Abstract
Background. Proton therapy of lung cancer holds the potential for a reduction of the volume of irradiated normal lung tissue. In this work we investigate the robustness of intensity modulated proton therapy (IMPT) plans to motion, and evaluate a geometrical tumour tracking method to compensate for tumour motion. Material and methods. Seven patients with a nine targets with 4DCT scans were selected. IMPT plans were made on the midventilation phase using a 3-field technique. The plans were transferred and calculated on the remaining nine phases of the 4DCT, and the combined dose distribution was summed using deformable image registration (DIR). An additional set of plans were made in which the proton beam was simply geometrically shifted to the centre of the gross tumour volume (GTV), i.e. simulating tracking of the tumour motion but without on-line adjustment of the proton energies. A possible interplay effect between the dynamics of the spot scanning delivery and the tumour motion has not been considered in this work. Results. Around 97–100% of the GTV was covered by 95% of the prescribed dose (V95) for a tumour displacement of less than about 1 cm with a static beam. For the remaining three of nine targets with a larger motion the tracking method studied provided a marked improvement over static beam; raising the GTV V95 from 95 to 100%, 82 to 98% and 51 to 97%, respectively. Conclusion. The possibility of performing DIR and summing the dose on the 4DCT data set was shown to be feasible. The fairly simplistic tracking method suggested here resulted in a marked improvement in GTV coverage for tumours with large intra-fractional motion (>1 cm displacement), indicating that on-line adjustment of the proton energies may be redundant.
Lung cancer was recently shown to be the most common cause of cancer-related death, and responsible for 1.3 million deaths worldwide each year [Citation1,Citation2]. The overall 5-year survival remains poor despite considerable technical advancements in the treatment and detection of lung tumours [Citation3–5]. Recent clinical data shows that increasing the radiation dose to the tumour for early stage and locally advanced cancers improves the local control of the disease [Citation6–8]. But in clinical practice this is difficult to achieve since increasing the radiation dose to the tumour and surrounding normal tissue also increases the risk of treatment-related toxicity [Citation9]. Even very low dose levels were recently shown to be of relevance; the relative lung volume receiving 5 Gy was shown to correlate with the risk of treatment related pneumonitis [Citation10].
A treatment modality that offers the possibility of both increasing the radiation dose to the tumour and reducing the dose to the healthy tissue is proton therapy. The relatively sharp drop in the depth dose curve owing to the well-defined range of protons in tissue can be used effectively in modern treatment planning software, and generally allows for greater sparing of healthy tissue than external photon-beam radiotherapy (XRT) [Citation11,Citation12]. Modern treatment planning software allow for several ‘beam spots’ to be optimised with respect to intensity and energy. The proton beam is then scanned across the target from a given beam direction (i.e. ‘spot-scanning’). The optimal dose-distribution is sought through adjusting a large number of beam spots from a number of beams in order to obtain the optimal (summed) dose-distribution, which will deliver a high dose to the tumour, while minimising the dose to healthy tissue; the process is commonly referred to as Intensity Modulated Proton Therapy (IMPT) planning [Citation13,Citation14].
Proton therapy has been suggested as a treatment modality in a number of cancers [Citation12], and has been demonstrated to be superior to XRT in treatment planning studies for lung cancers in some studies (for instance [Citation15]) but only marginally so in others (for instance [Citation16]). Recent reviews comparing the available clinical outcome for patients treated with state of the art XRT and passively scattered proton therapy on lung cancer were inconclusive [Citation17–19]. Proton therapy has some inherent weaknesses, which could mitigate its successful implementation for lung cancer treatment. Firstly, the well-defined range of protons in tissue causes dose distributions to be sensitive to temporal density variations. Secondly, for the spot-scanning technique, interplay with the scanning- and the breathing frequency might occur [Citation20–23]. Basically, because both the delivery system and the treated volume are subject to change with time the target may move momentarily out of the dose deposition area. For instance, if the scanning and breathing frequencies are similar, significant under- and over-dose may occur in different parts of the target, and the effectiveness of the treatment may be compromised.
