Abstract
Lipid-based drug delivery systems show great potential for enhancing oral bioavailability but have not been broadly applied, largely due to lack of general formulation guidance. In the previous studies, three different formulations including anionic SLNs, cationic SLNs, and liposomes were investigated and significantly enhanced the oral bioavailability of N3-o-toluyl-fluorouracil (TFu) compared with its aqueous suspension, which indicated their high potential as oral delivery carriers. In order to define which formulation is worthy of being further researched and developed, the studies on Caco-2 cell model and rat intestine were investigated. In both studies of crossing Caco-2 cell monolayers and the single-pass intestinal perfusion (SPIP) in rat, SLNs exhibited much more capability to enhance transport of TFu than liposomes. More specifically, in cell study, the Papp values of cationic SLNs (p < 0.01) and anionic SLNs (p < 0.05) were significantly higher than liposomes. Especially the cationic SLNs present the most effective capacity. During SPIP study, both Ka and uptake percentage of these three different formulations followed a rank order: anionic SLN > cationic SLN > liposomes. In addition, the Peff of different nanocarriers in various intestinal segments indicated they all exhibited site-dependent absorption behavior. By comparing the transmucosal behavior of these nanocarriers in vitro and in vivo, the anionic SLNs were identified to be more effective in the transport of TFu and were worthy of being further researched and developed.
Introduction
A significant number (40–70%) of drug candidates identified in drug discovery programs, such as combinatorial chemistry research or high-throughput screening, are quite often very lipophilic, which are insufficiently soluble in aqueous media to allow for their adequate and reproducible absorption from the gastrointestinal tract after oral administration (CitationMuller et al., 2006). This challenges drug delivery institutions in industry or academia to develop carrier systems for the optimal oral and parenteral administration of these drugs. In the past decade, significant efforts in pharmaceutical and academic laboratories have been made in finding ways to deliver poorly bioavailable hydrophobic drug molecules by the oral route. Fortunately, the knowledge regarding the mechanisms of interaction between the nanostructured biomaterials and the biological surfaces, as well as the discovery of new nanotechnologies and characterization approaches, have led to a promising perspective on the use of lipid-based nanocarriers for transmucosal macromolecular drug delivery (CitationAlonso, 2004; CitationAlonso & Sánchez, 2004). The effect of the nanocarriers based on lipid formulations, such as liposomes and solid lipid nanoparticles (SLN), on oral bioavailability of lipophilic drugs was extensively reviewed and different mechanisms (physicochemical, biochemical, and lymphatic transport) for the enhanced bioavailability have been illustrated (CitationPorter & Charman, 2001).
N3-o-toluyl-fluorouracil (tofluding, TFu), the chemical structure of which is shown in , is a newly developed potential anti-tumor lipophilic drug under investigation by our group. Its anti-cancer efficacy was ascertained through rigorous experiments in vitro and in mice (CitationLiu et al., 2006). As one of the pro-drugs of 5-fluorouracil (5-Fu), TFu could be selectively metabolized to 5-Fu in the tumor tissue due to the higher level of amidase activity in the tumor cells. However, the harsh conditions of the gastrointestinal tract and the limited permeability across the intestinal barrier makes the situation very complex and, hence, the clinical application of TFu is greatly restricted by the low bioavailability after oral administration (CitationNorris et al., 1998; CitationSzakács et al., 2001; CitationNassar et al., 2008).
Hence, an oral formulation with a high degree of oral absorption would be highly desirable (CitationLuo et al., 2006). We have successfully prepared TFu-liposomes and two types (cationic and anionic) of TFu-SLNs (CitationSun et al., 2008; CitationZou et al., 2008; CitationBai et al., 2009; Liu et al., 2009). Based on a common conclusion that positively charged colloidal drug carriers could increase the permeability and potential uptake of the slightly soluble drugs when compared with the neutral or negatively charged ones (CitationEl-Shabouri, 2002, CitationPeira et al., 2008), we hypothesized that the cationic one would be the most potential carriers depending on their electrostatic interaction with the epithelial cells in gastrointestinal tract. However, the initial studies led us to know that all three formulations could significantly enhance the bioavailability of TFu, but there are no significant differences among them. In order to define which formulation is worthy of being further researched and developed, further investigations in both cell and animal levels were conducted.
