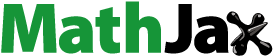
Abstract
This study aimed to evaluate aminoclay (3-aminopropyl-functionalized magnesium phyllosilicate) as an effective protectant for the stabilization of protein formulation in freeze-drying. Bovine serum albumin (BSA), as a model protein, was freeze-dried with aminoclay at various concentrations, and the effects of aminoclay on the structural stability of proteins were compared with those of the conventional stabilizers. The structural characteristics of the protein were determined by size exclusion chromatography (SEC), circular dichroism (CD), and Fourier transform infrared (FTIR) spectroscopy. Furthermore, physicochemical and morphological characteristics were examined by X-ray powder diffraction (XRPD), differential scanning calorimetry (DSC), and scanning electron microscopy (SEM). XRPD and DSC patterns indicated that the glass transition temperature (Tg) of the amorphous formulation of aminoclay mixed with proteins was gradually elevated as the concentration of aminoclay increased. FTIR and CD spectral analysis suggested that the protein structure was well maintained with aminoclay during the freeze-drying process and 3 months of storage at 4°C and 40°C. Furthermore, aminoclay conferred the greatest protection against aggregation and retained the monomer content of BSA even at a high temperature. The morphological characteristics of lyophilized proteins were also well conserved during the storage with aminoclay. These results suggested that aminoclay may be useful as an alternative stabilizer for maintaining the structural stability of protein formulations.
Introduction
Therapeutic proteins have some advantages over small molecular drugs in terms of high specificity and activity as well as low adverse effects at relatively low concentrations. In addition to these benefits, advances in biotechnology and protein engineering have expedited the clinical application of various therapeutic proteins. However, there are still many challenges and issues in the pharmaceutical development of therapeutic proteins. One of the major barriers is the structural alteration of proteins due to the chemical and/or physical instabilities, resulting in activity loss and immunogenicity issues.Citation1–Citation3 Although there have been many attempts to overcome these issues, protein stabilization is still a big challenge in the manufacturing process and storage of protein drugs because of the complexity of protein structures and diverse instability mechanisms.Citation4,Citation5 Therefore, the development of stable formulations that can avoid the biological activity loss and potential immunogenic effect of physically and/or chemically altered protein molecules is critical to promote the commercialization of therapeutic proteins.
In general, as water can facilitate the chemical degradation of proteins, excluding water from the surrounding environment of a protein may improve the chemical stability of the protein structure during storage and various processes.Citation6,Citation7 Therefore, freeze-drying is often used to prepare the solid formulation of pharmaceutical proteins and retain the long-term stability of various proteins.Citation3,Citation8,Citation9 However, water is also critical in maintaining the folding structures of proteins. During folding, nonpolar amino acids are located toward the inner core of the molecule while the residues with polar side chains are predominately located toward the outer surface.Citation10–Citation12 Hydrogen bonds of water with these surface-accessible polar groups significantly contribute to the stabilization of protein structures.Citation10–Citation12 In that sense, freeze-drying processes with water exclusion would often cause the physical instability of proteins with partial unfolding and the formation of protein aggregates. Furthermore, even after successful lyophilization, solid protein formulation may experience a variety of instabilities including aggregation, oxidation, the Maillard reaction, and hydrolysis; thus, its long-term storage stability may still be limited, particularly at high storage temperatures. These instability issues during freeze-drying as well as storage may be minimized by proper selection of pH, residual moisture content, and more importantly, formulation stabilizers such as cryoprotectant and lyoprotectant.Citation3 Various examples of protein instability and stabilization by excipients have been reviewed in detail elsewhere.Citation3
Given that during freeze-drying process, proteins undergo freezing and dehydration, which are fundamentally different stresses, a good protectant should have stabilization effect against both freezing stress (cryoprotectant) and dehydration stress (lyoprotectant).Citation3,Citation13–Citation15 Among various protective agents, nonreducing sugars (eg, sucrose and trehalose) are commonly used cryo- and lyoprotectants upon freeze-drying of protein formulations.Citation11,Citation16 They can improve protein stability by replacing the surrounding water molecules through hydrogen bonding with the proteins during the freeze-drying process and by immobilization of the protein in an amorphous solid cake that could reduce chemical degradation during storage.Citation17–Citation20 In particular, sucrose has been added to many freeze-drying formulations because of its potent structure-stabilizing effect and safety; however, it also has some limitations. For example, sucrose-based amorphous freeze-dried solids have low glass transition temperatures, resulting in stability problems during storage at a higher temperature or higher humidity.Citation21 Furthermore, glycation has been reported in previous studies.Citation22,Citation23 Glycation is the process in which aldehyde groups of reducing sugars interact with the primary and secondary amines of amino acids and form covalent bonds with proteins. Glycation alters the biological activity and the degradation processes of proteins. Khan et alCitation24 reported that glycation of glucose oxidase promoted the formation of genotoxic aggregates. Since sucrose, a nonreducing sugar, can be hydrolyzed into reducing sugars such as glucose and fructose under the low pH of accelerated stress conditions, sucrose-based freeze-dried formulation may potentially undergo glycation, affecting the stability, efficacy, and safety of therapeutic protein formulations.Citation23,Citation25,Citation26 Therefore, efforts should be continued to discover alternative stabilizers for overcoming the limitations of carbohydrates.