To date, there is limited prospective treatment planning data on the potential gain of using advanced techniques for motion management in IMPT [Citation19]; i.e. gating, deep inspiration breath-hold, tumour motion tracking coupled with real-time plan adjustment. Some data has been published on proton therapy and motion management and planning strategies recently [Citation15,Citation24–27]. A method was suggested by Kang et al. [Citation24] where the voxels in the union of all the gross tumour volumes (GTV) for each phase of a 4DCT scan were replaced by a constant Hounsfield value, which proved to yield treatment plans robust to motion. Engelsman et al. [Citation26], optimised proton therapy plans on each individual phase of a 4DCT scan, which was shown to yield a good target coverage. The aim of this study was to investigate the robustness of intensity modulated (spot-scanned) proton therapy (IMPT) with respect to target dose coverage for lung cancer treatment. In addition, we evaluate the possible benefit of performing a simplistic adaptive therapy using real-time geometrical tumour tracking.
Material and methods
This study was based on free breathing 4DCT scans from seven patients referred for stereotactic radiotherapy of peripheral early stage lung cancer. The patients were enrolled in an ongoing prospective study involving implantation of markers into the tumour, repeated 4DCT scans and fluoroscopic movies with the purpose of examining the intra- and interfraction variation in tumour motion.
A Siemens Sensation Open (Siemens Medical Solutions) multi-slice CT scanner was used in a helical mode to acquire the 4DCT raw scan data. The pitch and rotation time for the CT scanner was 0.1 cm and 1 second, respectively. The raw scan data was reconstructed in ten phase bins and with a slice thickness of 3 mm.
Tumours were located peripherally: right lower lobe (3), right middle lobe (1) right upper lobe (2), left upper lobe (2) and left side of the superior mediastinum (1); i.e. totally nine. One of the patients had two tumours in the same lobe and one patients had an unexpected lymph node metastasis in the superior mediastinum, all of which were treated with stereotactic radiotherapy out of protocol. The tumour displacement in the cranio-caudal direction ranged 0.3–2.4 cm and tumour size (diameter) ranged 0.9–6.2 cm. GTV delineation in all phase bins of the 4DCTs was done by the same physician. In the midventilation phase, a GTV to PTV margin of 5 mm in the transversal plane and 10 mm in the cranio-caudal (CC) direction was applied, corresponding to a PTV margin for daily image guidance.
IMPT plans to 45 Gy in three fractions were made using the Eclipse™ version 8.6 treatment planning system (Varian Medical Systems, USA). The radiation absorbed dose was corrected to the radiobiological equivalence of 45 Gy using a cobalt-60 source; often denoted as “cobalt-equivalent Gray” (abbreviated as CGy, GyE or Gy-Eq). The fractionation was identical to that used clinically. A co-planar 3-field technique was used in this study, where the fields were separated by 45 degrees and placed in order to reach the targets through irradiating the healthy tissue to as little extent as possible. The field directions were selected in order to avoid impinging the body surface at an oblique angle. An isocentric field setup was employed, resulting in a focus to skin distance of around 225 cm. The generic beam data of PT2 Varian Proton Therapy System was used in the treatment planning. The machine has a span of proton kinetic energies of 70–250 MeV. Since many of the targets had a proximal depth of less than 4.1 g/cm2, corresponding to the lowest energy available (70 MeV) a range shifter was introduced in the beam. The system included only a 57 mm water equivalent range shifter, which was used for all beams to accommodate placing beam spots at the most proximal part of the target. The range shifter slightly increases the energy straggling and the lateral spread of the beam which results in a blurring of the otherwise very sharp Bragg-peak. This will tend to make the dose fall-off close to the target somewhat less sharp but might also make the plans somewhat more robust to tumour motion. The spot spacing in the scanning direction was set to 0.5 g/cm2 and the spacing between scanning lines was 0.5 cm. The “Simultaneous Spot Optimisation” method was used to optimise the plans. A calculation grid of 2.5 mm was used using the proton convolution superposition calculation algorithm. The planning strategy was similar to that used clinically; i.e. aiming for keeping the dose to the PTV within 95 to 107% of the prescription, and minimising the dose to the lungs and the spinal cord. The average dose to the PTV (on the midventilation phase) was normalised to be equal to the prescription.
