Abstract
Stress is the leading psychopathological cause for several mental disorders. Physiological and psychological responses to stress are mediated by the hypothalamic–pituitary–adrenal (HPA), sympathoadrenal system (SAS), and brain monoaminergic systems (BMS). Eugenol is reported to substantially modulate brain functions by regulating voltage-gated cation channels and release of neurotransmitters. This study was designed to evaluate the anti-stress effect of eugenol in the 4-h restraint model using rats. Ulcer index was measured as a parameter of the stress response. HPA axis and the SAS were monitored by estimating plasma corticosterone and norepinephrine (NE), respectively. Analysis of NE, serotonin (5-HT), dopamine, and their metabolites in discrete brain regions was performed to understand the role of BMS in the anti-stress effect of eugenol. Stress exposure increased the ulcer index as well as plasma corticosterone and NE levels. Eugenol pretreatment for 7 days decreased the stress-induced increase in ulcer index and plasma corticosterone but not NE levels, indicating a preferential effect on the HPA axis. Furthermore, eugenol showed a “U”-shaped dose–response curve in decreasing ulcer index and plasma corticosterone levels. Eugenol also reversed the stress-induced changes in 5-HT levels in all brain regions, whereas NE levels were reversed in all brain regions except hippocampus. These results suggest that eugenol possesses significant anti-stress activity in the 4-h restraint model and the effect is due to modulation of HPA and BMS.
Introduction
Stress is the major cause of several psychiatric and metabolic disorders. Stress is involved in the psychopathology of mental disorders such as schizophrenia, depression, and addiction at all levels ranging from development and maintenance to relapse of disorders (Marinelli and Piazza Citation2002; Charney Citation2004; Hasler et al. Citation2004; Brady and Sinha Citation2005). Stress can be defined as a non-specific response of the body to the demands (usually noxious) imposed on it (Selye Citation1936). The body responds to stress by the way of allostasis in which there is continuous effort to maintain physiological functions within a certain range, which is variable to demand. However, too much stress or inefficient management of allostasis leads to allostatic load, leading to the development of mental disorders (McEwen Citation2000).
The two major effector systems, which serve to maintain homeostasis, are the hypothalamic–pituitary–adrenal (HPA) axis and the autonomic nervous system. Activation of the HPA axis leads to a cascade of events culminating in the secretion of steroids, while the autonomic response is responsible for fight-or-flight responses. The pathological conditions of stress are observed as a result of alterations in the above psychological homeostatic processes (Burchfield Citation1979). The psychopathological consequences of stress have been linked to the overactivation of the body's normal behavioral and emotional systems (Hennessy and Levine Citation1979; Pfaff Citation2006). The most well-studied and pronounced interaction of the central nervous system (CNS) and HPA axis is the bidirectional regulation of corticotrophin-releasing hormone (CRH) and norepinephrine (NE) systems. The feedforward system consisting of CRH and NE acts at different levels of the CNS and promotes the activation of each other (Koob Citation1999). This feedforward cycle is hypothesized to orchestrate the biological response of an organism to environmental challenge. Any derangement in its function would lead to the collapse of the stress response and increase the vulnerability to stress disorders (Koob Citation1999).
Glucocorticoid (GR) and mineralocorticoid receptors are widely distributed in different regions of brain which regulate the HPA axis, i.e. hippocampus (HIP), amygdala (AMY), and prefrontal cortex (PFC). Paraventricular nucleus (PVN) through GRs is believed to be involved in the negative feedback regulation of the HPA axis at higher concentrations of corticosteroids (Phillips et al. Citation2006). Apart from being negatively regulated by GR in the PVN (Phillips et al. Citation2006), the HPA axis can also be influenced by higher brain regions such as the HIP, AMY, and PFC (Feldman and Weidenfeld Citation1995; Herman and Cullinan Citation1997; Herman et al. Citation2005). Several studies have shown that the HPA axis is negatively modulated by HIP (Sapolsky et al. Citation1986; Jacobson and Sapolsky Citation1991; Herman and Cullinan Citation1997). The AMY regulates stress-related GR secretion (Jankord and Herman Citation2008) by activating the HPA axis in response to either a physical or psychological stressor (Herman et al. Citation2005). PFC has a dual action depending on the hemisphere activated (Czéh et al. Citation2008). Each of the above brain regions are interconnected with each other. There is evidence to show that AMY has an inhibitory effect on HIP (Akirav and Richter-Levin Citation1999). Furthermore, PFC is reported to inhibit long-term potentiation associated with AMY activation (Maroun and Richter-Levin Citation2003). HIP through afferent neuronal projections modulates functions of PFC (Floresco and Grace Citation2003). These brain regions, apart from being interconnected with each other, also communicate with the PVN of the hypothalamus (HYPO) through various neurotransmitters (Herman et al. Citation2005). Among the neurotransmitters, NE, 5-HT, and dopamine (DA) are the important monoamines which are widely distributed in brain, and their functional role is well established during stressful conditions. Changes in these neurotransmitter activities lead to alteration in HPA axis homeostasis, leading to development of various psychological and physiological disorders (Sheikh et al. Citation2007). Thus, in stress, there is a complex interaction of different brain regions as part of the homeostatic response. Therefore, therapies targeting central stress mechanisms are fundamental for the development of successful treatment strategies.
Eugenol is an essential oil abundantly found in clove oil, nutmeg, cinnamon, and bay leaf. Eugenol is commonly being used as an analgesic, local anesthetic, and anti-inflammatory agent (Varel and Miller Citation2004). It has also been reported to have several effects on the CNS, where eugenol protected neuronal cells against excitotoxicity induced by ischemia and amyloid-β peptide (Wie et al. Citation1997; Won et al. Citation1998; Irie and Keung Citation2003; Ardjmand et al. Citation2006). It prevented acute neural swelling and reduced neural death due to oxygen–glucose deprivation (Wie et al. Citation1997). Furthermore, eugenol showed neuroprotective effects in hippocampal tissue due to its ability to induce brain-derived neurotrophic factor (Irie et al. Citation2004). Eugenol has been reported to inhibit monoamine oxidase-A (MAO-A) activity and MAO-B at higher concentrations (Tao et al. Citation2005). Eugenol interfered with rat phrenic nerve transmission due to membrane-stabilizing (local anesthetic) effect at low concentrations (Brodin and Roed Citation1984). Experimental data on rodents suggest that eugenol has anxiolytic (Yazaki Citation1989), anti-epileptic (Müller et al. Citation2006), and anti-depression-like (Irie et al. Citation2004) activities. Anti-stress effects of eugenol, by reducing stress-induced increases in protein cauterization and fluidity of synaptosomal membranes, have been reported (Sen et al. Citation1992). However, a detailed study on stress pathways would be useful in understanding the mechanism of action and the rationalization of the use of eugenol as an anti-stress agent.