3-Aminopropyl-functionalized magnesium phyllosilicate (aminoclay) is well dispersed in water as cationic nanosheets.Citation27 Since the positively charged aminoclay can interact with anionic molecules by electrostatic interactions, aminoclay has been utilized for constructing hybrid materials with biomolecules as well as small organic molecules.Citation27–Citation32 Thermal stability of the protein–aminoclay complex has also been reported in previous studies.Citation27,Citation31 Furthermore, previous in vitro and in vivo studies have suggested that aminoclay should be nontoxic and almost completely eliminated via urine and feces; thereby, the risk of long-term tissue accumulation is low.Citation33,Citation34 Therefore, in this study, the effects of aminoclay on protecting proteins from stresses of the freeze-drying process and ensuring the long-term storage stability of protein formulation were evaluated using bovine serum albumin (BSA) as a model protein. Freeze-dried formulations were prepared with or without aminoclay. The effectiveness of aminoclay in protein stabilization during freeze-drying and storage was examined and compared with conventional stabilizers such as sucrose and trehalose.
Materials and methods
Materials
BSA Fraction V was purchased from Biosesang (Kyung-gi, Korea). Sucrose, d-(+)-trehalose dehydrate, and 3-aminopropyl-triethoxysilane were purchased from Sigma-Aldrich (St Louis, MO, USA). Magnesium chloride hexahydrate and sodium phosphate dibasic anhydrate were obtained from Junsei Chemical Co., Ltd. (Tokyo, Japan). Ethanol was purchased from Merck KGaA (Darmstadt, Germany). All other chemicals were of analytical grade, and all solvents were of HPLC grade.
Synthesis of aminoclay
Aminoclay was obtained as reported in previous studies.Citation27,Citation28 Briefly, 3-aminopropyltriethoxysilane (APTES) (13 mL) was added dropwise with rapid stirring to magnesium chloride hexahydrate (8.4 g) dissolved in ethanol (200 mL). The resulting white slurry was stirred overnight and then centrifuged at 6,000× g for 10 min. The precipitate was washed with ethanol and dried at 40°C. For the exfoliation of aminoclay, the dry powder was dispersed in water and ultrasonicated for 5 min.
Freeze-drying of BSA
All aqueous formulations containing 20 mg/mL of BSA were freeze-dried with/without stabilizing excipients at various concentrations (sucrose, trehalose, and aminoclay). Freeze-drying of the protein solutions was performed using a freeze-drier (LYOPH-PRIDE 10R; Ilshin BioBase Co., Ltd., Korea) as reported previously.Citation35 Samples were loaded onto the shelves at room temperature, and the shelf temperature was gradually reduced to and maintained at −45°C for 2 h. For annealing, the temperature was increased to and maintained at −30°C for 1 h. Following the annealing step, the temperature was ramped to and maintained at −45°C for 2 h. Primary drying was conducted at 57 mTorr with a shelf temperature of −25°C for 60 h. Secondary drying was conducted at a shelf temperature of 40°C for 10 h.
For the evaluation of long-term stability, all freeze-dried samples were stored at different temperatures and relative humidities (RHs) (4°C/60% RH and 40°C/75% RH) for 3 months in sealed glass bottles.
