Sci. 8.
- Perna NT, Kocher TD. 1995. Patterns of nucleotide composition at fourfold degenerate sites of animal mitochondrial genomes. J Mol Evol. 41(3).
- Ronquist F, Teslenko M, van der Mark P, Ayres DL, Darling A, Höhna S, Larget B, Liu L, Suchard MA, Huelsenbeck JP, et al. 2012. MrBayes 3.2: efficient Bayesian phylogenetic inference and model choice across a large model space. Syst Biol. 61(3):539–542.
- Sambrook J, Russell D. 2001. Molecular cloning. A laboratory manual. New York (NY): Cold Spring Harbor Laboratory Press.
- Si R, Ding W, Chen H, Chen X, Ai W. 2016. Complete mitochondrial genome and the phylogenetic position of the White-spotted guitarfish Rhynchobatus australiae (Rajiformes, Rhinobatidae). Mitochondrial DNA B Resour. 1(1):315–317.
- Si R, Chen H, Ai W, Chen X, Chen S. 2016. Complete mitochondrial genome and the phylogenetic position of the Bowmouth guitarfish Rhina ancylostoma (Rajiformes, Rhinobatidae). Mitochondrial DNA B Resour. 1(1):270–272.
- Stothard P. 2000. The Sequence Manipulation Suite: JavaScript programs for analyzing and formatting protein and DNA sequences. BioTechniques. 28(6):1102–1104.
- Tamura K, Stecher G, Kumar S. 2021. MEGA11: molecular evolutionary genetics analysis version 11. Mol Biol Evol. 38(7):3022–3027.
- White J, Simpfendorfer CA, Tobin AJ, Heupel MR. 2014. Spatial ecology of shark-like batoids in a large coastal embayment. Environ Biol Fishes. 97(7):773–786.