The IMPT plans were made on the midventilation phase and then applied and recalculated on each of the remaining phase bins of the 4DCT. One additional set of plans were made where the beam was moved to accommodate for tumour displacement and centred on the delineated GTV of each phase.
The total absorbed dose was summed to the midventilation phase using deformable image registration (VelocityAI™, Velocity Medical Solutions, USA). Various deformable image registration algorithms are contained within the VelocityAI software, including model based segmentation, B-Spline and Demons algorithms all of which have been modified and integrated in an user-friendly graphical interface. The system was used to deform each of the nine phase bins of the 4DCT image sets to the midventilation phase. The dynamic series was composed of the acquired 4D image phase bins (9) and samples the patient respiration at different stages of the respiratory transform containing the information of the moving anatomy. The Velocity system was used to deduce the motion contained in the dynamic image series and match this motion to the static midventilation image through deformable registration. The resulting deformation field produces an approximation of the patient's organ motion during the whole breathing cycle. The Velocity's B-Spline model was chosen due to its simplicity and efficiency in approximating the smooth thoracic motion. The B-Spline model defines the deformation only on a sparse lattice of nodes overlaid on the image, and the displacement at any voxel is obtained by interpolation from closest lattice nodes. One of the main advantages of using the spline model is that the deformation is interpolated between grid points, making it stable to pixel-level noise. Unlike other spline models, the B-Splines are locally controlled. Thus, the displacement of an interpolation point is influenced only by that of the closest grid points and changing a lattice node only affects the transformation regionally, making it efficient in describing local deformations. The specifics of the registration are described in detail in literature [Citation28,Citation29]. The metric over which the optimisation occurs is the Mattes formulation of the mutual information concept [Citation30]. Mattes implementation does not use all voxels in the input images, but rather evaluates a random sample of the voxels and uses interpolation to evaluate the joint histogram, creating histograms that are less noisy if used as criteria for the optimisation. The average and maximum residual error of the deformation was previously shown to be around 1 mm and 2 mm, respectively, for the B-Spline algorithm [Citation28]. The deformation field was subsequently applied to the RT dose matrix, and thereby used to deform each dose matrix calculated by the treatment planning system to the midventilation phase. Following this dose deformation step, the dose available in each voxel was summed to each respective voxel in the registered image sets of the mid-ventilation scan to produce an expected, calculated total absorbed dose.
The summed 4D dose distribution deformed to the midventilation phase was used in the subsequent analysis, where we looked at the PTV and GTV coverage in terms of V95% (the volume receiving 95% of the prescription) and the average dose to both the PTV and GTV. The method used assumes therefore regular breathing and movement of the tumour, equal to the 4DCT. We did not consider the effects related to setup uncertainty and variations in breathing pattern in between fractions in the present study. In addition, the interplay effect of the scanning of the proton beam and the tumour motion was disregarded here but will be the topic of future studies.
Results
In the midventilation (i.e. planning) phase the 95% isodose covered the complete PTV in all cases studied. In , the plans on the midventilation phase were shown.
Figure 1. IMPT plans on targets 1–9 studied in this work on the midventilation phase of a 4DCT study. The doses 10 to 110% of the prescription is displayed in dose colour-wash.
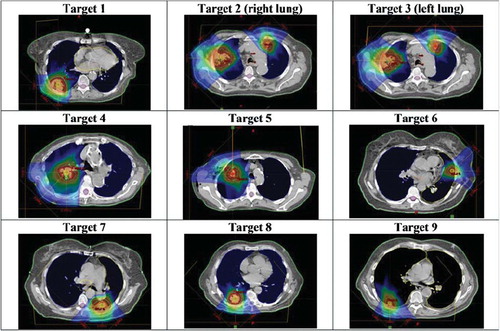
Around 97–100% of the gross tumour volume (GTV) was covered by 95% of the prescribed dose (V95) for a tumour displacement of less than 1 cm; a summary of the data was presented in . GTV V95% and GTV average dose as a function of cranio-caudal (CC) tumour peak-to-peak displacement was shown in . Tumour tracking provided slight deterioration, no or only minor improvement for six of nine targets on the GTV dose coverage. For these targets, typically a few phases of the 4DCT for the static beam plan demonstrated rather poor dose coverage of the GTV, but the summed dose distribution was found to be fairly robust, which stresses the need for 4D treatment planning methods to evaluate dose plans. This effect was shown in , where dose volume histograms for the GTV for target 4 was shown for all phases for the tracked and the static beam plans, respectively.