Differential scanning calorimetry (DSC)
DSC measurements were performed using a DSC Q2000 (TA Instruments, Ghent, Belgium). Samples (10–15 mg) were placed into a T-zero aluminum pan sealed by a hermetic lid. All thermograms were obtained at a scanning rate of 2°C/min over a temperature range of 10°C–170°C in the modulated mode (amplitude, 0.75°C at an interval of 30 s) under an inert atmosphere flushed with nitrogen. Transition temperatures were calculated using the Universal Analysis software (Version 4.7A; TA Instruments).
X-ray powder diffraction (XRPD)
XRPD patterns of the freeze-dried powder were determined using an X-ray diffractometer (X’Pert APD; Philips, the Netherlands) with CuKα radiation at 20 mA and 40 kV. Each sample was mounted on an X-ray sample holder and scanned from 3° to 70° (2θ) at a rate of 2°/min with a step size of 0.05°. XRPD experiments were performed at the Korea Basic Science Institute (Daegu, Korea).
Scanning electron microscopy (SEM)
The morphological characteristics of freeze-dried BSA formulations were examined by SEM. Freeze-dried samples were mounted on a specimen stub using double-sided adhesive tape, coated with platinum, and analyzed by a field-emission scanning electron microscope (S-4700; Hitachi, Tokyo, Japan).
Circular dichroism (CD) spectroscopy
CD analysis was performed on the reconstituted protein solutions to examine any change in the secondary structure of proteins. Far UV CD spectra were collected using a Chirascan™-Plus Spectrometer (Applied Photophysics, Surrey, UK). Samples were diluted in 6 mM sodium phosphate buffer (pH 7.4), and BSA concentrations were adjusted to ~0.1 mg/mL. Wavelength spectra were collected from 190 nm to 250 nm at 25°C with a bandwidth of 1 nm and a light path length of 0.5 mm. The reported spectra were expressed as mean residue molar ellipticity (degrees cm2/dmol) as a function of wavelength (nm).
Fourier transform infrared (FTIR) spectroscopy
The secondary structures of freeze-dried BSA samples during storage were assessed by the ATR-FTIR spectroscopy (Nicolet™ iS™ 5; Thermo Fisher Scientific, Waltham, MA, USA) with a ZnSe crystal accessory. FTIR spectra of all samples were obtained over a wavenumber range of 4,000–400 cm−1 with 64 scans at a resolution of 4 cm−1. All spectra were area normalized for comparison among samples.
Size exclusion chromatography (SEC)
Monomer content (% monomer) was analyzed by SEC-HPLC using a Flexar HPLC system (PerkinElmer, Waltham, MA, USA) equipped with a TSKgel G3000SWxl column (300×7.8 mm) (Tosoh Bioscience, King of Prussia, PA, USA). The detection wavelength was 214 nm. All samples were diluted to 1 mg/mL in water and analyzed in isocratic mode with a mobile phase of 0.2 M sodium phosphate buffer (pH 7.0) at a flow rate of 0.7 mL/min.
Results and discussion
Physicochemical and morphological characteristics of freeze-dried BSA
Aminoclay was delaminated in water and underwent spontaneous reassembly by the addition of negatively charged proteins, followed by immediate freeze-drying. The structural characteristics of the obtained freeze-dried solid cakes were examined by XRPD and DSC. Based on XRPD patterns, the interaction mode of BSA with aminoclay was proposed as illustrated in .
The amorphous aminoclay exhibited a basal spacing of d001 =1.5 nm at 2θ =5.84° and broad in-plane reflections at 2θ =22.7°, 35.5°, and 59.4° (). The XRPD pattern of freeze-dried BSA with aminoclay indicated a low angle interlayer d001 reflection similar to that of free aminoclay,Citation31 implying that the electrostatic interaction between BSA and aminoclay may occur at the aminoclay surface rather than the intercalation of proteins into the interlayer space of aminoclay. The large molecular size (14×4×4 nm)Citation36 of BSA may be attributed, at least in part, to produce a disordered matrix of dispersed inorganic sheets where the protein was immobilized. Previous studies also reported that the XRPD patterns of some proteins mixed with aminoclay were dependent on the dimension and surface charge of proteins.Citation31,Citation32 In other words, during exfoliated aminoclay reassembly with the protein by electrostatic interactions, the formation of an ordered lamellar structure would depend on the minimum dimension of the protein.
Figure 2 XRPD patterns (A) and DSC thermograms (B) of BSA and freeze-dried cakes.
Abbreviations: BSA, bovine serum albumin; DSC, differential scanning calorimetry; XRPD, X-ray powder diffraction.