This study was designed to evaluate the anti-stress effect of eugenol in the 4-h restraint model. The two important modulators of stress, namely the HPA axis and the sympathoadrenal system SAS, were monitored by estimating plasma corticosterone and NE, respectively. Furthermore, to understand the role of brain monoaminergic systems (BMS) in the anti-stress effect of eugenol, analysis of NE, 5-HT, DA, and their metabolites was performed in discrete brain regions such as HIP, HYPO, PFC, and AMY.
Materials and methods
Animals
All experiments were conducted in accordance with the principles of laboratory animal care (NIH publication number 85-23, revised 1985) guidelines. Male, adult, Charles Foster strain albino rats, about 3 months of age (200 ± 20 g), were purchased from the Central Animal House, Institute of Medical Sciences, Banaras Hindu University. The animals were housed in polypropylene cages under controlled environmental conditions of temperature of 25 ± 1°C and 45–55% relative humidity and a 12:12 h light–dark cycle. The experimental animals had free access to commercial rat feed (Doodh Dhara Pashu Ahar, India) and water ad libitum during the experiment. Animals were acclimatized for at least 1 week before using them for experiments and were exposed only once to every experiment.
Drugs
Eugenol was gifted by Prime Dental Pvt. Ltd., Mumbai. It was dissolved in 40% propylene glycol in phosphate buffer (pH 6.8). All other chemicals and reagents of high-performance liquid chromatography (HPLC) and analytical grade were procured from local suppliers.
Restraint stress model
After 18 h fasting, one stress session consisting of a 4-h restraint period (5 × 5 × 20 cm restrainer cages) at room temperature was performed during the early phase of the light cycle (Shah et al. Citation2004).
Experimental protocol
All the animals were divided into six groups. Eugenol was administered by oral gavage using a ball-ended feeding needle, to three different groups (25, 50, and 100 mg/kg) for 7 consecutive days. On day 7, the animals were restrained after 1 h following administration of the last dose. The group receiving vehicle without stress exposure served as the control group, while the group receiving vehicle with stress exposure served as sham. After 4 h of stress, all the animals were killed followed by microdissection and estimation of ulcer index.
Estimation of ulcer index
The stomach was cut through its greater curvature and the ulcer index was calculated following a standard protocol by an observer blind to the treatment group (Sairam et al. Citation2003).
Estimation of plasma corticosterone
Plasma corticosterone levels were quantified by HPLC coupled to an ultraviolet (UV) detector (Waters, Milford, MA, USA), according to the method of Woodward and Emery (Citation1987), with minor modifications, using dexamethasone as an internal standard. Briefly, 500 μL of plasma containing a known quantity of dexamethasone was extracted with 5 mL of dichloromethane. The dichloromethane extract was evaporated to dryness and dissolved in 100 μL of mobile phase. Twenty microliters of the extract were injected into the HPLC system for quantification. Mobile phase consisted of methanol: water (70:30) at a flow rate of 1.2 ml/min and corticosterone was detected at 250 nm using a UV detector (Model 2487, Waters). The chromatogram was recorded and analyzed with Breeze software (Version 3.2).
Estimation of plasma NE
The plasma NE was quantified by using an HPLC coupled to an electrochemical detector (ECD) system (Waters; Sastre et al. Citation2004). Blood samples were collected in heparinized tubes by retro-orbital puncture and were centrifuged for 10 min at 1547 g (Biofuge Stratos, Heaureas, Germany) at 10°C. They were separated in two aliquots and frozen at − 70°C before analysis. Five hundred microliters of plasma sample were first washed by hexane to remove lipids. Proteins were precipitated with sulfosalicylic acid (10 g/100 ml), and 0.1 ml of internal standard 3, 4-dihydroxybenzylamine was added. After centrifugation, the supernatant was washed with ethylacetate saturated by sodium chloride. The ethylacetate phase containing NE was evaporated to dryness at 37°C under a stream of dry nitrogen and frozen at − 24°C until analysis. For NE analysis, the residue was reconstituted in 0.1 ml of mobile phase and 20 μL was injected via HPLC pump (Model 1525, Binary Gradient Pump, Waters) into a column (Spherisorb, RP C18, 5-μm particle size, 4.6 mm i.d. × 250 mm at 30°C) connected to an ECD (Model 2465, Waters) at a potential of +0.8 V with glassy carbon working electrode vs. Ag/AgCl reference electrode. The mobile phase consists of 0.1 M sodium acetate, 0.02 M citric acid, 0.4 mM sodium octyl sulfonate, and 0.2 mM EDTA Na2. The pH of the buffer running solution was adjusted to 4.92 and then filtered through a 0.45-μm filter (Millipore, Bedford, MA, USA). Methanol was added to give a final composition of 4.5% methanol (v/v). A flow rate of 0.8 ml/min was used. The chromatogram was recorded and analyzed with Breeze software (Version 3.2).
Estimation of NE, serotonin, DA, and their metabolites
After decapitation, the brains were removed and microdissected according to Palkovits and Brownstein, (Citation1988) on glass plates over ice into four regions: the total HIP, total HYPO, PFC, and AMY. The levels of NE, 5-HT, DA, and their metabolites were estimated from the isolated tissues by using an HPLC–ECD system, as described by Kim et al. (Citation1987). In brief, the brain tissue samples were homogenized in 0.17 M perchloric acid by Polytron homogenizer. Homogenates were then centrifuged at 33,000 g (Biofuge Stratos) at 4°C. Twenty microliters of the supernatant were injected via the HPLC pump (Model 1525, Binary Gradient Pump) into a column (Spherisorb, RP C18, 5 μm particle size, 4.6 mm i.d. × 250 mm at 30°C) connected to an ECD (Model 2465) at a potential of +0.8 V with glassy carbon working electrode vs. Ag/AgCl reference electrode. The mobile phase consisted of 32 mM citric acid, 12.5 mM disodium hydrogen orthophosphate, 1.4 mM sodium octyl sulfonate, 0.05 mM EDTA, and 16% (v/v) methanol (pH 4.2) at a flow rate of 1.2 ml/min. The protein content was estimated using the method of Lowry et al. (Citation1951).
Statistical analysis
The results are expressed as mean ± SEM. The data were analyzed with GraphPad Prism 4 (San Diego, CA, USA). Statistical significance was determined by one-way analysis of variance (ANOVA) followed by post hoc Student–Newman–Keuls test. P < 0.05 was considered to be statistically significant.