Figure 2. GTV V95% (left) and GTV average dose (right) as a function of Cranio-Caudal (CC) tumour peak-to-peak displacement for static beam and tracking.
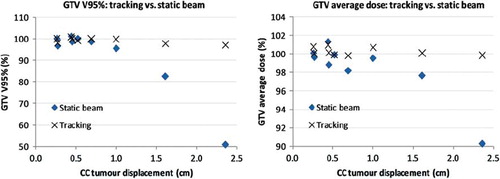
Figure 3. An example of cumulative dose volume histograms for the GTV in each phase of the 4DCT for Target 4 for Tracking (left) and Static beam (right), respectively.
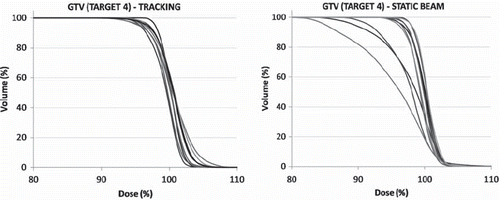
Table I. Patient and planning data for targets 1–9 studied in the present work; Left-Right (LR), Anterior-Posterior (AP) and Cranio-Caudal (CC) peak-to-peak displacement in cm, PTV and GTV V95%, as well as the volume in cm3 of the PTV and the GTV.
Lung doses tended to be similar for tracked and static plans. In , the inspiration phase of the 4DCT was shown as an example with the dose distribution overlaid for the tracked and the static beam, respectively. Large peak doses occurring for the static beam in the lung tissue appears to average out in the summation of all phases.
Figure 4. Example of sagittal plane through target (number 8) with the dose distribution overlaid for tracked beam (left) and static beam (right), respectively. A maximum of 122% of the prescription dose can be observed in the lung tissue for the static beam (right).
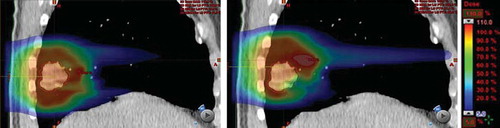
For the tumours with the largest displacement in the CC direction, the tracking technique provided a markedly increase of V95 and of the average dose to the GTV.
Discussion
Radiation pneumonitis occurs for hypo-fractionated radiation therapy of the lung; even though usually at a moderate level (i.e. <10% of patients) [Citation31]. However, higher incidence of radiation pneumonitis (∼25%) have been reported, e.g. Yamashita et al. [Citation32] where 48 Gy in 4 fractions was delivered for most of the patients. The risk of radiation pneumonitis can potentially be reduced using proton therapy.
The 3-field technique employed in the present study was found to result in very good coverage of the GTV and the PTV in the midventilation (i.e. planning) phase, disregarding the impact of the motion on the dose distribution. Taking motion in to account, the GTV dose coverage was still acceptable to the majority of the targets (six out of nine), indicating that motion management strategies for most lung tumours may not be required (assuming the interplay effect can be minimised). For the tumours with equal to or larger displacement than 1 cm peak-to-peak we found a rather substantial drop in both the average dose to the GTV and the volume receiving 95% of the prescription dose (three out of nine targets). For these targets, the simplistic geometrical tracking method suggested here was found to be quite effective. The PTV coverage was also presented in for tracked and static beams. However, considering that the PTV in a few of the cases extended into the chest wall that does not move with the tumour we believe the PTV coverage to be a questionable parameter to use in the evaluation of the tracking method.