Since thermal stability is generally related to a high glass transition temperature (Tg) of amorphous materials, the thermal stability of BSA would be improved in freeze-dried cakes with excipients. As shown in , the Tg values of the freeze-dried samples with excipients tended to be higher than the Tg value of the excipient-free formulation (). The Tg of BSA was 111°C without excipients, and it was increased to 117°C, 118°C, and 125°C in the presence of sucrose, trehalose, and aminoclay, respectively. In particular, the formation of glass matrix by electrostatic interactions between BSA and aminoclay greatly elevated Tg, which would be critical for producing a stable amorphous solid cake with good redispersibility and good protein stabilization upon storage.Citation37 Therefore, the thermal stability of protein formulations might be improved with aminoclay, which would prevent protein aggregation during storage at high temperatures.
The morphological characteristics of the freeze-dried powder were examined by SEM. Morphology is usually dependent on protein nature, formulation components, and processing conditions.Citation38 As shown in , the morphological characteristics of the freeze-dried formulation with aminoclay were similar to those of freeze-dried formulation with sucrose, and its shape was maintained for 3 months of storage at both 4°C and 40°C.
Structural stability
To assess the effectiveness of aminoclay as a formulation stabilizer, the physicochemical stability of BSA in the freeze-dried cake with or without aminoclay or conventional lyoprotectants was examined. In addition, 3 months storage stability was evaluated at low and high temperatures.
In the stabilization of protein formulations, preventing conformational changes in protein structure is a critical step because the destruction of protein structure can affect protein activity, solubility, and immunogenicity.Citation1–Citation3 Therefore, in this study, the secondary structure of BSA in each formulation was examined by FTIR and CD spectroscopy. Owing to the predominant α-helical region, native BSA has strong absorbance bands at 1,652–1,654 cm−1 in an FTIR spectrumCitation39,Citation40 and strong negative bands at 208 nm and 222 nm in a CD spectrum.Citation41,Citation42 In general, the freeze-drying process can cause a decrease in α-helix; thus, α-helix would be a good indicator of protein structural integrity.Citation43
FTIR spectra
As shown in , FTIR spectra showed the similar patterns in all the tested formulations. The freeze-dried formulation with aminoclay exhibited a typical solid-state amide I spectrum with strong absorbance at 1652–1654 cm−1, thus indicating predominantly α-helical proteins and appeared to be comparable to those from the sucrose- or trehalose-containing formulations. Furthermore, FTIR indicated that the structural stability of BSA in the presence of aminoclay was well conserved during 3 months storage at both 4°C and 40°C ().
Figure 4 FTIR spectra of freeze-dried cakes with/without stabilizing excipients.
Notes: Solid line: at day 0; dotted line: after 3 months storage at 4°C; and dashed line: after 3 months storage at 40°C. (A) BSA; (B) BSA + 1% sucrose; (C) BSA + 1% trehalose; (D) BSA + 1% aminoclay.
Abbreviations: BSA, bovine serum albumin; FTIR, Fourier transform infrared.

CD spectroscopy
CD spectra were also examined to observe any secondary structural changes of freeze-dried protein samples with or without excipients. As shown in , CD spectra displayed distinct characteristics of BSA in all tested conditions. They exhibited similar spectra pattern with strong negative bands at 208 nm and 222 nm, and there was no significant change in the CD spectra after excipient addition. These results suggest that the secondary structure of the protein was not altered following freeze-drying with or without excipients.
Figure 5 CD spectra of freeze-dried cakes with/without stabilizing excipients.
Notes: Solid line: at day 0; dotted line: after 3 months storage at 4°C; and dashed line: after 3 months storage at 40°C. (A) BSA; (B) BSA + 1% sucrose; (C) BSA + 1% trehalose; (D) BSA + 1% aminoclay.
Abbreviations: CD, circular dichroism; BSA, bovine serum albumin.

Taken together, results from FTIR and CD spectral analysis suggest that the structural stability of BSA was well maintained during the freeze-drying process and also during 3 months storage in the presence of aminoclay as a stabilizer.
Effect of aminoclay on BSA aggregation
To examine the extent of BSA aggregation during freeze-drying process, monomer content of BSA in each formulation was determined by SEC analysis. Monomer content (%) was calculated with the following equation:
Monomer content (%) in the excipient-free formulation of BSA was 77.2%±0.12% and 75.6%±0.45% before and after freeze-drying, respectively, indicating that protein aggregation was minimal during the freeze-drying process (). For the formulation containing both aminoclay and conventional protectants such as sucrose and trehalose, the monomer contents in all the tested formulations were similar in the range of 74%–78%. These results indicated that the freeze-drying process did not affect protein aggregation.
