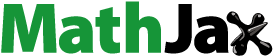
Abstract
The mineralization of collagen scaffolds can improve their mechanical properties and biocompatibility, thereby providing an appropriate microenvironment for bone regeneration. The primary purpose of the present study is to fabricate a synergistically intra- and extrafibrillar mineralized collagen scaffold, which has many advantages in terms of biocompatibility, biomechanical properties, and further osteogenic potential. In this study, mineralized collagen scaffolds were fabricated using a traditional mineralization method (ie, immersed in simulated body fluid) as a control group and using a biomimetic method based on the polymer-induced liquid precursor process as an experimental group. In the polymer-induced liquid precursor process, a negatively charged polymer, carboxymethyl chitosan (CMC), was used to stabilize amorphous calcium phosphate (ACP) to form nanocomplexes of CMC/ACP. Collagen scaffolds mineralized based on the polymer-induced liquid precursor process were in gel form such that nanocomplexes of CMC/ACP can easily be drawn into the interstices of the collagen fibrils. Scanning electron microscopy and transmission electron microscopy were used to examine the porous micromorphology and synergistic mineralization pattern of the collagen scaffolds. Compared with simulated body fluid, nanocomplexes of CMC/ACP significantly increased the modulus of the collagen scaffolds. The results of in vitro experiments showed that the cell count and differentiated degrees in the experimental group were higher than those in the control group. Histological staining and micro-computed tomography showed that the amount of new bone regenerated in the experimental group was larger than that in the control group. The biomimetic mineralization will assist us in fabricating a novel collagen scaffold for clinical applications.
Introduction
Although autografts and allografts have been used to reconstruct bone defects caused by tumor resections or traumas in clinic, significant drawbacks still remain, including the morbidity of the donor site, the limited volume of available bone, the host’s immunoreactions, and even some infectious diseases. Thus, repairing large bone defects continues to be a challenge for bone regeneration. With the development of bone tissue engineering, scaffolds fabricated using different biomaterials, such as bioactive glasses, polylactic acid, collagen, chitosan, and hydroxyapatite (HA), have been explored for bone regeneration due to their biocompatibility, sufficient supply, and no secondary damage for patients.Citation1–Citation7 Among these biomaterials, type 1 collagen and HA, as the predominant organic and inorganic components of bone extracellular matrix (ECM), are always considered to be the ideal materials for scaffolds.Citation5 Natural bone tissue is a complex hierarchical system of synergistically intra- and extrafibrillar mineralized collagen fibers.Citation1 The mineral phase within bone and dentin can be divided into two primary existing forms: intrafibrillar mineralization, which is located inside or near the gap zones of collagen microfibrils and stretches along the long axis of the fibrils, and extrafibrillar mineralization, which is deposited outside the collagen fibrils.Citation8 Therefore, an ideal collagen scaffold should not only mimic the native bone ECM in composition but also reproduce its microstructure of intra- and extrafibrillarly mineralized collagen fibers.Citation9
To date, mixing collagen with minerals and mineralizing collagen have been used to modify collagen scaffolds for simulating the composition and microstructure of bone tissue to the greatest extent possible.Citation10–Citation17 Because HA is a primary mineral component of natural bone and possesses outstanding bioactivity, osteoconductivity, biocompatibility with bone cells, good mechanical properties, and a slow degradation rate in vivo, it has often been the first choice for modifying collagen scaffolds.Citation10,Citation18–Citation20 Although directly mixing collagen with HA powders in certain proportions can result in collagen scaffolds with compositions similar to those of natural bone tissues, it is difficult to simulate their microstructure and microenvironment via this process. Therefore, to solve the aforementioned problems, researchers are devoting more attention to the biomimetic mineralization of collagen scaffolds.Citation12,Citation16,Citation17,Citation21–Citation23 The aforementioned works primarily focus on depositing HA crystals outside the collagen fibrils using traditional mineralization methods, such as immersing the collagen scaffolds in different modified simulated body fluids (SBFs). This process is called extrafibrillar mineralization.Citation9,Citation23,Citation24 Although extrafibrillar mineralization can produce composite scaffolds that are similar to natural bone ECM in terms of chemical composition and a small fraction of structures, it is incapable of simulating the nanoscale hierarchical self-assembled structure in the mineralized collagen fibrils of natural bone.Citation9 More recently, some researchers have demonstrated that collagen scaffolds mineralized using a polymer-induced liquid precursor (PILP) mineralization process based on nonclassical crystallization theory can achieve a bone-like nanostructure with HA nano-crystals embedded within collagen fibrils. The biomimetic mineralization through this process is called intrafibrillar mineralization.Citation8,Citation9,Citation14,Citation25–Citation27 In contrast to the classical crystallization pathways, the nonclassical crystallization route involves mesoscopic transformations of self-assembled, metastable, or amorphous precursor particles into nanoparticulate superstructures.Citation28,Citation29 Because this process assembles materials from the nanoscopic scale to large sizes, it is also called the bottom-up strategy.Citation8,Citation30 Many previous experiments and studies have shown that biomineralization is a bottom-up process based on the theory of nonclassical crystallization.Citation8,Citation28,Citation31 The key of the PILP process is to find negatively charged polymers for stabilizing amorphous precursor particles to achieve liquid–liquid phase separation. Many stabilizers have been used in previous experiments, such as polyaspartic acid, polyacrylic acid, poly-l-glutamic acid, etc, which are analogs of dentine matrix protein-1 (DMP-1).Citation25,Citation32–Citation34 DMP-1 is an important acidic noncollagenous protein delivered at the beginning of mineralized matrix formation in bone and dentine. It has been demonstrated that DMP-1 can stabilize amorphous calcium phosphate (ACP) nanoclusters to form nanocomplexes of DMP-1/ACP by self-assembly, revealing special functions during the mineralization in the gap zone of collagen.Citation35–Citation38 The minor phase formed in this process is composed of metastable, amorphous, liquid-phase nanodroplets, which are the precursors of ACP.Citation25 It has been proposed that these precursors can be drawn into the interstices of the collagen fibrils by capillary forces of fluidic nature. Subsequently, due to the transformation of ACP to HA, collagen fibrils are embedded with HA nanocrystals similar to the natural bone structure.Citation39,Citation40