Results
Effect of eugenol on stress-induced gastric ulcers
illustrates the effect of eugenol pretreatment (25, 50, and 100 mg/kg) on ulcer index. One-way ANOVA revealed that there was significant difference in ulcer index among groups [F (4, 20) = 17.20, P < 0.05]. Post hoc analysis indicated a significant increase in ulcer index in sham compared to control that in animals. Eugenol pretreatment significantly decreased the restraint stress (RS)-induced increase in ulcer index in all the doses tested. Interestingly, the anti-stress effect in terms of reduction in ulcer index of eugenol exhibited a “U”-shaped dose–response relationship.
Figure 1. Bar diagram representing the RS-induced changes in ulcer index of control, sham, and eugenol-treated [25 mg/kg (E-25), 50 mg/kg (E-50), and 100 mg/kg (E-100)] groups. Results are represented as mean ± SEM with n = 6 in each group. “n” is the number of rats used. *P < 0.05 compared to control, †P < 0.05 compared to sham, P < 0.05 compared to E-25, and P < 0.05 compared to E-50 (Student–Newmann–Keuls post hoc test).
![Figure 1. Bar diagram representing the RS-induced changes in ulcer index of control, sham, and eugenol-treated [25 mg/kg (E-25), 50 mg/kg (E-50), and 100 mg/kg (E-100)] groups. Results are represented as mean ± SEM with n = 6 in each group. “n” is the number of rats used. *P < 0.05 compared to control, †P < 0.05 compared to sham, P < 0.05 compared to E-25, and P < 0.05 compared to E-50 (Student–Newmann–Keuls post hoc test).](/cms/asset/cac06c44-bed7-44bc-a313-51d577a7d2f1/ists_a_521602_f0001_b.gif)
Eugenol reverses stress-induced increase in plasma corticosterone levels
The effect of eugenol pretreatment (25, 50, and 100 mg/kg) on plasma corticosterone levels is shown in . Statistical analysis by one-way ANOVA showed a significant interaction between vehicle and drug-treated groups [F (4, 20) = 41.54, P < 0.05]. Post hoc analysis revealed that stress significantly increased plasma corticosterone levels. Eugenol pretreatment at 50 and 100 mg/kg but not 25 mg/kg significantly reduced the stress-induced increase in plasma corticosterone levels. However, plasma corticosterone levels were significantly higher in rats treated with eugenol at a dose of 100 mg/kg than in rats treated with eugenol 50 mg/kg. Thus, eugenol shows a U-shaped curve in inhibiting stress response in terms of plasma corticosterone levels.
Figure 2. Bar diagram representing the RS-induced changes in plasma corticosterone (A) and NE levels (B) of control, sham, and eugenol-treated groups. Results are represented as mean ± SEM with n = 6 in each group. “n” is the number of rats used. *P < 0.05 compared to control, †P < 0.05 compared to sham, ‡P < 0.05 compared to E-25, ¶P < 0.05 compared to E-50, and P < 0.05 compared to E-100 (Student–Newmann–Keuls post hoc test).
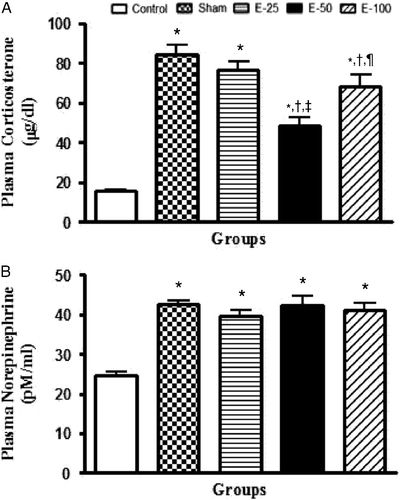
Eugenol does not modulate stress-induced increase in plasma NE levels
shows the graphical representation of the effect of eugenol on plasma NE levels. One-way ANOVA revealed that there was a significant difference in plasma NE levels between groups [F (4, 20) = 22.18, P < 0.05]. Post hoc analysis indicated that RS significantly increased plasma NE levels. Eugenol pretreatment was not able to mitigate the effect of stress on plasma NE levels.
Effect of eugenol on brain NE levels
The effect of eugenol on NE levels in HIP, HYPO, PFC, and AMY is shown in . One-way ANOVA indicated a significant interaction in NE levels among groups in HIP [F (2, 12) = 5.695, P < 0.05], HYPO [F (2, 12) = 7.966, P < 0.05], PFC [F (2, 12) = 9.286, P < 0.05], and AMY [F (2, 12) = 18.76, P < 0.05]. Post hoc analysis indicated that stress exposure significantly increased NE levels in all the brain regions. Pretreatment with eugenol 50 mg/kg for 7 days decreased the stress-induced increase in NE levels in HYPO, PFC, and AMY but not in HIP. Furthermore, eugenol pretreatment decreased NE levels near to control values in HYPO and PFC but not in AMY.
Figure 3. Bar diagram representing the RS-induced changes in the levels of NE (A), 5-HT (B), and 5-HIAA (C) of control, sham, and eugenol treated (50 mg/kg) groups in HIP, HYPO, PFC, and AMY regions of brain. Results are represented as mean ± SEM with n = 6 in each group. “n” is the number of rats used. *P < 0.05 compared to control and †P < 0.05 compared to sham group (Student–Newmann–Keuls post hoc test).
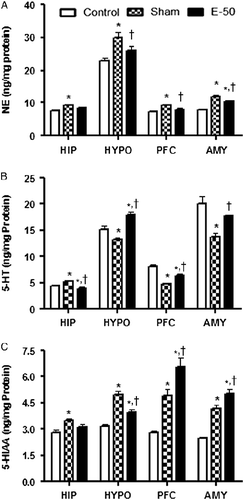
Effect of eugenol on brain serotonin (5-HT) levels
The effect of eugenol on 5-HT levels in HIP, HYPO, PFC, and AMY is illustrated in . Statistical analysis by one-way ANOVA revealed that there was a significant difference in 5-HT levels among groups in HIP [F (2, 12) = 15.04, P < 0.05], HYPO [F (2, 12) = 20.94, P < 0.05], PFC [F (2, 12) = 32.09, P < 0.05], and AMY [F (2, 12) = 13.35, P < 0.05]. Post hoc analysis suggested that RS increased 5-HT levels in HIP and on the contrary decreased 5-HT levels in other brain regions, namely HYPO, PFC, and AMY. Eugenol (50 mg/kg) pretreatment reversed the effect of RS on 5-HT levels in all the brain regions. However, 5-HT levels were increased near to normal values after eugenol pretreatment only in AMY.