Table 1 Monomer content (%) of BSA during 3 months storage at the different temperatures (mean ± SD, n=3)
To examine the extent of aggregation during storage, monomer content (%) was also monitored during 3 months storage at different temperatures. As shown in and , the monomer content in all the tested formulations was well maintained during 3 months storage at 4°C. However, when the freeze-dried powder was exposed to 40°C, the monomer content was significantly (P<0.05) reduced in the excipient free-formulation in which 23.9% of the monomer content was lost during 3 months storage. However, in the presence of excipients (aminoclay, sucrose, or trehalose at 1%), the change in monomer content was minimal during 3 months storage at 40°C. In particular, 1% of aminoclay conferred the greatest protection against aggregation and retained the monomer content of BSA during 3 months storage even at the elevated temperature of 40°C.
Figure 6 Monomer content (%) change of BSA in the freeze-dried cakes with/without stabilizing excipients during 3 months storage at 4°C (A) and 40°C (B).
Abbreviation: BSA, bovine serum albumin.

To examine the effect of aminoclay concentration on protein aggregation, protein formulations were prepared with aminoclay in a concentration range from 0.001% to 1% and their monomer contents were determined after 3 months storage at 4°C and 40°C. Although all the formulations containing 0.001%–1% of aminoclay maintained their monomer content at 4°C during storage, there was a significant change in monomer content at 40°C depending on the concentration of aminoclay. As shown in , after 3 months storage at 40°C, monomer contents were significantly decreased in the formulations containing <1% aminoclay, suggesting that the protective effect against aggregation became weaker as the concentration of aminoclay decreased. Since secondary structural changes were not observed in the protein formulations at all the tested aminoclay concentrations when investigating structural stability (in the “Structural stability” section), the observed monomer content decrease at a lower concentration of aminoclay might result from noncovalent aggregation that did not accompany the conformational changes in protein structures.
In contrast, 1% of aminoclay appeared to be effective in preventing protein aggregation during storage at 40°C and its protective effect was better than that of sucrose and trehalose at the same concentration. Sucrose and trehalose may substitute for water removed from freeze-drying and maintain hydrogen bonds with surface-accessible polar sites on the protein, thus facilitating the preservation of the native-like solid-state protein structure and reducing aggregation during storage.Citation17–Citation20 Alternatively, sucrose and trehalose may improve the structural stability of proteins by embedding the protein within an inert glassy matrix with limited molecular mobility.Citation18–Citation20 On the other hand, aminoclay may undergo a different stabilization mechanism. Aminoclay dispersed in water is cationic and can spontaneously interact with the surface of protein anions by Coulomb forces. As a result, the charged interaction between BSA and aminoclay could form an organic–inorganic hybrid complex that may restrict molecular mobility (). The resulting environment would provide a physical barrier for protein–protein contacts and prevent aggregation. Moreover, a higher concentration of aminoclay may further dilute the protein in the amorphous glassy matrix and provide a physical barrier for protein–protein contacts, leading to slower aggregation. This was also supported by the increased Tg of BSA in the presence of aminoclay, as demonstrated in DSC profiles ().
Taken together, aminoclay may be useful as a stabilizer to minimize protein instability during freeze-drying, thus preventing its aggregation and protecting it from the mechanical stress of ice crystals.
Reducing sugars such as glucose, maltose, and fructose can undergo glycation, which may affect the stability, efficacy, and safety of therapeutic protein formulations.Citation23–Citation26 Even stable nonreducing sugars such as sucrose may be hydrolyzed forming glucose and fructose during freeze-drying under the accelerated stress conditions, raising glycation issues.Citation23,Citation26 Considering that effective protectants should have certain characteristics including a high glass transition temperature, less hygroscopicity, and lack of internal hydrogen bonds,Citation44,Citation45 aminoclay could meet these requirements and its use as a nonsugar-based stabilizer for freeze-drying may overcome certain limitations of sugars. Furthermore, since aminoclay is applicable as a drug delivery carrier,Citation27–Citation32 it can provide some additional benefits. For example, while oral delivery of therapeutic proteins is very limited due to the instability in the gastrointestinal tract, intercalation of proteins into the interlayer spaces of aminoclay may protect the protein from enzymatic degradation and improve the biological activity of proteins.Citation31,Citation32 Therefore, aminoclay may have a dual function in the protein formulations as a carrier and a cryo-/lyoprotectant. Given that in many cases, the use of currently available cryo-/lyoprotectants is insufficient to stabilize proteins completely, the identification of a new protective agent should be beneficial in developing a stable protein formulation.