Based on this bionic concept, we have found a new type of analog called chitosan, which is derived from the shells of shellfish and natural polysaccharides of some fungal cell walls. It has been reported that carboxymethyl chitosan (CMC), the derivative of chitosan enriched in carboxyl groups, can retard or inhibit the rate of spontaneous calcium phosphate precipitation.Citation41 In our previous study, we demonstrated that CMC can stabilize ACP to form liquid-phase nanocomplexes of CMC/ACP, which can aid in intrafibrillar mineralization of collagen using the bottom-up strategy based on nonclassical crystallization theory, thereby facilitating the remineralization of demineralized dentine.Citation37 Therefore, this prompted us to consider using CMC to imitate the function of the important noncollagenous protein, dentin matrix protein, and then biomimetically mineralize the type 1 collagen scaffolds for bone tissue regeneration.
The objective of the present study was to manufacture a mineralized three-dimensional biomimetic mineralized collagen scaffold (BMC) based on biomimetic theory and to compare it with a non-mineralized collagen scaffold (NMC) and traditional mineralized collagen scaffold (TMC) by SBF in terms of morphology, mechanical properties, and biological properties through in vivo and in vitro experiments. The hypothesis of this study was that the collagen scaffold can undergo synergistic intra- and extrafibrillar mineralization by the nanocomplexes of CMC/ACP based on PILP theory. The BMC scaffold, which was similar to natural bone ECM in terms of chemical composition and microstructure, can better promote the regeneration of bone in defects.
Materials and methods
Fabrication of BMC, TMC, and NMC scaffolds
The modified SBF was prepared by dissolving NaCl (7.996 g/L), NaHCO3 (0.350 g/L), KCl (0.244 g/L), K2HPO4∙3H2O (0.288 g/L), MgCl2∙6H2O (0.305 g/L), CaCl2 (0.278 g/L), Na2SO4 (0.071 g/L), and tris(hydroxymethyl) aminomethane (6.057 g/L) in distilled water as described previously by Shakir et al.Citation42 The pH was adjusted to 7.4 using 1 mol/L HCl at room temperature. The CMC/ACP mineral solution was prepared by mixing 1% CMC into 40 mL of water under stirring (1,000 rpm) until the CMC powder was completely dissolved. Then, 0.052 g of K2HPO4 was added to the CMC solution under stirring (500 rpm). Next, 0.074 g of CaCl2 was added to 10 mL of deionized water, and this solution was added dropwise into the CMC solution under stirring (500 rpm) for 5 minutes to form the CMC/ACP solution. The final concentrations of calcium and phosphate ions were 10 mM and 6 mM, respectively.
The method for preparing type 1 collagen used in this experiment was modified according to that of Price.Citation43 Rat tail tendons from Sprague Dawley (SD) rats were dissolved in acetic acid (0.03 mol/L) for 3 days, and then the collagen solution was centrifuged in a refrigerated centrifuge (4°C, 3,000 rpm, 30 minutes). The supernatant was placed in dialysis bags to dialyze for 3–5 days in dipotassium phosphate solution (0.02 mol/L). Then, the collagen hydrogel collected from the dialysis bags was placed in deionized water, SBF, or CMC/ACP mineral solution for a period of time, followed by lyophilization.
Characterization of BMC, TMC, and NMC scaffolds
Scanning electron microscopy
The micromorphology of the scaffolds was observed using a scanning electron microscope (SEM; Nova NanoSEM 430; FEI, Hillsboro, OR, USA). Prior to the observations, the samples were sputter-coated with gold under an argon atmosphere using a sputter coater (K575XD, Emitech; Quorum Technologies Ltd., East Sussex, UK).
X-ray diffraction analysis
X-ray diffraction (XRD) patterns were used to determine the mineral phases of the samples. The samples were scanned with Cu Kα X-ray radiation at 40 kV and 20 mA over the 2θ range of 10°–60°.
Swelling tests
The three different scaffolds were weighed after lyophilization at dry condition (Wd). Then the scaffolds were soaked in normal saline for 10, 20, 30, 40, 50, 60, 70, and 80 minutes at 37°C and weighed at different time points (Ww). The swelling ratio was calculated using the formula:
Each experiment was repeated three times.
Mechanical properties
The three different scaffolds were prepared into definite specimens (2 mm in thickness, 8 mm in diameter) for tensile strength measurements. The testing results were obtained using an ultimate tensile test machine (3367; Instron, Norwood, MA, USA) with a crosshead speed of 5 mm/min under both wet (soaking in normal saline for 0.5 hours in advance) and dry conditions. Subsequently, the elastic moduli of the specimens were computed and recorded using a Bluehill System (Instron, Norwood, MA, USA).
Biodegradation of the scaffold in vitro
The different scaffolds were immersed in 1 mol/L phosphate-buffered saline (PBS; pH 7.4) with lysozyme (0.5 mg/mL; Sigma-Aldrich, St Louis, MO, USA). They were incubated under sterile conditions at 37°C. The solution was replaced every 3 days with fresh medium. Samples were taken out from the medium at different testing points, washed with distilled water, lyophilized, and weighed. The extent of degradation was calculated using the equation: D = (W − W0)/W0 ×100%, where D represents mass loss, W0 is the initial weight of different scaffolds, and W is the weight after degradation for 3, 5, 7, 14, 21, and 28 days. Each experiment was repeated three times.
Quantitative analysis of minerals within mineralized scaffolds
The three different kinds of samples of the same quality were divided into dry group and wet group. Afterward, the different kinds of samples in dry group were soaked in 5.25% sodium hypochlorite with sufficient shock. Next, the solution was centrifuged (3,000 rpm, 15 minutes). The precipitate was lyophilized for weighing. After soaking in normal saline for 0.5 hours, the samples in wet group were processed in the same way as the samples in dry group. The mineral content of each specimen was calculated by the formula:
Transmission electron microscopy and selected-area electron diffraction
Transmission electron microscopy (TEM) was performed to investigate the intra- and extrafibrillar mineralization of different scaffolds. Briefly, 2% glutaraldehyde was used to fix the specimens. Then, the specimens were dehydrated in an ascending ethanol series from 50% to 100%. Subsequently, they were immersed in propylene oxide and embedded in epoxy resin. Ultrathin sections were examined using a TEM (JEOL, Tokyo, Japan) operating at 180 kV. Selected-area electron diffraction patterns were used to determine the crystallinity of the specimens.
Cell and incubation conditions