Effect of eugenol on brain 5-hydroxyindoleacetic acid levels
illustrates the effect of eugenol (50 mg/kg) on 5-hydroxyindoleacetic acid (5-HIAA) levels of HIP, HYPO, PFC, and AMY. Significant differences in 5-HIAA levels of HIP [F (2, 12) = 6.734, P < 0.05], HYPO [F (2, 12) = 30.14, P < 0.05], PFC [F (2, 12) = 26.83, P < 0.05], and AMY [F (2, 12) = 35.52, P < 0.05] between vehicle and treatment group were shown by statistical analysis using one-way ANOVA. Post hoc analysis showed a significant increase in 5-HIAA levels in all the brain regions in sham compared to those in control group. Eugenol pretreatment decreased the effect of RS on 5-HIAA levels in HYPO. It potentiated the effects of RS on 5-HIAA concentrations in the PFC and AMY. However, eugenol pretreatment did not alter the 5-HIAA levels in HIP relative to those in stress controls.
Effect of eugenol on brain DA levels
The effect of eugenol pretreatment on DA levels in HIP, HYPO, PFC, and AMY is shown in . Statistical analysis by one-way ANOVA showed a significant difference in DA levels among groups in HIP [F (2, 12) = 20.37, P < 0.05], HYPO [F (2, 12) = 8.646, P < 0.05], PFC [F (2, 12) = 27.90, P < 0.05], and AMY [F (2, 12) = 13.99, P < 0.05]. Post hoc analysis revealed that the DA levels were significantly increased in the HIP, while the levels were significantly decreased in HYPO, PFC, and AMY after stress exposure. Eugenol pretreatment significantly attenuated the stress-induced decreases in DA concentrations in PFC and AMY, but not in the HYPO. Furthermore, eugenol did not alter the effect of RS-induced increase in hippocampal DA levels.
Figure 4. Bar diagram representing the RS-induced changes in the levels of DA (A), DOPAC (B), and HVA (C) of control, sham, and eugenol treated (50 mg/kg) groups in HIP, HYPO, PFC, and AMY regions of brain. Results are represented as mean ± SEM with n = 6 in each group. “n” is the number of rats used. *P < 0.05 compared to control and †P < 0.05 compared to sham group (Student–Newmann–Keuls post hoc test).
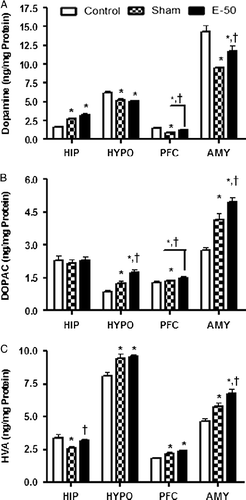
Effect of eugenol on brain 3, 4-dihydroxyphenylacetic acid levels
illustrates the effect of eugenol pretreatment on dihydroxyphenylacetic acid (DOPAC) levels of HIP, HYPO, PFC, and AMY. One-way ANOVA indicated significant changes in DOPAC levels of HIP [F (2, 12) = 0.1325, P < 0.05], HYPO [F (2, 12) = 14.84, P < 0.05], PFC [F (2, 12) = 1.961, P < 0.05], and AMY [F (2, 12) = 21.32, P < 0.05] among groups. Post hoc analysis suggested that RS significantly increased the DOPAC levels in HYPO, PFC, and AMY, but not in the HIP. Interestingly, the increase in DOPAC in HYPO, PFC, and AMY by eugenol was significantly higher than sham values. Eugenol did not alter the hippocampal DOPAC levels which were, however, unaltered by stress.
Effect of eugenol on brain homovanillic acid levels
illustrates the effect of eugenol on homovanillic acid (HVA) levels in HIP, HYPO, PFC, and AMY. Statistical analysis by one-way ANOVA indicated a significant interaction among groups in terms of HVA levels in HIP [F (2, 12) = 4.886, P < 0.05], HYPO [F (2, 12) = 10.42, P < 0.05], PFC [F (2, 12) = 11.51, P < 0.05], and AMY [F (2, 12) = 14.76, P < 0.05]. Post hoc analysis suggested that RS significantly increased HVA levels in HYPO, PFC, and AMY similar to the DOPAC observations. However, HVA levels were significantly decreased in the HIP after RS in contrast to DOPAC which was unaltered by stress. RS-induced decrease in HVA levels in the HIP was reversed by eugenol pretreatment. Eugenol pretreatment did not alter the effect of RS on HVA levels in the HYPO and PFC. However, amygdala HVA levels were significantly higher in treatment group than in sham.
Effect of eugenol on brain DOPAC/DA ratio
Effect of eugenol pretreatment on DOPAC/DA ratio in HIP, HYPO, PFC, and AMY is shown in . One-way ANOVA revealed that there was a significant interaction among groups in terms of DOPAC/DA ratio in HIP [F (2, 12) = 52.07, P < 0.05], HYPO [F (2, 12) = 21.50, P < 0.05], PFC [F (2, 12) = 13.50, P < 0.05], and AMY [F (2, 12) = 25.33, P < 0.05]. Post hoc analysis suggested that RS significantly increased the DOPAC/DA ratio in HYPO, PFC, and AMY. However, the DOPAC/DA ratio was significantly decreased in the HIP after RS. Eugenol pretreatment did not alter the effect of RS on DOPAC/DA ratio in HIP and AMY. Surprisingly, in HYPO, the DOPAC/DA ratio was significantly higher in treatment group than in sham. In PFC, eugenol pretreatment reversed the stress-induced increase in DOPAC/DA ratio.
Figure 5. Bar diagram representing the RS-induced changes in the metabolite/monoamine ratios, DOPAC/DA (A), HVA/DA (B), and 5-HIAA/5-HT (C) of control, sham, and eugenol-treated (50 mg/kg) groups in HIP, HYPO, PFC, and AMY regions of brain. Results are represented as mean ± SEM with n = 6 in each group. “n” is the number of rats used. *P < 0.05 compared to control and †P < 0.05 compared to sham group (Student–Newmann–Keuls post hoc test).