Conclusion
Various analytical methods indicated that aminoclay was able to retain the structural stability of BSA during the freeze-drying process and storage at an elevated temperature. Furthermore, aminoclay exhibited a greater protective effect on protein aggregation compared with conventional carbohydrates. These results suggest that aminoclay could be useful as an alternative stabilizer for freeze-dried protein formulations.
Acknowledgments
This study was supported by the National Research Foundation of Korea (NRF) grant funded by the Korea government (MSIP) (No 2016R1A2B2010097).
Supplementary material
Disclosure
The authors report no conflicts of interest in this work.
References
- SchellekensHHow to Predict and Prevent the Immunogenicity of therapeutic ProteinsAmsterdam, RadarwegElsevier B.V.2008
- ManningMCPatelKBorchardtRTStability of protein pharmaceuticalsPharm Res19896119039182687836
- WangWLyophilization and development of solid protein pharmaceuticalsInt J Pharm20002031–216010967427
- ChiEYKrishnanSRandolphTWCarpenterJFPhysical stability of proteins in aqueous solution: mechanism and driving forces in nonnative protein aggregationPharm Res20032091325133614567625
- GuptaPHallCKVoeglerACEffect of denaturant and protein concentrations upon protein refolding and aggregation: a simple lattice modelProtein Sci1998712264226529865959
- HillJJShalaevEYZografiGThermodynamic and dynamic factors involved in the stability of native protein structure in amorphous solids in relation to levels of hydrationJ Pharm Sci20059481636166715965985
- TownsJKMoisture content in proteins: its effects and measurementJ Chromatogr A199570511151277620565
- KettVMcMahonDWardKFreeze-drying of protein pharmaceuticals – the application of thermal analysisCryo Letters200425638940415660166
- LimJYKimNALimDGProcess cycle development of freeze drying for therapeutic proteins with stability evaluationJ Pharm Investig2016466519536
- IzutsuKKadoyaSYomotaCKawanishiTYonemochiETeradaKStabilization of protein structure in freeze-dried amorphous organic acid buffer saltsChem Pharm Bull200957111231123619881273
- ArakawaTPrestrelskiSJKenneyWCCarpenterJFFactors affecting short-term and long-term stabilities of proteinsAdv Drug Deliv Rev2001461–330732611259845
- PrestrelskiSJTedeschiNArakawaTCarpenterJFDehydration-induced conformational transitions in proteins and their inhibition by stabilizersBiophys J19936526616717693001
- CroweJHCarpenterJFCroweLMThe role of vitrification in anhydrobiosisAnnu Rev Physiol199860731039558455
- OhtakeSKitaYArakawaTInteractions of formulation excipients with proteins in solution and in the dried stateAdv Drug Deliv Rev201163131053107321756953
- CroweJHCarpenterJFCroweLMAre freezing and dehydration similar stress vectors? A comparison of modes of interaction of stabilizing solutes with biomoleculesCryobiology1990273219231
- CarpenterJFPikalMJChangBSRational design of stable lyophilized protein formulations: some practical advicePharm Res19971489699759279875
- CarpenterJFCroweJHArakawaTComparison of solute-induced protein stabilization in aqueous solution and in the frozen and dried statesJ Dairy Sci1990731236273636
- KreilgaardLFrokjaerSFlinkJMRandolphTWCarpenterJFEffects of additives on the stability of humicola lanuginosa lipase during freeze-drying and storage in the dried solidJ Pharm Sci199988328129010052984
- SampedroJGGuerraGPardoJ-PTrehalose-mediated protection of the plasma Membrane H-ATPase from Kluyveromyces lactis during freeze-drying and rehydrationCryobiology19983721311389769163
- SunWQDavidsonPProtein inactivation in amorphous sucrose and trehalose matrices: effects of phase separation and crystallizationBiochim Biophys Acta1998142512352449813347
- te BooyMPWMde RuiterRAde MeereALJEvaluation of the physical stability of freeze-dried sucrose-containing formulation by differential scanning calorimetryPharm Res1992911091141589394