MC3T3-E1 osteoblasts were cultured in Dulbecco’s Modified Eagle’s Medium (DMEM) containing 10% fetal bovine serum, 100 mg/mL streptomycin, and 100 U/mL penicillin. The three types of scaffolds were cut into discs of diameter 15 mm to match the well size of a 24-well plate and then sterilized using gamma rays (cobalt-60) at a dose of 25 kGy (Institute of Radiation Medicine, Chinese Academy of Medical Sciences and Peking Union Medical College, People’s Republic of China). These sterilized scaffolds were prewetted with complete growth medium (DMEM with 10% fetal bovine serum, 100 mg/mL streptomycin, and 100 U/mL penicillin) prior to adding osteoblasts. Then, 10,000 osteoblasts per 1,000 μL of medium were seeded onto each sterilized scaffold placed in a new 24-well plate, which were maintained in a 5% CO2 incubator at 37°C for various culturing intervals (ie, 1,3, 5, and 7 days).
Cell assays
Live/dead cell double staining and immunofluorescent staining
To observe the distribution and morphology of cells seeded on each scaffold, live/dead cell double staining and immunofluorescent staining were carried out. The different scaffolds were removed from the 24-well plates after 1, 3, 5, and 7 days, washed three times with PBS, placed in new plates, and then stained with an acridine orange/ethidium bromide solution (100 mL per well). For immunofluorescent staining, the cells were fixed with 4% paraformaldehyde after being cultured for 12 hours. Alexa Fluor 488 Phalloidin (Sigma-Aldrich) and 4′,6-diamidino-2-phenylindole (Thermo Fisher Scientific, Waltham, MA, USA) were used to label the F-actin and nuclei of 3T3-E1 cells. The osteoblasts on the samples were observed by laser scanning confocal microscopy (Fv-1000; Olympus, Tokyo, Japan).
Cell proliferation
To study cell proliferation on different scaffolds, 1×104 3T3-E1 cells were seeded onto BMC, TMC, and NMC scaffold disks (n=3) and cultured in 24-well plates with 1 mL of culture medium for various culturing intervals (ie, 1, 3, 5, and 7 days), and then 10 μL per well of Cell Counting Kit-8 (CCK-8; Solarbio, Beijing, People’s Republic of China) and 90 μL per well of fresh medium were added to the 24-well plates to replace the original culture medium. These plates were then incubated in a 5% CO2 incubator at 37°C for 4 hours. Subsequently, an aliquot of the supernatant solution (80 mL) was transferred to a 96-well plate, and the absorbance was measured at 450 nm using a microplate reader (RT-6000; Rayto, Guangdong, People’s Republic of China). Corrected absorbance values were obtained by subtracting the blank absorbance (medium without cells) from the obtained absorbance.
Alkaline phosphatase activity
To determine the differentiation of 3T3-E1 at different time points, an alkaline phosphatase (ALP) experiment was performed. 3T3-E1 cells seeded onto BMC, TMC, and NMC scaffolds were cultured in DMEM for 3, 5, or 7 days (n=3). ALP activity was determined using an ALP assay kit (NJJ-CBIO, Nanjing, People’s Republic of China).
Animal study
The Institutional Ethics Committee of the Tianjin Medical University approved the use of SD rats for the experiments conducted in this study. The animal study was performed to evaluate the performances of different scaffolds in vivo. The skull-penetrating defect model of SD rats was established to compare the bone regeneration of different scaffolds. The 8-week-old rats (250 g; male) were divided into four groups: 1) control, 2) BMC scaffold, 3) TMC scaffold, and 4) NMC scaffold. The rats were administered general anesthesia via an intraperitoneal injection of chloral hydrate (1 mL/330 g; Yulong, Yangshuo, People’s Republic of China). After exposure of the parietal calvarium, a full-thickness skull defect (5 mm in diameter) was prepared with a trephine bur, which was cooled by continuously dripping sterile saline. Then, the different scaffolds (5 mm in diameter) were implanted into the defects, and the incisions were closed with sutures using a 5-0 suture line. In the control group, the defects were sutured without scaffolds.
Micro-computed tomography
To investigate bone regeneration in situ, micro-computed tomography (CT) scans were performed using a Micro-CT (Sky Scan 1174v2, SkyScan N.V., Kontich, Belgium). The test settings were as follows: each specimen was scanned for 360 rotational steps under an X-ray voltage of 50 kVp, anode current of 800 μA, and exposure time of 1,700 ms. The images were used to reconstruct tomograms with a software package called NRecon.
Histological preparation and evaluation
The animals were sacrificed by injecting an overdose of pentobarbital sodium at the end of different experimental stages (4 weeks and 8 weeks), and then the bone defects with surrounding cranial tissues were fixed in 10% neutral buffered formalin. Subsequently, specimens were decalcified in a 17% ethylenediaminetetraacetic acid solution, dehydrated in an ascending graded series of alcohol, and embedded in paraffin. In the center of the bone defects, 5 μm sequential transverse sections were performed and stained with hematoxylin and eosin (H&E) and Masson’s trichrome (Baso Diagnostics Inc., Zhuhai, Peoples Republic of China) so that they could be observed by light microscopy (BX51; Olympus).
Statistical analysis
Statistical analyses were performed using Student’s t-test. All quantitative experiments were repeated at least three times. Values were expressed as mean ± standard deviation. Statistical significance was considered at P<0.05.
Results
Micromorphology and characterization of NMC, TMC, and BMC scaffolds
As shown in , the collagen molecules extracted from rat tail tendons completed self-assembly into collagen fibrils in K2HPO4 solution (0.02 mM) and arranged themselves into nanofibers with distinctive cross-bands of 67 nm periods. Compared to the mineralized scaffolds, fibrils without mineralization had a smaller diameter. The nanofibers of the TMC scaffold () were the thickest because the larger apatite crystals gathered on the outside of the nanofibers. The apatite crystals made the fibers hard and brittle, and thus, fractures were visible on these fibers. Conversely, the BMC scaffold () mineralized via the PILP process made the nanoapatites regularly arranged within the collagen fibrils and nanofiber; therefore, the distinctive cross-bands on the nanofibers were more evident. shows the BMC scaffold at low magnification, demonstrating that collagen fibrils interweave into a network with porous microstructures.
Figure 1 SEM photographs showing the morphologies of NMC, TMC, and BMC scaffolds.
Notes: (A) NMC scaffold at high magnification; the arrow shows collagen fibrils without mineralization. (B) TMC scaffold at high magnification; the arrows show collagen fibrils mineralized by SBF after 24 hours. (C) BMC scaffold at high magnification; the arrows show collagen fibrils mineralized by CMC/ACP after 24 hours. (D) BMC scaffold at low magnification; the arrows show porous microstructures of BMC scaffold. The upper right illustrations represent local amplification of each photograph, respectively.
Abbreviations: ACP, amorphous calcium phosphate; CMC, carboxymethyl chitosan; SBF, simulated body fluid; SEM, scanning electron microscopy; BMC, biomimetic mineralized collagen; NMC, non-mineralized collagen; TMC, traditional mineralized collagen.