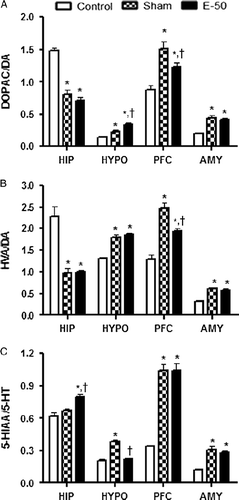
Effect of eugenol on brain HVA/DA ratio
Results of the effect of eugenol pretreatment on HVA/DA ratio in HIP, HYPO, PFC, and AMY are illustrated in . One-way ANOVA revealed that there was a significant difference in HVA/DA ratio in HIP [F (2, 12) = 24.40, P < 0.05], HYPO [F (2, 12) = 45.79, P < 0.05], PFC [F (2, 12) = 34.48, P < 0.05], and AMY [F (2, 12) = 32.23, P < 0.05] among groups. Post hoc analysis suggested that stress significantly decreased the HVA/DA ratio in HIP. However, HVA/DA ratio was increased in HYPO, PFC, and AMY after stress exposure. Eugenol pretreatment significantly reversed the stress-induced increase in HVA/DA ratio in the PFC. However, eugenol pretreatment did not alter the effect of RS on HVA/DA ratio in HIP, HYPO, and AMY.
Effect of eugenol on brain 5-HIAA/5-HT ratio
shows the effect of eugenol on 5-HIAA/5-HT ratio in HIP, HYPO, PFC, and AMY. One-way ANOVA showed a significant interaction among groups in HIP [F (2, 12) = 15.24, P < 0.05], HYPO [F (2, 12) = 45.50, P < 0.05], PFC [F (2, 12) = 56.98, P < 0.05], and AMY [F (2, 12) = 22.36, P < 0.05]. Post hoc analysis revealed that there was a significant increase in the 5-HIAA/5-HT ratio in HYPO, PFC, and AMY except in HIP after exposure to RS compared to control values. Eugenol pretreatment significantly increased the 5-HIAA/5-HT ratio in HIP compared to sham group. However, eugenol significantly reversed the RS-induced increase in 5-HIAA/5-HT ratio in the HYPO and did not alter the RS-induced 5-HIAA/5-HT ratio in PFC and AMY.
Discussion
In this study, eugenol pretreatment showed significant anti-stress activity in the 4-h RS model. Interestingly, for the first time we report a U-shaped dose–response relationship for the anti-stress effect of eugenol both in terms of reduction in ulcer index and in its effect on plasma corticosterone. The anti-stress effect of eugenol, reducing stress-induced increase in protein cauterization and fluidity of synaptosomal membrane, has been reported elsewhere (Sen et al. Citation1992). However, we for the first time report the anti-stress activity of eugenol by its ability to reduce stress-induced HPA axis activation.
An individual is exposed regularly to day-to-day life mild stressors. These mild stressors may lead to development of chronic stress disorders. Acute RS is considered to be a mild stressor. Exposure of organism to any kind of stressor markedly activates the SAS and HPA systems (Kvetnanský et al. Citation1995) leading to an increase in plasma NE and corticosterone levels, respectively. Plasma corticosterone and NE levels are reported to increase after acute immobilization stress (Gavrilovic and Dronjak Citation2005). In this study, RS for 4 h produced gastric ulcers, while eugenol pretreatment decreased gastric ulcers demonstrated by a decrease in ulcer index. Interestingly, eugenol showed a “U”-shaped dose–response curve in terms of reduction in ulcer scores. Stress exposure increased both plasma NE and corticosterone levels indicating activation of both SAS and HPA systems, respectively. Eugenol pretreatment for 7 days decreased the stress-induced increase in plasma corticosterone levels. Furthermore, eugenol showed a dose-dependent U-shaped response curve with plasma corticosterone levels; the median dose being more effective than the lower or higher doses. This effect was similar to the U-shaped anti-stress effect assessed by ulcer index, indicating a possibility that eugenol may act specifically on the hyperactive HPA axis during stress. Surprisingly, eugenol did not alter stress-induced increase in plasma NE levels. These results taken together suggest that eugenol may selectively inhibit stress-induced activation of HPA axis without affecting SAS. SAS is an important component of fight-or-flight in response to stressful stimuli, and it can activate the central noradrenergic system as part of allostatic response (Viljoen and Panzer Citation2007). Activation of central noradrenergic system is known to increase plasma corticosterone levels, forming a feedforward cycle of plasma corticosterone and NE levels. It may be assumed that by selectively interfering with the HPA axis independently of SAS, eugenol may break the feedforward cycle of CRH–NE interaction. This can prevent the progression from allostasis to allostatic load and subsequent development of stress-related disorders. However, this contention has to be clarified by further experiments to evaluate the specific role of eugenol on the HPA axis and its relation with the SAS system.
The HPA axis is regulated by higher brain regions such as HIP, PFC, and AMY. The HIP has inhibitory action on the HPA axis, while AMY activates the HPA axis as discussed earlier (Herman and Cullinan Citation1997). PFC has dual action depending on the hemisphere activated (Czéh et al. Citation2008). These brain regions innervate the PVN of the HYPO through various neurotransmitters (Herman et al. Citation2005). The neurotransmitters NE, 5-HT, and DA are important monoamines which are widely distributed in brain, and their functional role is well established during stressful conditions. Changes in their activity lead to alteration in the HPA axis homeostasis, leading to development of various psychological and physiological disorders (Sheikh et al. Citation2007). Most of the NE in the brain arises from cell bodies in the locus ceruleus (LC; Gregory et al. Citation2002). The PFC has substantial noradrenergic input from the LC (Ziegler et al. Citation1999), which also innervates the limbic brain regions, including the AMY and HIP (Gregory et al. Citation2002). In addition to innervations of the forebrain regions, the LC also densely innervates other monoaminergic nuclei, including the serotonergic raphe nuclei and the dopaminergic ventral tegmental area (VTA). During stress, the brain NE levels have been reported to increase in central nucleus of AMY (Pacák et al. Citation1993). Prolongation of the noradrenergic response in the frontal cortex and HYPO was reported for aversive environmental stimuli (McQuade and Stanford Citation2000). Similar results were obtained in this study. RS increased NE levels in HIP, HYPO, PFC, and AMY. It has been reported that NE excites raphe neurons through noradrenergic input to raphe nucleus. Raphe nuclei innervate the higher brain regions via serotonergic pathways, mostly HIP, cortex, and AMY. The pathway connecting the median raphe nucleus (MRN) and the HIP is involved in regulation of the stress response (Graeff et al. Citation1996, Joca et al. Citation2007). Ascending 5-HT pathways running from the dorsal raphe nucleus (DRN) innervate AMY and PFC and facilitate active avoidance behavior after potential threat. 