- LeblancYBihoreauNJubeMAndreMHTellierZChevreuxGGlycation of polyclonal IgGs: effect of sugar excipients during stability studiesEur J Pharm Sci2016102185190
- FischerSHoernschemeyerJMahlerHCGlycation during storage and administration of monoclonal antibody formulationsEur J Pharm Sci20087014250
- KhanTAAmaniSNaeemAGlycation promotes the formation of genotoxic aggregates in glucose oxidaseAmino Acids20124331311132222198547
- SinghRBardenAMoriTBeilinLAdvanced glycation end-products: a reviewDiabetologia200144212914611270668
- BanksDDHamblyDMScavezzeJLSiskaCCStackhouseNLGadgilHSThe effect of sucrose hydrolysis on the stability of protein therapeutics during accelerated formulation studiesJ Pharm Sci200998124501451019388069
- PatilAJMannSSelf-assembly of bio–inorganic nanohybrids using organoclay building blocksJ Mater Chem200818394605
- YangLShaoYHanHKImproved pH-dependent drug release and oral exposure of telmisartan, a poorly soluble drug through the formation of drug–aminoclay complexInt J Pharm20144711–225826324834880
- YangLShaoYHanHKAminoclay–lipid hybrid composite as a novel drug carrier of fenofibrate for the enhancement of drug release and oral absorptionInt J Nanomedicine2016111067107627042061
- PatilAJLiMDujardinEMannSNovel bioinorganic nanostructures based on mesolamellar intercalation or single-molecule wrapping of DNA using organoclay building blocksNano Lett2007792660266517705435
- PatilAJMuthusamyEMannSFabrication of functional protein–organoclay lamellar nanocomposites by biomolecule-induced assembly of exfoliated aminopropyl-functionalized magnesium phyllosilicatesJ Mater Chem20051535–3638383843
- PatilAJMuthusamyEMannSSynthesis and self-assembly of organoclay-wrapped biomoleculesAngew Chem Int Ed2004433749284933
- HanHKLeeYCLeeMYPatilAJShinHJMagnesium and calcium organophyllosilicates: synthesis and in vitro cytotoxicity studyACS Appl Mater Interfaces2011372564257221609130
- YangLLeeY-CKimMIBiodistribution and clearance of aminoclay nanoparticles: implication for in vivo applicability as a tailor-made drug delivery carrierJ Mater Chem B201424375677574
- LewisLMJohnsonREOldroydMECharacterizing the freeze-drying behavior of model protein formulationsAAPS PharmSciTech20101141580159021057905
- KentwrightAThompsonMRHydrodynamic structure of bovine serum albumin determined by transient electric birefringenceBiophys J1975152 pt 11371411167468
- ChatterjeeKShalaevEYSuryanarayananRPartially crystalline systems in lyophilization: II. Withstanding collapse at high primary drying temperatures and impact on protein activity recoveryJ Pharm Sci200594480982015729705
- WangWProtein aggregation and its inhibition in biopharmaceuticsInt J Pharm20052891–213015652195
- AllisonSDDongACarpenterJFCounteracting effects of thiocyanate and sucrose on chymotrypsinogen secondary structure and aggregation during freezing, drying, and rehydrationBiophys J1996714202220328889176
- BylerDMSusiHApplication of computerized infrared and Raman spectroscopy to conformation studies of casein and other food proteinsJ Ind Microbiol199837388
- FarnsworthPNGroth–VasselliBGreenfieldNJEffects of temperature and concentration on bovine lens h-crystallin secondary structure: a circular dichroism spectroscopic studyInt J Biol Macromol19972042832919253648
- SunCYangJWuXHuangXWangFLiuSUnfolding and refolding of bovine serum albumin induced by cetylpyridinium bromideBiophys J20058853518352415731386
- FontePSoaresSSousaFStability study perspective of the effect of freeze-drying using cryoprotectants on the structure of insulin loaded into PLGA nanoparticlesBiomacromolecules201415103753376525180545
- LibrizziFVitranoECordoneLDehydration and crystallization of trehalose and sucrose glasses containing carbonmonoxy-myoglobinBiophys J19997652727273410233087
- CroweLMReidDSCroweJHIs trehalose special for preserving dry biomaterials?Biophys J1996714208720938889183