To further confirm the level of mineralization, TEM was used to analyze the relationship between collagen fibrils and HA crystals, as shown in . In general, fibrils of the collagen scaffold are not visible due to their low material contrast. shows the distinctive cross-band of collagen fibrils within the NMC scaffold after being stained by uranyl acetate. Without staining, HA nanocrystals of the BMC scaffold () depositing inside and near the gap zones of collagen fibrils made the cross-band as clear as being stained. However, the apatite crystals were located outside the fibrils of the TMC scaffold () because of the large size, which was consistent with the SEM results.
Figure 2 TEM and SAED characterization of NMC, TMC, and BMC scaffolds.
Notes: (A) Image of NMC scaffolds stained by uranyl acetate; the arrow shows distinctive cross-band of collagen fibrils without mineralization. (B) Image of TMC scaffolds unstained; the arrows show the apatite crystals located outside of the fibrils of TMC scaffold. The lower left illustration (SAED) shows obvious ring pattern characteristic of crystal structure. (C) Image of BMC scaffolds without staining; the arrows show HA nanocrystals deposit inside and nearby the gap zones, which make the cross-band quite clear. The lower left illustration (SAED) indicates that the main composition is amorphous phase.
Abbreviations: BMC, biomimetic mineralized collagen; NMC, non-mineralized collagen; TMC, traditional mineralized collagen; HA, hydroxyapatite; SAED, selected-area electron diffraction; TEM, transmission electron microscopy.