5-HT released from the nerve terminals of DRN increases anxiety through AMY (Graeff et al. Citation1996). Exposure of rats to restraint, forced swim stress, or defeat by a larger rat, increases the expression of c-Fos protein in the DRN (Van De Kar and Blair Citation1999). It has been reported that brain 5-HT levels were increased after 1, 2, and 3 h of RS, but not after 5 h of RS possibly due to adaptation to the stressful stimuli (Richardson Citation1984). In contrast, Kirby et al. (Citation1997) have shown an increase in hippocampal 5-HIAA but not 5-HT after 100 min of RS. Furthermore, another study has shown that RS of 180 min had no effect on 5-HT levels in frontal cortex, HIP, HYPO, and AMY, but the 5-HIAA levels in AMY were significantly higher after 120 and 180 min, and in HYPO after 180 min (Mitchell and Thomas Citation1988). In this study, 5-HT levels were increased in the HIP, but were decreased in HYPO, PFC, and AMY after 4 h of RS. It has been reported that acute stress preferentially activates the MRN 5-HT neurons rather than the DRN 5-HT neurons (Corley et al. Citation2002). The above findings justify an increase in only hippocampal 5-HT. Collectively, the results from the 5-HT levels from discrete brain regions show the effort of limbic structures to mitigate the effects of the stress response. Stress increased the metabolism of 5-HT to 5-HIAA in all the brain regions. It has been suggested that 5-HIAA mainly reflects intraneuronal metabolism of 5-HT and probably has no relation to the 5-HT release (Auerbach et al. Citation1989). 5-HIAA/5-HT ratio is considered to be the index of serotonin deamination (Popova et al. Citation2001). Stress increased the 5-HIAA/5-HT ratio in HYPO, PFC, and AMY, but no change was observed in HIP. This indicates that stress increased the 5-HT metabolism in HYPO, PFC, and AMY but not in HIP. Eugenol reversed the stress-induced changes on 5-HT levels in all the brain regions. Furthermore, eugenol pretreatment decreased the effect of RS on 5-HIAA levels in HYPO. It potentiated the effects of RS on 5-HIAA concentrations in the PFC and AMY. However, eugenol pretreatment did not alter the 5-HIAA levels in HIP relative to stress controls. This probably is due to a decrease in hippocampal 5-HT by eugenol. Eugenol pretreatment potentiated 5-HT metabolism in the HIP but decreased it in HYPO. However, eugenol did not alter the 5-HT metabolism in PFC and AMY. One of the factors responsible for the decrease in 5-HT metabolism by eugenol may be its reported inhibition of human recombinant MAO-A and MAO-B at higher concentrations (Tao et al. Citation2005). In support of its MAO inhibitory activity, eugenol attenuated the stress-induced increase in 5-HIAA in PFC and AMY, but, however, increased the 5-HIAA levels in HIP and HYPO. The likely region-specific MAO-A inhibitory activity has to be further investigated before arriving at any conclusions.
Similar to the serotonergic system, LC activation is also responsible for activation of the VTA, leading to an increase in dopaminergic activity. Excitation of the VTA by NE has been reported to occur through α1-receptor. Kurata et al. (Citation1993) have reported an increase in DA levels in the anteromedial frontal cortex (AMFCx) during 2 h of tight RS. However, in the same study, AMFCx DA levels had reached baseline values at 2 h following the immediate increase within 20 min. Another study had shown an increase in extracellular DOPAC levels in the AMY during 2 h of RS (Pacák et al. Citation1993). In this study, RS increased the DA levels in the HIP but reduced DA levels in other brain regions, which was similar to the effect of RS on 5-HT levels indicating a region-specific effect of stress. Dopaminergic innervations of the AMY are believed to be highly responsive to stress (Inglis and Moghaddam Citation1999). A decrease in DA levels in the PFC can be explained by the fact that mesoamygdaloid DA exerts a facilitatory action on the right mPFC (Stevenson et al. Citation2003). Hence, a decrease in DA levels of the AMY may lead to a decrease in DA levels in the PFC. Eugenol selectively attenuated stress-induced decreases in DA concentrations in the PFC and AMY. Furthermore, there was a increase in DOPAC and HVA in all the regions except HIP after stress exposure. The primary route of DA metabolism is through the intraneural oxidative deamination to DOPAC by MAO. DOPAC is then O-methylated extra-neuronally by catechol-O-methyl transferase (COMT) to HVA (Westerink Citation1985). Hence, DOPAC/DA and HVA/DA ratios are regarded as indexes of DA metabolism by MAO and COMT, respectively. RS showed a region-specific effect on DA metabolism. The DOPAC/DA and HVA/DA ratios were increased by stress in HYPO, PFC, and AMY, but these ratios decreased in HIP. This shows that stress increased in both MAO-dependent and COMT-dependant metabolisms of DA in HYPO, PFC, and AMY but decreased the DA metabolism in the HIP. Eugenol pretreatment further augmented stress-induced increase in MAO-dependent DA metabolism in terms of DOPAC/DA ratio in the HYPO but not in COMT-dependant metabolism in terms of HVA/DA ratio. In contrast, eugenol attenuated both the metabolic pathways of conversion of DA to DOPAC and HVA in PFC. However, eugenol did not alter the stress-induced changes in intraneural or extraneural metabolism of DA in the HIP and AMY. Thus, eugenol had region- and enzyme-specific actions on stress-induced changes in DA metabolism. In summary, the reversal of stress-induced changes in DA by eugenol was region-specific. However, eugenol reversed the stress-induced changes in the NE and 5-HT levels in all the brain regions. These results indicate that the anti-stress activity of eugenol in a 4-h restraint model is mediated through the HPA axis and brain noradrenergic and serotonergic systems. Furthermore, studies involving pharmacological depletion of specific neurotransmitters in discrete brain regions would provide more specific information on the role of these transmitters in the anti-stress response of eugenol.
In this study, eugenol showed significant anti-stress effects in the 4-h RS model. The anti-stress effect of eugenol in terms of the reduction in ulcer index showed a “U”-shaped curve; the median dose was more active than the corresponding lower and higher doses. Eugenol pretreatment reversed the stress-induced increase in corticosterone, but not plasma NE levels possibly indicating specific activity on the HPA axis allostatic response rather than on the SAS. It is interesting to note that the nature of the dose–response effect of eugenol on corticosterone was similar to the anti-stress response in terms of reduction of ulcer index. This observation perhaps suggests that the major pathway mediating the anti-stress response could be the HPA axis. Furthermore, eugenol reversed the stress-induced changes on brain noradrenergic and serotonergic systems and most of the changes in dopaminergic system in HIP, HYPO, PFC, and AMY, suggesting the involvement of BMS pathways in the anti-stress effect. It would be interesting to further investigate the cellular and molecular mechanisms of anti-stress activity of eugenol.