The stability in the biological fluids and their interaction with the mucosal surfaces of the particles are governed by many factors, including particle size, surface properties, and composition of the nanocarriers (CitationYuan et al., 2007). Indeed, at present, there is no doubt on the fact that the size is a critical parameter for the nanocarriers to cross the biological barriers (CitationDesai et al., 1997; CitationFlorence, 1997). However, the question that remains to be answered is how to optimize the abilities of these systems to overcome the barriers. The intestinal permeability is the propensity of a compound to move across the epithelial barrier of the intestine. In vitro and in situ absorption models, such as in situ perfusion of rat intestine, Caco-2 cell monolayer model, and excised intestinal segments in the ussing chamber, are valuable tools in the discovery of new chemical entities that are bioavailable after oral administration (CitationStewart et al., 1995).
Therefore, in the present study, the specific objective of the study was to compare the interaction of the nanoparticles with the Caco-2 cell culture model and the intestinal permeabilities obtained in the three absorption nanocarriers, and, eventually, to provide the evidence of the nanocarrier designation for a potential lipid-based drug delivery system for enhancing oral bioavailability of poorly soluble drugs and their effective clinical application.
Materials and methods
Materials
N3-o-toluyl-fluorouracil (TFu) was donated by the Institute of Pharmacochemistry of the Shandong University. Injectable soya lecithin (phosphatidylcholine accounts for 95%, pH = 5.0∼7.0) was provided by the Shanghai Taiwei Pharmaceutical Co., Ltd. (Shanghai, China). Cholesterol and sodium dodecyl sulfate (SDS) were obtained from the Shanghai Medical Chemical Reagent Co. Ltd. (Shanghai, China). ATO888 (Compritol® 888 ATO) was purchased from Gattefossé (Saint-Priest, France). Poloxamer 188 (Pluronic F-68®) was supplied by Sigma-Aldrich (St. Louis, MO, USA). Cetyl Trimethyl Ammonium Bromide (CTAB) was purchased from Sigma-Aldrich (St. Louis, MO, USA). All the other chemicals and reagents used were of analytical purity grade or higher, obtained commercially.
Formulation and characterization of nanocarriers
Formulation
Different TFu-loaded lipid-based nanocarriers were prepared according to the procedure previously described (CitationSun et al., 2008; CitationBai et al., 2009; Liu et al., 2009). More specifically, cationic TFu-SLNs were prepared by film-dispersion method. Briefly, various amounts of lecithin, Compritol®888, and TFu were dissolved in 5 ml chloroform, and the solution evaporated under reduced pressure to form a thin layer of uniform film at the bottom of the bottle using a rotary evaporator (RE52-98, Shanghai Yarong Biochemistry Instrument Factory, Shanghai, China). Residue of the organic solvent was expelled under vacuum under a water bath at 60°C for 1 h. Ten milliliters of aqueous phase containing surfactant CTAB was added to allow the film to expand and disperse, and then the mixture further dispersed ultrasonically for 4 min by ultrasonicor (250 W, Ultrasonic Cell Pulverizer, JY88-II, Xinzhi Scientific Instrument Institute, Ningbo, China).
Anionic TFu-SLNs were obtained following the same procedure described above but substituting the CTAB with F-68.
TFu-liposomes were prepared by lipid film hydration technique and homogenized to the desired sizes. Briefly, Soya lecithin, cholesterol, and TFu were dissolved in ether, and ether was evaporated using a rotary vacuum evaporator to produce a thin lipid film (RE52-98 Shanghai Yarong Biochemistry Instrument Factory, Shanghai, China) under a water bath at 40°C for 30 min. The lipid film was exposed to vacuum at room temperature for 2 h to remove the residue of ether. The resultant lipid film was hydrated in phosphate buffered saline (PBS, pH 7.4) to prepare crude liposomes suspensions. Then, the expected sized liposomes suspensions were obtained from the crude liposomes suspensions using a homogenizer under certain pressures (NS1001L, Niro Soavi, Co., Italy).
Characterization
The different TFu-loaded nanocarriers were characterized with regard to morphology, particle size, zeta potential, and encapsulation efficiency.
Morphology examination
The morphology of nanocarriers was examined by transmission electronic microscopy (TEM) (JEM-1200EX, Japan).
Size and zeta potential measurements
The size distribution of nanocarriers was determined by photon correlation spectroscopy (PCS) with a Zetasizer 3000 (Malvern Instruments, Malvern, UK). All measurements were carried out in triplicate. The average particle size was expressed as volume mean diameter and the reported value was represented as mean ± SD (n = 3).