presents the XRD patterns of the three different collagen scaffolds. The curve of the TMC scaffold presented three prominent crystalline peaks at 28.9°, 31.7°, and 45.3° (2θ), which were the distinctive diffraction peaks of HA crystals. The curve of the BMC scaffold presented broad and inconspicuous peaks at the same points, indicating that the minerals within the collagen were in the amorphous state. The XRD pattern of the NMC scaffold had no obvious peaks compared with the results of the other two scaffolds.
Figure 3 XRD plots for NMC, TMC, and BMC scaffolds.
Note: The distinctive diffraction peaks’ sites of HA crystals can be seen as arrows.
Abbreviations: BMC, biomimetic mineralized collagen; NMC, non-mineralized collagen; TMC, traditional mineralized collagen; HA, hydroxyapatite; XRD, X-ray diffraction.

The results of swelling ratio of different scaffolds at different time points are shown in . The swelling ratios of these three different samples are 889%±4% (NMC scaffolds), 972%±6% (BMC scaffolds), and 1,141%±6% (TMC scaffolds). All the swelling degrees exceeded 100%. The good absorbent characteristic and porous structures of collagen-based scaffolds may be the main reasons of the high swelling property.
Figure 4 Swelling ratios of NMC, TMC, and BMC scaffolds.
Note: Error bars represent mean ± SD for n=3.
Abbreviations: BMC, biomimetic mineralized collagen; NMC, non-mineralized collagen; TMC, traditional mineralized collagen; SD, standard deviation; min, minutes.

shows a comparison of the elastic moduli on different collagen scaffolds under dry and wet conditions. The results showed that mineralization significantly enhanced the elastic moduli of the collagen scaffolds under both wet and dry conditions (P<0.05). Compared with the TMC scaffold (21.64±2.33), the elastic modulus of the BMC scaffold (41.64±3.72) clearly increased (P<0.01). Both collagen (NMC) scaffolds and mineralized collagen (TMC and BMC) scaffolds decreased markedly in terms of the elastic modulus under wet conditions (P<0.01), although the BMC scaffolds still had a significantly higher modulus compared with the other two scaffolds (P<0.05).
Figure 5 Elastic modulus of NMC, TMC, and BMC scaffolds at dry and wet conditions.
Notes: Error bars represent mean ± SD for n=4. In dry conditions, the difference on elastic modulus among three subgroups is statistically significant (**P<0.01; n=4). In wet conditions, the difference is also statistically significant (*P<0.05; n=4).
Abbreviations: BMC, biomimetic mineralized collagen; NMC, non-mineralized collagen; TMC, traditional mineralized collagen; SD, standard deviation.

The mineral content of each scaffold is shown in . The results show that the mineral content of TMC and BMC scaffolds has no statistically significant difference before and after wetting. The trace amount of mineral in NMC scaffolds is likely to come from the inorganic salt solution during the preparation of rat tail tendons from SD rats.
Figure 6 Mineral content of NMC, TMC, and BMC scaffolds at dry and wet conditions.
Notes: Error bars represent mean ± SD for n=3. There is no statistically significant difference on mineral content of different collagen scaffolds before and after wetting (*P>0.05; n=3).
Abbreviations: BMC, biomimetic mineralized collagen; NMC, non-mineralized collagen; TMC, traditional mineralized collagen; SD, standard deviation.

shows the biodegradation of the different scaffolds in vitro. The results reveal that the greatest loss of mass occurred in NMC scaffolds after incubation in PBS with lysozyme. Approximately 80% of the NMC scaffolds were degraded within 28 days, whereaŝ50% of the mineralized collagen (TMC and BMC) scaffolds were degraded under the same conditions. The degradation rates of BMC scaffolds were significantly slower than those of the other two groups (P<0.05). By contrast, mineralization can significantly protect collagen from degradation in PBS with lysozyme, especially synergistic intra- and extrafibrillar mineralization, when compared with NMC samples.
Figure 7 Degradation of NMC, TMC, and BMC scaffolds in vitro.
Notes: Error bars represent mean ± SD for n=3. The difference in degradation among three subgroups is statistically significant (*P<0.05 vs other groups; n=3).
Abbreviations: BMC, biomimetic mineralized collagen; NMC, non-mineralized collagen; TMC, traditional mineralized collagen; SD, standard deviation.

Evaluation of biocompatibility in vitro
The characteristics of 3T3-E1 on the three different scaffolds were evaluated using a laser scanning confocal microscope after being stained by acridine orange/ethidium bromide (). There were no significant dead cells (red staining) on all scaffolds after they were cultured for 7 days. This result indicates that all the three different scaffolds exhibit satisfactory cytocompatibility. However, the preosteoblasts on the NMC scaffold maintained a rounded cell morphology and were lesser in number, whereas they were spindle shaped and plentiful on the mineralized collagen scaffolds (, column IF) under the same conditions. In contrast with the TMC scaffolds, the preosteoblasts on the BMC scaffolds were more spread out, indicating that the BMC scaffolds were more advantageous for the transformation of preosteoblast to osteoblasts.
Figure 8 LSCM images of 3T3-E1 cells seeded on BMC, TMC, and NMC scaffolds after culturing for 12 hours (immunocytochemical staining), 1, 3, 5, and 7 days (AO/EB staining).
Notes: Column “IF” shows the F-actin cytoskeleton (green) and nuclei (blue) of preosteoblasts grown on different collagen scaffolds.
Abbreviations: AO/EB, acridine orange/ethidium bromide; BMC, biomimetic mineralized collagen; NMC, non-mineralized collagen; TMC, traditional mineralized collagen; d, days; LSCM, Laser scanning confocal microscopy.

presents the CCK-8 assay results of the preosteoblasts seeded on the three different scaffolds after 1, 3, 5, and 7 days. Compared with the NMC and TMC scaffolds, the absorbance of BMC scaffolds exhibits a statistically significant difference at certain time intervals (P<0.05), indicating that the BMC scaffold is beneficial to the growth of preosteoblasts.
Figure 9 CCK-8 assay results of 3T3-E1 cells growing in NMC, TMC, and BMC scaffolds after culturing for 1, 3, 5, and 7 days.
Notes: There is a statistically significant difference on cell proliferation between BMC scaffolds and the other two scaffolds at each testing time. The cell proliferation on TMC and NMC scaffolds has no statistical differences at each testing time (*P<0.05; n=3; **P<0.01; n=3; ***P>0.05; n=3).
Abbreviations: BMC, biomimetic mineralized collagen; NMC, non-mineralized collagen; TMC, traditional mineralized collagen; CCK-8, Cell Counting Kit-8.