Acknowledgement
We gratefully acknowledge Prime Dental Pvt. Ltd, Mumbai, for supplying us a gift sample of eugenol.
Declaration of Interest: The authors report no conflicts of interest. The authors alone are responsible for the content and writing of the paper.
References
- Akirav I, Richter-Levin G. 1999. Biphasic modulation of hippocampal plasticity by behavioral stress and basolateral amygdala stimulation in the rat. J Neurosci. 19 23: 10530–10535.
- Ardjmand A, Fathollahi Y, Sayyah M, Kamalinejad M, Omrani A. 2006. Eugenol depresses synaptic transmission but does not prevent the induction of long-term potentiation in the CA1 region of rat hippocampal slices. Phytomedicine. 13:146–151.
- Auerbach SB, Minzeberg MJ, Wilkinson LO. 1989. Extracellular serotonin and 5-hydroxyindoleacetic acid in hypothalamus of the anaesthetized rat measured by in vivo dialysis coupled to high-performance liquid chromatography with electrochemical detection: Dialysate serotonin reflects neuronal release. Brain Res. 499:281–290.
- Brady KT, Sinha R. 2005. Co-occurring mental and substance use disorders: The neurobiological effects of chronic stress. Am J Psychiatry. 162:1483–1493.
- Brodin P, Roed A. 1984. Effects of eugenol on rat phrenic nerve and phrenic nerve-diaphragm preparations. Arch Oral Biol. 29:611–615.
- Burchfield SR. 1979. The stress response: A new perspective. Psychosom Med. 41 8: 661–672.
- Charney DS. 2004. Psychobiological mechanisms of resilience and vulnerability: Implications for successful adaptation to extreme stress. Am J Psychiatry. 161:195–216.
- Corley KC, Phan TH, Daugherty WP, Boadle-Biber MC. 2002. Stress-induced activation of median raphe serotonergic neurons in rats is potentiated by the neurotensin antagonist, SR 48692. Neurosci Lett. 319 1: 1–4.
- Czéh C, Perez-Cruz C, Fuchs E, Flügge G. 2008. Chronic stress-induced cellular changes in the medial prefrontal cortex and their potential clinical implications: Does hemisphere location matter?. Behav Brain Res. 190:1–13.
- Feldman S, Weidenfeld J. 1995. Neural mechanisms involved in the corticosteroid feedback effects on the hypothalamo–pituitary–adrenocortical axis. Prog Neurobiol. 45:129–141.
- Floresco SB, Grace AA. 2003. Gating of hippocampal-evoked activity in prefrontal cortical neurons by inputs from the mediodorsal thalamus and ventral tegmental area. J Neurosci. 23 9: 3930–3943.
- Gavrilovic L, Dronjak S. 2005. Activation of rat pituitary–adrenocortical and sympatho-adrenomedullary system in response to different stressors. Neuro Endocrinol Lett. 26 5: 515–520.
- Graeff FG, Guimarães FS, De Andrade TG, Deakin JF. 1996. Role of 5-HT in stress, anxiety, and depression. Pharmacol Biochem Behav. 54:129–141.
- Gregory AO, Violetta K, John JM. 2002. Neurocircuitry of mood disorders. Neuropsychopharmacology. 73:1051–1064.
- Hasler G, Drevets WC, Manji HK, Charney DS. 2004. Discovering endophenotypes for major depression. Neuropsychopharmacology. 29:1765–1781.
- Hennessy MB, Levine S. 1979. Sensitive pituitary–adrenal responsiveness to varying intensities of psychological stimulation. Physiol Behav. 21 3: 295–297.
- Herman JP, Cullinan WE. 1997. Neurocircuitry of stress: Central control of the hypothalamo–pituitary–adrenocortical axis. Trends Neurosci. 20:78–84.
- Herman JP, Ostrander MM, Mueller NK, Figueiredo H. 2005. Limbic system mechanisms of stress regulation: Hypothalamo–pituitary–adrenocortical axis. Prog Neuropsychopharmacol Biol Psychiatry. 29:1201–1213.
- Inglis FM, Moghaddam B. 1999. Dopaminergic innervation of the amygdala is highly responsive to stress. J Neurochem. 72 3: 1088–1094.
- Irie Y, Keung WM. 2003. Rhizoma acori graminei and its active principles protect PC-12 cells from the toxic effect of amyloid-β peptide. Brain Res. 963:282–289.
- Irie Y, Itokazu N, Anjiki N, Ishige A, Watanabe K, Keung WM. 2004. Eugenol exhibits antidepressant-like activity in mice and induces expression of metallothionein-III in the hippocampus. Brain Res. 1011 2: 243–246.
- Jacobson L, Sapolsky R. 1991. The role of the hippocampus in feedback regulation of the hypothalamic–pituitary–adrenocortical axis. Endocr Rev. 12 2: 118–134.
- Jankord R, Herman JP. 2008. Limbic regulation of hypothalamo–pituitary–adrenocortical function during acute and chronic stress. Ann N Y Acad Sci. 1148:64–73.
- Joca SR, Ferreira FR, Guimarães FS. 2007. Modulation of stress consequences by hippocampal monoaminergic, glutamatergic and nitrergic neurotransmitter systems. Stress. 10:227–249.
- Kim C, Speisky MB, Kharouba SN. 1987. Rapid and sensitive method for measuring norepinephrine, dopamine, 5-hydroxytryptamine and their major metabolites in rat brain by high-performance liquid chromatography. Differential effect of probenecid, haloperidol and yohimbine on the concentrations of biogenic amines and metabolites in various regions of rat brain. J Chromatogr. 386:25–35.
- Kirby LG, Chou-Green JM, Davis K, Lucki I. 1997. The effects of different stressors on extracellular 5-hydroxytryptamine and 5-hydroxyindoleacetic acid. Brain Res. 760 1–2: 218–230.
- Koob GF. 1999. Stress, corticotropin-releasing factor, and drug addiction. Ann N Y Acad Sci. 897:27–45.
- Kurata K, Tanii Y, Shibata R, Kurachi M. 1993. Differential effects of tight and loose 2-hour restraint stress on extracellular concentrations of dopamine in nucleus accumbens and anteromedial frontal cortex. Jpn J Psychiatr Neurol. 47 1: 57–61.
- Kvetnanský R, Pacák K, Fukuhara K, Viskupic E, Hiremagalur B, Nankova B, Goldstein DS, Sabban EL, Kopin IJ. 1995. Sympathoadrenal system in stress: Interaction with the hypothalamic–pituitary–adrenocortical system. Ann N Y Acad Sci. 771:131–158.