The method used for tracking in this study corresponds approximately to steering the beam in two dimensions to accommodate for temporal tumour displacement, however, the method used here might also move the beam slightly in the direction of the beam axis. Given the large source to skin distance and the small movement of the lung tumours in the anterior-posterior and right-left directions makes the movement in the direction of the beam axis negligible. The positional deviation from the midventilation phase was less than half a centimetre in the direction of the beam axis for all phases and patients; the dosimetric difference between the tracking method used here and a purely 2D tracking was therefore estimated to <0.5%.
Interestingly, the geometrical tracking method provided an adequate target coverage for even the patients with a large tumour displacement of about 2 cm, suggesting that on-line tracking as well as adaptation of the proton energies (as previously studied [Citation26]) may not be required. This result was a bit surprising due to the fact that the temporal density changes do not only involve the tumour but also healthy tissue, such as ribs, that does not necessarily move synchronously with the tumour. Such effects occur to some extent but appear to average out, under the assumption of regular breathing and no interplay effect beam scanning vs. tumour motion (which was not studied here).
The importance of the interplay of the beam scanning and tumour motion could be studied using a treatment planning system, such as the system used in this study. This would involve simulating the dynamics of the spot scanning and breathing pattern, which was demonstrated previously by Paganetti et al. [Citation22]. Data on for instance the speed of changing energy and moving spots would be needed for such a study. The delivery sequence could potentially be optimised taking the machine and patient's tumour dynamics in to account in order to minimise the interplay effect. The idea of “repainting” the target, i.e. repeated application of the spot-scanning sequence, was suggested and studied previously, showing that a reduction of the interplay effect was feasible [Citation33]. With current cyclotron- and synchrotron-based technology the repainting using the same energy level is fast. However, changing energy is slower; typically one second, which is practically making real-time adjustments unrealistic. In that sense, the presented method might potentially offer a reasonable technical solution, which we envision would involve only an adjustment of the position of the electromagnetically deflected, scanned proton beam.
An alternative to both the geometrical tracking suggested here and a full 4D plan optimisation and delivery [Citation26] would be to force the treatment planning optimisation to use the same spot grid in all the breathing phases, and then limit the optimisation to adjusting the spot weights. That would then generate ten sets of spots with identical direction and energy information, but each with a different set of weights (i.e. different “intensities”). We postulate that such an approach would improve the plan quality somewhat compared to the method used for tracking in this study, but that needs to be investigated. Technically, the real-time tumour tracking positioning data would then simply direct the beam control software to the appropriate list of spot weights. Adjusting the spot weights in real-time might be easier from an engineering point of view than to steer the beam in real-time, as was implied in this study.
The beam tracking procedure suggested in this study would involve a real-time tracking of the tumour position and correction of the beam position, such as demonstrated for photon therapy [Citation34]. The actual technical solution was not the topic of the present publication; several systems commercially available are capable of providing real-time positional data.
In the present study the largest tumour motion observed in the patient group as a whole was perpendicular to the beam direction. It is possible that a different choice of beam entry angles could affect the plan robustness to intra- and inter-fraction motion to some extent. IMPT for lung cancer might require plan adaptation in order to account for inter-fraction variations; see Sonke and Belderbos for a recent review for XRT [Citation35]. Further studies are required to clarify if IMPT using the tracking method proposed here compares favourably to other methods previously shown to be effective [Citation24,Citation26,Citation27].
Conclusion
In this study we have studied the effect of intra-fraction motion in silico for small lung tumours treated with stereotactic radiotherapy at our institution. We demonstrate that a good target coverage can be achieved by planning on the midventilation phase of a 4DCT scan using intensity modulated proton therapy for targets exhibiting a relatively modest motion, i.e. less than around 1 cm peak-to-peak displacement, and assuming that the interplay of the beam spot-scanning and patient motion can be minimised. For targets with a peak-to-peak displacement of 1 cm or larger we find that a real-time geometrical tracking of the proton beam could substantially improve the target coverage. The summed dose distributions for the plans using the geometrical tracking method were on par in terms of quality with the plans optimised on the midventilation phase. Further, we demonstrate that four-dimensional treatment planning is a useful tool in the selection and exploration of motion management strategies in proton therapy.