The ALP activity of preosteoblasts distributing on different scaffolds at 3, 5, and 7 days is shown in . The BMC and TMC scaffolds both presented a significant increase in ALP activity compared with the NMC scaffolds. Although the TMC group exhibited the highest ALP activity at the beginning of the test, the BMC group exhibited clear growth and the highest activity during later testing times. The NMC group always presented the lowest activity during the test phase.
Figure 10 ALP activity of 3T3-E1 cells distributing on NMC, TMC, and BMC scaffolds after culturing for 3, 5, and 7 days.
Notes: The difference between BMC scaffolds and the other two scaffolds is statistically significant at each testing time. The ALP activities on TMC and NMC scaffolds are statistically significant at each testing time (*P<0.05; n=3; **P<0.01; n=3).
Abbreviations: ALP, alkaline phosphatase; BMC, biomimetic mineralized collagen; NMC, non-mineralized collagen; TMC, traditional mineralized collagen.

Results of osteogenic potential in vivo
The diameter of the penetrating defect of the rat skull was 5 mm, as shown in . After 4 weeks of implantation, the micro-CT result showed little mineralization in the blank control group () and only a slight amount of mineralization in the TMC group (). A certain amount of bone regeneration at the edge of the defect was observed in both the TMC and BMC groups (). At 8 weeks, the diameters of the defects in each group had different levels of decrease. Compared with the blank control and NMC groups, the new bone formation in the TMC and BMC groups was more obvious (). The bone regeneration of the BMC group was the most remarkable of all the groups. These results indicate that the BMC scaffolds have a faster bone regeneration rate compared to the other groups.
Figure 11 Skull-penetrating defect model of SD rats.
Notes: (A) Penetrating defect of SD rat; the arrow shows the bone taken from the skull of SD rat. (B) Scaffold implanted in the defect; the arrow shows the collagen scaffold.
Abbreviation: SD, Sprague-Dawley.

Figure 12 Micro-CT images of new bone formation in each group after 4 and 8 weeks.
Notes: The four rows are reconstructed tomograms of rat craniums after 4 weeks (A) and 8 weeks (C) and coronary sections of rat craniums after 4 weeks (B) and 8 weeks (D).
Abbreviations: BMC, biomimetic mineralized collagen; NMC, non-mineralized collagen; TMC, traditional mineralized collagen; CT, computed tomography.

and show the images of histological sections containing H&E and Masson’s trichrome staining. More fibrous connective tissues rather than new bone tissues were observed in the defect of the control group (, ). In contrast, more new bone tissues and osteoid appeared in the bone stump in the BMC ( and ) and TMC ( and ) groups, particularly in the BMC group. Additionally, at the end of the second month, not only new bone tissues but also marrow organ-like structures appeared in the BMC group ( and ).
Figure 13 Histological sections (H&E staining) of rat cranial defect and surrounding tissue at different testing times.
Abbreviations: BMC, biomimetic mineralized collagen; NMC, non-mineralized collagen; TMC, traditional mineralized collagen; H&E, hematoxylin and eosin.

Figure 14 Histological sections (Masson’s trichrome staining) of rat cranial defect and surrounding tissue at different testing times: blank control (A), filled with NMC scaffolds (B), filled with TMC scaffolds (C), and filled with BMC scaffolds (D).
Notes: Histological sections (Masson’s trichrome staining) of rat cranial defect and surrounding tissue at different testing times: blank control (A), filled with NMC scaffolds (B), filled with TMC scaffolds (C), and filled with BMC scaffolds (D). Black arrows, new bone; white arrows, host bone; black stars, scaffold materials; white star, osteoid.
Abbreviations: BMC, biomimetic mineralized collagen; NMC, non-mineralized collagen; TMC, traditional mineralized collagen.

Notes: Blank control (A) NMC scaffolds (B), TMC scaffolds (C), and BMC scaffolds (D). Black arrows, new bone; white arrows, host bone; black stars, scaffold materials; green stars, osteoid.
Discussion
With the progress of testing technology, it has been found that the basic unit of natural bone ECM is mineralized collagen fibrils.Citation14 Therefore, collagen has been widely used as the basic element of scaffolds for bone tissue engineering due to its excellent biocompatibility and biodegradability. However, because collagen possesses poor mechanical properties and too high a degradation rate in vivo when used alone, many studies have attempted to modify it through mineralization.Citation1,Citation44–Citation48 In the present work, we fabricated a novel synergistically intra- and extrafibrillar mineralized collagen scaffold using precursors of ACP stabilized by CMC, a negatively charged polymer. Type 1 collagen self-assembles into fibrils with a triple-helical molecular structure, which is known as tropocollagen, and thus, we can see the distinctive cross-band of 67 nm periods.Citation14 Nevertheless, the sizes of HA crystals are considerably larger than the gap zones of collagen fibrils.Citation49,Citation50 Hence, we provided a new method for biomimetic mineralization based on the PILP strategy, in which collagen gels after self-assembly, but not dry collagen scaffolds, were immersed into the CMC/ACP nanocomplex solution. Compared with the direct mixing of collagen solution and mineral ionsCitation11,Citation46,Citation47 or immersing the dry collagen scaffolds in the mineral solution,Citation13,Citation23,Citation51 the mineralized method described in this study can cause the collagen scaffold to have a water oversaturated status after self-assembly. This status may make the nanocomplexes of CMC/ACP more easily intercalate into the interstices of the collagen fibrils, which is the crux of intrafibrillar mineralization of collagen scaffolds.
Collagen fibrils extracted from the rat tails after self-assembly, similar to the collagen of natural bone, exhibited distinctive cross-band characteristics. The BMC scaffolds acquired using the bionic method described earlier made the cross-band more obvious in a short period of mineralization (24 hours). During this period, most minerals within the collagen scaffolds were in the amorphous state, which was confirmed by the selected-area electron diffraction results, and the diameters of the BMC fibrils increased because the ACP inside and outside the fibrils transform to HA crystals as time passes. The package of HA also made the collagen fibrils harder, and thus, we can observe traces of fractures on the fibrils under SEM (). The diameter of the CMC/ACP nanocomplexes was small enough to make it easy for CMC/ACP nanocomplexes to be deposited inside the gap zones of collagen fibrils, which was confirmed by TEM results. In contrast, due to the large sizes of the crystals generated in the SBF, they were only located on the surface of the fibrils (). During the biomimetic mineralization, collagen fibrils are considered to be the primary template for deposition and orientation of the HA nanocrystals. In addition to collagen templates, noncollagenous proteins, particularly DMP-1, are considered to have a close relationship with the mineralization process. DMP-1 has ACP binding sites at one end and collagen binding sites at the other end such that DMP-1 can stabilize the ACP nanoclusters after self-assembly and guide the nanocomplexes into the gap zone of collagen templates.Citation52–Citation54 In this study, CMC, as the analog of DMP-1, can stabilize ACP over a proper period of time and guide CMC/ACP nanocomplexes into the interior of collagen fibrils. The CMC/ACP nanocomplexes were drawn into the interstices of the collagen fibrils by both the capillary forces of fluidic nature and the interactions between negatively charged nanocomplexes and positive domains in the collagen fibrils (), which have been demonstrated by other research groups.Citation53,Citation55 After a period of time, the nanocomplexes inside the interstices of the collagen fibrils reached saturation levels such that the other nanocomplexes began to be deposited on the surface of the fibrils. As time passes (72 hours), collagen scaffolds accomplished the synergistic intra- and extrafibrillar mineralization via the transformation of ACP to HA. Therefore, the collagen scaffolds fabricated using this biomimetic method can complete synergistic intra- and extrafibrillar mineralization with the assistance of CMC.
Figure 15 The experimental scheme of synergistic intra- and extrafibrillar mineralization process using CMC/ACP nanocomplexes.
Abbreviations: ACP, amorphous calcium phosphate; BMC, biomimetic mineralized collagen; CMC, carboxymethyl chitosan; RT, room temperature; SBF, simulated body fluid; TMC, traditional mineralized collagen.