Entrapment efficiency (EE %)
The EE% of TFu to the nanocarriers was calculated from the concentration of the non-encapsulated drug. The separation of TFu-SLNs from the non-encapsulated TFu was performed by the cryogenic ultracentrifugation method (Shanghai Anting Scientific Instrument Ltd., China), whereas TFu-liposomes and free TFu were separated using a protamine aggregation method (CitationSun et al., 2006). Then, the concentration of TFu was measured at 257 nm by RP-HPLC using a SPD-10Avp Shimadzu pump and a LC-10Avp Shimadzu UV-VIS detector. Samples were chromatographed on a 4.6 mm × 250 mm reverse phase stainless steel column packed with 5 μm particles (Venusil XBP C-18, Agela, China) eluted with a mobile phase consisting of 50:50 (v/v) mixtures of acetonitrile and water at a flow rate of 1 ml/min. Quantification was achieved by comparison between observed peak area ratios of TFu of the samples and a calibration curve performed using the same conditions. Samples were performed in triplicate and the mean ± SD were recorded. The drug entrapment efficiency (EE%) and drug loading (DL%) were calculated from equations (1) and (2), respectively:
where Wfree is the analyzed weight of free drug in the supernatant; Wtotal the analyzed weight of drug in the NPs dispersions; Wlipids the total weight of lipids.
Oral bioavailability studies in mice
The process of oral bioavailability studies and the method of sample analysis were conducted according to the procedure previously described (CitationSun et al., 2008).
Caco-2 cell culture
The human colon adenocarcinoma cell line (Caco-2) was obtained from the American Type Culture Collection (Manassas, VA) and used between passages 25–35. Cells were cultured in MEM supplemented with 10% (v/v) FBS, 1% (v/v) L-glutamine, 1% (v/v) NEAA, and 1% antibiotic solution (1 × 104 UI/ml penicillin, 10 mg/ml streptomycin) in a humidified incubator 5% CO2/95% air atmosphere at 37°C. Cells were plated on 75 cm2 flask and the culture medium was changed every 2 days for ∼ 5–6 days until the cells reached ∼ 80–90% confluence. After the passage operation, the cells were seeded approximately at 2.5 × 105 cells per flask.
Determination of cell viability
The effect of the different nanocarriers on their in vitro biocompatibility was investigated at different concentrations using Caco-2 cell cultures. Briefly, the Caco-2 cells were seeded onto a 96-well plate (0.33 cm2/ well) at a density of 104 cells/cm2 for 5 days and until a cell monolayer was obtained. The cells were incubated with 100 μl of PBS containing different concentrations and composition of nanocarriers or without nanocarriers (control) at 37°C for 24 h. Sodium dedocyl sulfate (SDS) at 2% (w/v) was used as the positive control, and MEM served as the negative control. After 24 h, the supernatant was removed by aspiration and the effect of the different treatments on cell viability was assessed by a CCK-8 assay kit (CCK-8, Dojindo, Kumamoto, Japan) according to the manufacturer’s procedures, and the absorbance at 450 nm was measured using a microplate reader (Model 680, BIO-RAD, PA, USA). The cell viability was expressed as a percentage of the absorbance of cells treated with test samples compared to that of the negative control.
Caco-2 permeability studies
For the experiments, the cells were harvested with trypsin–EDTA and seeded onto polycarbonate membrane filters (0.4 μm pore size, 0.60 cm2 surface area, PICM 01250, Millicell, MA, USA) inside Transwell® cell culture chambers (Corning Costar, Cambridge, MA) at a density of 5 × 104 cells/cm2 in the cell culture medium. The culture medium (0.4 ml per insert and 0.6 ml per well) was replaced every 2 days for the first week and everyday thereafter. After 19–21 days in culture, the transepithelial electrical resistance (TEER) of monolayers was checked using a Millicell®-ER system (Millipore Corporation, Bedford, MA). Only the monolayers with a TEER values above 300 Ωcm2 were used for the following assays.
The Caco-2 cell monolayer permeability study was conducted as earlier described (CitationKorjamo et al., 2008). The transports of different formulations of TFu: free solution, cationic SLNs, anionic SLNs, and liposomes were studied in the apical to basolateral direction. The test solutions were diluted in MEM at 10 μg/ml TFu concentrations. Here it is necessary to explain that was diluted from 1 mg/ml of the TFu DMSO solution. The experiment was started by adding 0.4 ml of test solution at the apical side and 0.6 ml of HBSS at the basolateral side. Then inserts were incubated at 37°C and 0.2 ml samples were withdrawn from the receiver chambers at 15, 30, 45, 60, 90, and 120 min. TFu content was determined by LC-MS/MS.