Acknowledgements
Mr. Thomas Carlslund and Mr. Mikael Olsen are acknowledged for technical support. Mr. Richard diMonda (Velocity Medical Systems, USA) and Dr. Tim Fox (Emory University Hospital, Atlanta, USA) are acknowledged for valuable discussions. Stine Korreman and Ditte E. Nygaard thank the Lundbeck foundation for financial support.
Declaration of interest: Per Munck af Rosenschöld, Svend Aage Engelholm and Stine Korreman have research collaboration agreements with Varian Medical Systems, USA. Marianne Aznar has a research collaboration agreement with Velocity Medical Systems, USA.
References
- WHO (February 2006). “Cancer”. Lyon: World Health Organization; 2010.
- Jemal A, Siegel R, Ward E, Hao Y, Xu J, Thun MJ. Cancer statistics, 2009. CA Cancer J Clin 2009;59:225–49.
- Ferlay J, Autier P, Boniol M, Heanue M, Colombet M, Boyle P. Estimates of the cancer incidence and mortality in Europe in 2006. Ann Oncol 2007;18:581–92.
- Rowell NP, Williams CJ. Radical radiotherapy for stage I/II non-small cell lung cancer in patients not sufficiently fit for or declining surgery (medically inoperable): A systematic review. Thorax 2001;56:628–38.
- van Rens MT, de la Riviere AB, Elbers HR, van den Bosch JM. Prognostic assessment of 2,361 patients who underwent pulmonary resection for non-small cell lung cancer, stage I, II, and IIIA. Chest 2000;117:374–9.
- Belderbos JS, Heemsbergen WD, De JK, Baas P, Lebesque JV. Final results of a Phase I/II dose escalation trial in non-small-cell lung cancer using three-dimensional conformal radiotherapy. Int J Radiat Oncol Biol Phys 2006;66: 126–34.
- Dosoretz DE, Katin MJ, Blitzer PH, Rubenstein JH, Galmarini DH, Garton GR, . Medically inoperable lung carcinoma: The role of radiation therapy. Semin Radiat Oncol 1996;6:98–104.
- Sibley GS, Jamieson TA, Marks LB, Anscher MS, Prosnitz LR. Radiotherapy alone for medically inoperable stage I non-small-cell lung cancer: The Duke experience. Int J Radiat Oncol Biol Phys 1998;40:149–54.
- Bradley J, Graham MV, Winter K, Purdy JA, Komaki R, Roa WH, . Toxicity and outcome results of RTOG 9311: A phase I-II dose-escalation study using three-dimensional conformal radiotherapy in patients with inoperable non-small-cell lung carcinoma. Int J Radiat Oncol Biol Phys 2005;61: 318–28.
- Wang S, Liao Z, Wei X, Liu HH, Tucker SL, Hu CS, . Analysis of clinical and dosimetric factors associated with treatment-related pneumonitis (TRP) in patients with non-small-cell lung cancer (NSCLC) treated with concurrent chemotherapy and three-dimensional conformal radiotherapy (3D-CRT). Int J Radiat Oncol Biol Phys 2006;66:1399–407.
- Lomax AJ. Charged particle therapy: The physics of interaction. Cancer J 2009;15:285–91.
- Turesson I, Johansson KA, Mattsson S. The potential of proton and light ion beams in radiotherapy. Acta Oncol 2003;42:107–14.
- Lomax A. Intensity modulation methods for proton radiotherapy. Phys Med Biol 1999;44:185–205.
- Zurlo A, Lomax A, Hoess A, Bortfeld T, Russo M, Goitein G, . The role of proton therapy in the treatment of large irradiation volumes: A comparative planning study of pancreatic and biliary tumors. Int J Radiat Oncol Biol Phys 2000;48:277–88.
- Chang JY, Zhang X, Wang X, Kang Y, Riley B, Bilton S, . Significant reduction of normal tissue dose by proton radiotherapy compared with three-dimensional conformal or intensity-modulated radiation therapy in Stage I or Stage III non-small-cell lung cancer. Int J Radiat Oncol Biol Phys 2006;65:1087–96.
- Georg D, Hillbrand M, Stock M, Dieckmann K, Potter R. Can protons improve SBRT for lung lesions? Dosimetric considerations. Radiother Oncol 2008;88:368–75.