Rapid degradation and poor mechanical properties are the major disadvantages of collagen as scaffolds for bone tissue regeneration. The degradation rate and mechanical properties of scaffolds may affect the long-term success of bone tissue regeneration because the untimely stresses to the new bone are detrimental for the regeneration of tissues.Citation56,Citation57 The results in this study prove that the mineralization of collagen can protect collagen from degradation and improve its mechanical properties, which is consistent with other results of other studies.Citation9,Citation58,Citation59 To investigate the biological behaviors in vivo of the three different scaffolds, we designed animal experiments and tensile tests in this study. In our animal study, the BMC scaffolds were still visible at the end of 8 weeks after implantation, whereas the NMC scaffolds had almost completely degraded after 4 weeks, which was similar to previous degradation experiments in vitro.Citation9,Citation57 The TMC scaffolds can be observed at the end of 4 weeks. However, they almost dispersed after 8 weeks. Hence, the synergistic intra- and extrafibrillar mineralization can slow the degradation of collagen to a greater degree compared to the extrafibrillar mineralization. The comparison of the elastic modulus among three different scaffolds indicated that the synergistic intra- and extrafibrillar mineralization can improve the mechanical properties of collagen to the maximum extent possible. Compared with the TMC scaffolds, the considerably higher elastic modulus of the BMC scaffolds was primarily attributed to the minerals deposited within the gap zones of the fibrils, similar to natural bone.Citation9,Citation60 Because the scaffolds are under wet conditions in vivo, we tested the mechanical properties of the different scaffolds under wet conditions. All the scaffolds had significant reductions in elastic modulus under wet conditions, which was probably caused by the effect of incorporated water.Citation9 Despite this condition, the BMC scaffolds still possess the best mechanical properties among all the groups.
Sufficient space and a suitable microenvironment are required for the proliferation and differentiation of cells in vitro. The three-dimensional porous collagen scaffolds with appropriate mechanics, porosity, material composition, concentration, and matrix degradation kinetics will allow cells to be engineered to migrate and spread. However, the micropores of collagen scaffolds without mineralization collapse under the basal medium, which was confirmed by the in vitro studies of 3T3-E1. Because of mineralization, the micropores of TMC and BMC can be maintained during the process of cell culture such that they have more spaces for cell proliferation, which is similar to the results of another study.Citation57 We used 3T3-E1 to examine the osteogenic activity of the three different scaffolds in vitro in the present study. The results of cell proliferation () indicated that intra- and extrafibrillar mineralization can protect the micropores from collapsing to a greater degree compared with the extrafibrillar mineralization, and thus, the cell number on the BMC scaffolds was considerably higher than that on the TMC scaffolds. Another reason for the higher cell number on the BMC scaffold may be due to the biomimetic surface topography and excellent mechanical properties of the BMC scaffolds.Citation9 Because ALP is always recognized as one of the most important enzymes produced by osteoblasts, we tested it at different time points as an osteoblastic maker in this study. The level of ALP was obviously higher in the two mineralized groups. This result may be due to the significant effect of mineral HA of osteoblastic differentiation of preosteoblasts (3T3-E1) in vitro.Citation11,Citation61 The ALP activity on the TMC scaffolds was slightly higher than that on the BMC scaffolds at the beginning of the test (). This may be due to the earlier contacts between 3T3-E1 and HA on the TMC scaffolds while the cells were still proliferating on the BMC scaffolds. However, the ALP activity on the BMC scaffolds caught up with and surpassed that on the TMC scaffolds after a period of time. The nanosized HA within the BMC scaffolds may play an important role during this process, which was consistent with the results of other researchers.Citation10,Citation49,Citation62,Citation63 All the results of experiments in vitro indicated that the BMC scaffolds can provide more abundant spaces and a more suitable microenvironment to promote the proliferation and differentiation of 3T3-E1.
The animal experiments were used to evaluate the biological behaviors of the three different collagen scaffolds in vivo. The diameter of defects was selected at 5 mm based on the critical size of defects defined by Schmitz and Hollinger.Citation64 The results of micro-CT of specimens () at each time point (4 and 8 weeks) showed that the new bone formation in BMC group was the most marked among all groups at the macro level, indicating that the synergistically intra- and extrafibrillar mineralized scaffolds had good biological compatibility and osteoconductive effect. To observe the differences among the three scaffolds in vivo at the histological level, H&E and Masson’s trichrome stainings were performed, which confirmed the micro-CT results. At the end of 4 weeks, there was almost no residual collagen scaffolds in the NMC group, whereas a considerably greater amount of material could be observed in the TMC and BMC groups, particularly in the latter group. This result indicated that mineralization can protect collagen scaffolds from degrading, particularly synergistic intra- and extrafibrillar mineralization. Hence, the scaffolds can acquire an appropriate degradation rate adapted to the speed of new bone formation such that it is more beneficial to the regeneration of bone tissue in the defects. Furthermore, the osteoid formation with osteoblasts and the initiation of capillary vessels could be well observed at both 4 and 8 weeks in the BMC group, which showed the potential of osteoinduction of the BMC scaffolds.Citation65 Masson’s trichrome staining of specimens reveals the same results as with H&E. The biomimetic BMC scaffolds fabricated in this study present a satisfactory regeneration effect of bone tissue in defects and an ideal degradation rate in vivo. Because the BMC scaffolds acquired using this strategy have satisfactory results both in vitro and in vivo, it has the potential for clinical application for treating bone defects. Due to the abundant sources and low price of chitosan, the scaffolds can be industrially produced in the near future.
Conclusion
In this study, we fabricated synergistically intra- and extrafibrillar mineralized collagen scaffolds using a new method of biomimetic mineralization in gel form in a CMC/ACP solution based on PILP theory. The results indicated that the BMC scaffold, which was similar to natural bone ECM in terms of chemical composition and microstructure, can better promote the proliferation and differentiation of mouse preosteoblasts and subsequently accelerated the regeneration of bone in the defects of rat calvaria bone compared to the other two groups. Synergistic mineralization can also increase the mechanical strength and decrease the degradation rate of collagen scaffolds at the same time such that the BMC scaffolds can better promote the regeneration of bone tissue in defects.
Acknowledgments
This study was munificently supported by National Natural Science Foundation of China (Grant numbers 31470920 and 81571016). The authors wish to thank Jichao Wang of Beihang University as well as Shanshan Zhang of Hebei University of Technology for their contributions.
Disclosure
The authors report no conflicts of interest in this work.
References
- LiaoSSCuiFZZhangWFengQLHierarchically biomimetic bone scaffold materials: nano-HA/collagen/PLA compositeJ Biomed Mater Res B Appl Biomater200469215816515116405
- NiemeyerPKrauseUFellenbergJEvaluation of mineralized collagen and alpha-tricalcium phosphate as scaffolds for tissue engineering of bone using human mesenchymal stem cellsCells Tissues Organs20041772687815297781
- MeinelLKarageorgiouVFajardoRBone tissue engineering using human mesenchymal stem cellsAnn Biomed Eng200432211212214964727
- WuSLiuXYeungKWKLiuCYangXBiomimetic porous scaffolds for bone tissue engineeringMater Sci Eng R Rep201480136
- StevensMMBiomaterials for bone tissue engineeringMater Today20081151825
- QuinlanEThompsonEMMatsikoAO’BrienFJLopez-NoriegaALong-term controlled delivery of rhBMP-2 from collagen-hydroxyapatite scaffolds for superior bone tissue regenerationJ Control Release201520711211925817394
- Costa-PintoARCorreloVMSolPCOsteogenic differentiation of human bone marrow mesenchymal stem cells seeded on melt based chitosan scaffolds for bone tissue engineering applicationsBiomacromolecules20091082067207319621927
- LiuYMaiSLiNDifferences between top-down and bottom-up approaches in mineralizing thick, partially demineralized collagen scaffoldsActa Biomater2011741742175121111071
- LiuYLuoDLiuSEffect of nanostructure of mineralized collagen scaffolds on their physical properties and osteogenic potentialJ Biomed Nanotechnol20141061049106024749399