- Lowry OH, Rosenborough NJ, Farr AL, Randall RJ. 1951. Protein measurement with folin phenol reagent. J Biol Chem. 193:265–275.
- Marinelli M, Piazza PV. 2002. Interaction between glucocorticoid hormones, stress and psychostimulant drugs. Eur J Neurosci. 16:387–394.
- Maroun M, Richter-Levin G. 2003. Exposure to acute stress blocks the induction of long-term potentiation of the amygdala-prefrontal cortex pathway in vivo. J Neurosci. 23 11: 4406–4409.
- McEwen BS. 2000. The neurobiology of stress: From serendipity to clinical relevance. Brain Res. 886 1–2: 172–189.
- McQuade R, Stanford SC. 2000. A microdialysis study of the noradrenergic response in rat frontal cortex and hypothalamus to a conditioned cue for aversive, naturalistic environmental stimuli. Psychopharmacology. 148:201–208.
- Mitchell SN, Thomas PJ. 1988. Effect of restraint stress and anxiolytics on 5-HT turnover in rat brain. Pharmacology. 37 2: 105–113.
- Müller M, Pape HC, Speckmann EJ, Gorji A. 2006. Effect of eugenol on spreading depression and epileptiform discharges in rat neocortical and hippocampal tissues. Neuroscience. 140 2: 743–751.
- Pacák K, Palkovits M, Kvetnanský R, Fukuhara K, Armando I, Kopin IJ, Goldstein DS. 1993. Effects of single or repeated immobilization on release of norepinephrine and its metabolites in the central nucleus of the amygdala in conscious rats. Neuroendocrinology. 57 4: 626–633.
- Palkovits M, Brownstein MJ. 1988. Maps and guide to microdissection of the rat brain. New York: Elsevier.
- Pfaff H. 2006. Health care research: The last mile in sight. Dtsch Med Wochenschr. 131 25-26: 1488–1490.
- Phillips LJ, McGorry PD, Garner B, Thompson KN, Pantelis C, Wood SJ, Berger G. 2006. Stress, the hippocampus and the hypothalamic–pituitary–adrenal axis: Implications for the development of psychotic disorders. Aus N Z J Psychiatry. 40:725–741.
- Popova NK, Gilinsky MA, Amstislavskaya TG, Morosova EA, Seif I, De Maeyer E. 2001. Regional serotonin metabolism in the brain of transgenic mice lacking monoamine oxidase A. J Neurosci Res. 66 3: 423–427.
- Richardson JS. 1984. Brain part monoamines in the neuroendocrine mechanisms activated by immobilization stress in the rat. Int J Neurosci. 23 1: 57–67.
- Sairam K, Priyambada S, Aryya NC, Goel RK. 2003. Gastroduodenal ulcer protective activity of Asparagus racemosus: An experimental, biochemical and histological study. J Ethnopharmacol. 86 1: 1–10.
- Sapolsky RM, Krey LC, McEwen BS. 1986. The neuroendocrinology of stress and aging: The glucocorticoid cascade hypothesis. Endocr Rev. 7 3: 284–301.
- Sastre B, Nicolay A, Bruguerolle B, Portugal H. 2004. Method for simultaneous measurement of norepinephrine, 3-methoxy-4-hydroxyphenylglycol and 3,4-dihydroxyphenylglycol by liquid chromatography with electrochemical detection: Application in rat cerebral cortex and plasma after lithium chloride treatment. J Chromatogr B Analyt Technol Biomed Life Sci. 801 2: 205–211.
- Selye H. 1936. A Syndrome Produced by Diverse Nocuous Agents. Nature. 138:32.
- Sen P, Maiti PC, Puri S, Ray A, Audulov NA, Valdman AV. 1992. Mechanism of anti-stress activity of Ocimum sanctum Linn, eugenol and Tinospora malabarica in experimental animals. Indian J Exp Biol. 30 7: 592–596.
- Shah ZA, Gilani RA, Sharma P, Vohora SB. 2004. Attenuation of stress-elicited brain catecholamines, serotonin and plasma corticosterone levels by calcined gold preparations used in Indian system of medicine. Basic Clin Pharmacol Toxicol. 96:469–474.
- Sheikh N, Ahmad A, Siripurapu KB, Kuchibhotla VK, Singh S, Palit G. 2007. Effect of Bacopa monniera on stress induced changes in plasma corticosterone and brain monoamines in rats. J Ethnopharmacol. 111:671–676.
- Stevenson CW, Sullivan RM, Gratton A. 2003. Effects of basolateral amygdala dopamine depletion on the nucleus accumbens and medial prefrontal cortical dopamine responses to stress. Neuroscience. 116 1: 285–293.
- Tao G, Irie Y, Li DJ, Keung WM. 2005. Eugenol and its structural analogs inhibit monoamine oxidase A and exhibit antidepressant-like activity. Bioorg Med Chem. 13 15: 4777–4788.
- Van De Kar LD, Blair ML. 1999. Forebrain pathways mediating stress-induced hormone secretion. Front Neuroendocrinol. 20:1–48.
- Varel VH, Miller DN. 2004. Plant oils thymol and eugenol affect cattle and swine waste emissions differently. Water Sci Technol. 50 4: 207–213.
- Viljoen M, Panzer A. 2007. The central noradrenergic system: An overview. Afr J Psychiatry. 10:135–141.
- Westerink BHC. 1985. Sequence and significance of dopamine metabolism in the rat-brain. Neurochem Int. 7:221–227.
- Wie MB, Won MH, Lee KH, Shin JH, Lee JC, Suh HW, Song DK, Kim YH. 1997. Eugenol protects neuronal cells from excitotoxic and oxidative injury in primary cortical cultures. Neurosci Lett. 225 2: 93–96.
- Won MH, Lee JC, Kim YH, Song DK, Suh HW, Oh YS, Kim JH, Shin TK, Lee YJ, Wie MB. 1998. Postischemic hypothermia induced by eugenol protects hippocampal neurons from global ischemia in gerbils. Neurosci Lett. 254 2: 101–104.
- Woodward CJ, Emery PW. 1987. Determination of plasma corticosterone using high-performance liquid chromatography. J Chromatogr. 419:280–284.
- Yazaki K. 1989. Study of behavioral pharmacology on rats. Tranquilizing effects induced by endogenous or exogenous bradykinin. Shikwa Gakuho. 89 10: 1529–1548.
- Ziegler DR, Cass WA, Herman JP. 1999. Excitatory influence of the locus coeruleus in hypothalamic–pituitary–adrenocortical axis responses to stress. J Neuroendocrinol. 11:361–369.