- Grutters JP, Kessels AG, Pijls-Johannesma M, De RD, Joore MA, Lambin P. Comparison of the effectiveness of radiotherapy with photons, protons and carbon-ions for non-small cell lung cancer: A meta-analysis. Radiother Oncol 2010;95:32–40.
- Pijls-Johannesma M, Grutters JP, Lambin P, Ruysscher DD. Particle therapy in lung cancer: Where do we stand? Cancer Treat Rev 2008;34:259–67.
- Widesott L, Amichetti M, Schwarz M. Proton therapy in lung cancer: Clinical outcomes and technical issues. A systematic review. Radiother Oncol 2008;86:154–64.
- van de WS, Kreuger R, Zenklusen S, Hug E, Lomax AJ. Tumour tracking with scanned proton beams: Assessing the accuracy and practicalities. Phys Med Biol 2009;54: 6549–63.
- Seco J, Robertson D, Trofimov A, Paganetti H. Breathing interplay effects during proton beam scanning: Simulation and statistical analysis. Phys Med Biol 2009;54: N283–N294.
- Paganetti H, Jiang H, Trofimov A. 4D Monte Carlo simulation of proton beam scanning: Modelling of variations in time and space to study the interplay between scanning pattern and time-dependent patient geometry. Phys Med Biol 2005;50:983–90.
- Lomax AJ. Intensity modulated proton therapy and its sensitivity to treatment uncertainties 2: The potential effects of inter-fraction and inter-field motions. Phys Med Biol 2008;53:1043–56.
- Kang Y, Zhang X, Chang JY, Wang H, Wei X, Liao Z, . 4D Proton treatment planning strategy for mobile lung tumors. Int J Radiat Oncol Biol Phys 2007;67:906–14.
- Hui Z, Zhang X, Starkschall G, Li Y, Mohan R, Komaki R, . Effects of interfractional motion and anatomic changes on proton therapy dose distribution in lung cancer. Int J Radiat Oncol Biol Phys 2008;72:1385–95.
- Engelsman M, Rietzel E, Kooy HM. Four-dimensional proton treatment planning for lung tumors. Int J Radiat Oncol Biol Phys 2006;64:1589–95.
- Zhao L, Sandison GA, Farr JB, Hsi WC, Li XA. Dosimetric impact of intrafraction motion for compensator-based proton therapy of lung cancer. Phys Med Biol 2008;53: 3343–64.
- Lawson JD, Schreibmann E, Jani AB, Fox T. Quantitative evaluation of a cone-beam computed tomography-planning computed tomography deformable image registration method for adaptive radiation therapy. J Appl Clin Med Phys 2007;8:96–113.
- Schreibmann E, Thorndyke B, Li T, Wang J, Xing L. Four-dimensional image registration for image-guided radiotherapy. Int J Radiat Oncol Biol Phys 2008;71:578–86.
- Mattes D, Haynor DR, Vesselle H, Lewellen TK, Eubank W. PET-CT image registration in the chest using free-form deformations. IEEE Trans Med Imaging 2003;22:120–8.
- Marks LB, Bentzen SM, Deasy JO, Kong FM, Bradley JD, Vogelius IS, . Radiation dose-volume effects in the lung. Int J Radiat Oncol Biol Phys 2010;76:S70–S76.
- Yamashita H, Nakagawa K, Nakamura N, Koyanagi H, Tago M, Igaki H, . Exceptionally high incidence of symptomatic grade 2-5 radiation pneumonitis after stereotactic radiation therapy for lung tumors. Radiat Oncol 2007;2:1–11.
- Lambert J, Suchowerska N, McKenzie DR, Jackson M. Intrafractional motion during proton beam scanning. Phys Med Biol 2005;50:4853–62.
- Falk M, Munck af Rosenschold P, Keall P, Cattell H, Cho BC, Poulsen P, . Real-time dynamic MLC tracking for inversely optimized arc radiotherapy. Radiother Oncol 2010;94:218–23.
- Sonke JJ, Belderbos J. Adaptive radiotherapy for lung cancer. Semin Radiat Oncol 2010;20:94–106.