- RodriguesSCSalgadoCLSahuAGarciaMPFernandesMHMonteiroFJPreparation and characterization of collagen-nanohydroxyapatite biocomposite scaffolds by cryogelation method for bone tissue engineering applicationsJ Biomed Mater Res A201310141080109423008173
- MinardiSCorradettiBTaraballiFEvaluation of the osteoinductive potential of a bio-inspired scaffold mimicking the osteogenic niche for bone augmentationBiomaterials20156212813726048479
- JiaoKNiuLNLiQHBiphasic silica/apatite co-mineralized collagen scaffolds stimulate osteogenesis and inhibit RANKL-mediated osteoclastogenesisActa Biomater201519233225792280
- AntebiBChengXHarrisJNGowerLBChenXDLingJBiomimetic collagen-hydroxyapatite composite fabricated via a novel perfusion-flow mineralization techniqueTissue Eng Part C Methods201319748749623157544
- ThulaTTRodriguezDELeeMHPendiLPodschunJGowerLBIn vitro mineralization of dense collagen substrates: a biomimetic approach toward the development of bone-graft materialsActa Biomater2011783158316921550424
- FicaiAAndronescuEVoicuGSelf-assembled collagen/hydroxyapatite composite materialsChem Eng J20101602794800
- WangYCuiFZHuKZhuXDFanDDBone regeneration by using scaffold based on mineralized recombinant collagenJ Biomed Mater Res B Appl Biomater2008861293518161820
- GelinskyMWelzelPBSimonPBernhardtAKönigUPorous three-dimensional scaffolds made of mineralised collagen: preparation and properties of a biomimetic nanocomposite material for tissue engineering of boneChem Eng J200813718496
- GomesPSSantosJDFernandesMHCell-induced response by tetracyclines on human bone marrow colonized hydroxyapatite and BonelikeActa Biomater20084363063718291737
- VaniRGirijaEKElayarajaKPrakash ParthibanSKesavamoorthyRNarayana KalkuraSHydrothermal synthesis of porous triphasic hydroxyapatite/(alpha and beta) tricalcium phosphateJ Mater Sci Mater Med200920Suppl 1S43S4818560768
- XieGSunJZhongGLiuCWeiJHydroxyapatite nanoparticles as a controlled-release carrier of BMP-2: absorption and release kinetics in vitroJ Mater Sci Mater Med20102161875188020300953
- ZhouCYeXFanYBiomimetic fabrication of a three-level hierarchical calcium phosphate/collagen/hydroxyapatite scaffold for bone tissue engineeringBiofabrication20146303501324873777
- YokoyamaAGelinskyMKawasakiTBiomimetic porous scaffolds with high elasticity made from mineralized collagen – an animal studyJ Biomed Mater Res B Appl Biomater200575246447216044430
- YangHSLaWGParkJKimCSImGIKimBSEfficient bone regeneration induced by bone morphogenetic protein-2 released from apatite-coated collagen scaffoldsJ Biomater Sci Polym Ed2011231659167121888760
- CuiF-ZLiYGeJSelf-assembly of mineralized collagen compositesMater Sci Eng R Rep2007571–6127
- ThulaTTSvedlundFRodriguezDEPodschunJPendiLGowerLBMimicking the nanostructure of bone: comparison of polymeric process-directing agentsPolymers201131103522328971
- LiuYLiNQiYPIntrafibrillar collagen mineralization produced by biomimetic hierarchical nanoapatite assemblyAdv Mater201123897598021341310
- LiuYKimYKDaiLHierarchical and non-hierarchical mineralisation of collagenBiomaterials20113251291130021040969
- NiederbergerMColfenHOriented attachment and mesocrystals: non-classical crystallization mechanisms based on nanoparticle assemblyPhys Chem Chem Phys20068283271328716835675
- MeldrumFCSearRPNow you see themScience20083321802180319095931
- ZhangSFabrication of novel biomaterials through molecular self-assemblyNat Biotechnol200321101171117814520402
- WongT-SBroughBHoC-MCreation of functional micro/nano systems through top-down and bottom-up approachesMol Cell Biomech200961119382535
- GowerLBOdomDJDeposition of calcium carbonate films by a polymer-induced liquid-precursor (PILP) processJ Cryst Growth20002104719734
- DaiLDouglasEPGowerLBCompositional analysis of a polymer-induced liquid-precursor (PILP) amorphous CaCO3 phaseJ Non-Cryst Solids20083541718451854
- RodriguezDEThula-MataTToroEJMultifunctional role of osteopontin in directing intrafibrillar mineralization of collagen and activation of osteoclastsActa Biomater201410149450724140612
- GeorgeAVeisAPhosphorylated proteins and control over apatite nucleation, crystal growth, and inhibitionChem Rev2008108114670469318831570
- HeGGeorgeADentin matrix protein 1 immobilized on type I collagen fibrils facilitates apatite deposition in vitroJ Biol Chem200427912116491165614699165
- ChenZCaoSWangHBiomimetic remineralization of demineralized dentine using scaffold of CMC/ACP nanocomplexes in an in vitro tooth model of deep cariesPLoS One201510119
- ZhangXLiYSunXBiomimetic remineralization of demineralized enamel with nano-complexes of phosphorylated chitosan and amorphous calcium phosphateJ Mater Sci Mater Med201425122619262825074834
- JeeS-SThulaTTGowerLBDevelopment of bone-like composites via the polymer-induced liquid-precursor (PILP) process. Part 1: influence of polymer molecular weightActa Biomater2010693676368620359554
- OlsztaMJChengXJeeSSBone structure and formation: a new perspectiveMater Sci Eng R Rep2007583–577116
- AndrewCAKhorEHastingsGWThe influence of anionic chitin derivatives on calcium phosphate crystallizationBiomaterials19981914130913169720895
- ShakirMJollyRKhanMSIramNKhanHMNano-hydroxyapatite/chitosan-starch nanocomposite as a novel bone construct: synthesis and in vitro studiesInternational Journal of Biological Macromolecules20158028229226116779
- PricePPreparation and use of rat-tail collagenTCA Manual1975114344
- BurgKJPorterSKellamJFBiomaterial developments for bone tissue engineeringBiomaterials2000212347235911055282
- DuCCuiFZZhuXDde GrootKThree-dimensional nano-HAp/collagen matrix loading with osteogenic cells in organ cultureJ Biomed Mater Res199944440741510397944
- RenXBischoffDWeisgerberDWOsteogenesis on nanoparticulate mineralized collagen scaffolds via autogenous activation of the canonical BMP receptor signaling pathwayBiomaterials20155010711425736501
- BanglmaierRFSanderEAVandeVordPJInduction and quantification of collagen fiber alignment in a three-dimensional hydroxyapatite-collagen composite scaffoldActa Biomater201517263525653215
- MarelliBGhezziCEMohnDAccelerated mineralization of dense collagen-nano bioactive glass hybrid gels increases scaffold stiffness and regulates osteoblastic functionBiomaterials201132348915892621889796
- ZhouHLeeJNanoscale hydroxyapatite particles for bone tissue engineeringActa Biomater2011772769278121440094
- LinKChangJStructure and properties of hydroxyapatite for biomedical applicationsMucaloMHydroxyapatite (Hap) for Biomedical ApplicationsCambridge, UKWoodhead Publishing20151319
- TeoWELiaoSChanCRamakrishnaSFabrication and characterization of hierarchically organized nanoparticle-reinforced nanofibrous composite scaffoldsActa Biomater20117119320220691289
- LandisWJSongMJEarly mineral deposition in calcifying tendon characterized by high voltage electron microscopy and three-dimensional graphic imagingJ Struct Biol199110721161271807348
- LiYThulaTTJeeSBiomimetic mineralization of woven bone-like nanocomposites: role of collagen cross-linksBiomacromolecules2012131495922133238
- HeGGajjeramanSSchultzDSpatially and temporally controlled biomineralization is facilitated by interaction between self-assembled dentin matrix protein 1 and calcium phosphate nuclei in solutionBiochemistry20054449161401614816331974
- NudelmanFPieterseKGeorgeAThe role of collagen in bone apatite formation in the presence of hydroxyapatite nucleation inhibitorsNat Mater20109121004100920972429
- FiniMGianniniSGiardinoRResorbable device for fracture fixation: in vivo degradation and mechanical behaviourInt J Artif Organs199518127727768964644
- LiaoSSCuiFZIn vitro and in vivo degradation of mineralized llagenBased composite scaffold: nanohydroxyapatite/collagen/poly(l-lactide)Tissue Eng200410738015009932
- KimYKGuL-SBryanTEMineralisation of reconstituted collagen using polyvinylphosphonic acid/polyacrylic acid templating matrix protein analogues in the presence of calcium, phosphate and hydroxyl ionsBiomaterials201031256618662720621767
- KimYKMaiSMazzoniABiomimetic remineralization as a progressive dehydration mechanism of collagen matrices–implications in the aging of resin–dentin bondsActa Biomater2010693729373920304110
- GuptaHSSetoJWagermaierWZaslanskyPBoeseckePFratzlPCooperative deformation of mineral and collagen in bone at the nanoscaleProc Natl Acad Sci U S A200610347177411774617095608
- ChuenjitkuntawornBInrungWDamrongsriDMekaapirukKSupapholPPavasantPPolycaprolactone/hydroxyapatite composite scaffolds: preparation, characterization, and in vitro and in vivo biological responses of human primary bone cellsJ Biomed Mater Res A201094124125120166220
- XieXHYuXWZengSXEnhanced osteointegration of orthopaedic implant gradient coating composed of bioactive glass and nanohydroxyapatiteJ Mater Sci Mater Med20102172165217320379763
- LaiGJShalumonKTChenJPResponse of human mesenchymal stem cells to intrafibrillar nanohydroxyapatite content and extrafibrillar nanohydroxyapatite in biomimetic chitosan/silk fibroin/nanohydroxyapatite nanofibrous membrane scaffoldsInt J Nanomedicine20151056758425609962
- SchmitzJPHollingerJOThe critical size defect as an experimental model for craniomandibulofacial nonunionsClin Orthop Relat Res19862052993083084153
- FangDSeoBMLiuYTransplantation of mesenchymal stem cells is an optimal approach for plastic surgeryStem Cells20072541021102817170063