The apparent permeability (Papp) of the monolayers was calculated according to the following equation:
where Papp is the apparent permeability coefficient; dQ/dt is the rate of appearance of TFu in the basolateral side (μg/s); A is the surface area of the monolayers (cm2) and C0 is the initial concentration of TFu in the apical side (μg/ml).
TFu analysis by LC-MS/MS
Liquid–liquid extraction was performed prior to the LC/MS in order to isolate the drugs from the transport buffer. Briefly, 1 ml acetic ether was added into 100 μl sample, followed by the addition of 10 μl glipizide as an internal standard dissolved in acetonitrile with a concentration of 1 μg/ml, then the mixture was vortexed for 3 min and centrifuged at 10,800 rpm for 5 min, and 900 μl of the supernatant was transferred to a clean test tube. After evaporation of the samples to dryness under nitrogen at 40°C, the residues were reconstituted in 100 μl acetonitrile.
The supernatants of 5 μl were analyzed by LC/MS (Agilent 1100 LC/MSD series) with a Diamonsil C18 column (5 μm, 150 × 4.6 mm; DIKMA, CA, USA). The mobile phase consisted of a gradient mixture of acetonitrile and 0.1% formic acid and was delivered at a flow rate of 0.8 ml/min. The retention times for TFu and the internal standard were 3.6 and 4.4 min, and the monitored m/z were 270.9 and 446.0 for TFu and glipizide, respectively. LC/MS analysis was carried out on an API-Electrospray SL (Applied Biosystems, Belgium) spectrometer and ionization was achieved using a turbo ion spray in positive ion mode. The detection wavelength was set at 257 nm and the linear dynamic range of the calibration curves ranged from 20–1600 ng/ml. The limit of detection was 5 ng/ml, and the limit of quantification was 10 ng/ml. The inter- and intra-batch accuracy was between 80–110% of the nominal value over the entire range. The precision was within 9% over the entire range.
Single-pass intestinal perfusion studies (SPIP) in rats
Male Sprague-Dawley rats weighing 250 ± 20 g were used for all perfusion studies, which were supplied by the Center of Experimental Animals, Shandong University, Ji’nan, China. All the animal experiments were conducted and the animals were housed and handled according to the requirements of the National Act on the Use of Experimental Animals (People’s Republic of China).
Perfusion solution
Krebs-Ringe perfusion buffer (K-R buffer solution containing 7.8 g NaCl, 0.35 g KCl, 1.37 g NaHCO3, 0.02 g MgCl2, 0.22 g NaH2PO4, and glucose in 1.48 g/1000 ml purified water, pH 6.5) was used as blank perfusion solution. The perfusate was prepared by dispersing the TFu nanosuspensions in the K-R buffer solution (20 mg TFu/100 ml) and installed in the cylinder, which was incubated in a 37°C water bath to maintain temperature.
SPIP experiment
The procedure for the in situ SPIP experiment followed previously published reports (CitationKim et al., 2006). Briefly, five male Sprague-Dawley rats were placed in individual cages and fasted overnight before the experiment with free access to water. After an overnight fast, rats were anesthetized with an intraperitoneal injection of ethylcarbamate (100 mg/100 g body weight), restrained in a supine position and kept at a body temperature of 37°C using infrared lamps. Upon verification of the loss of pain reflex, a mid-line abdominal incision of 3–4 cm was made and the whole small intestine was isolated and cannulated at both ends with two polypropylene tubes (3.2 mm diameter). The former tube was used as inlet and the latter as outlet. Then, it was first rinsed with 37°C physiological saline to clear the segment and purged by air, followed by being connected with the catheters to the perfusion system (BT00-100M, Baoding Longerpump Co. Ltd, China) with a 100 ml graduated cylinder as a simple device. Care was taken to avoid disturbance of the circulatory system, and drops of normal saline solution at 37°C were added onto the surgical area, which was then covered with wet pledget to avoid loss of fluid.
The isolated segment was rinsed with physiological saline to clean any residual debris until the out flowing perfusate became colorless. At the start of the study, the perfusion buffer containing sample solution was first perfused at a flow rate of 5 ml/min to ensure rapidly filling the segment and the time was set to zero with the immediate start of the perfusion and the solution volume in circulation was recorded as the 0 min volume. After ∼ 10 min, when steady-state condition was achieved, the flow rate was adjusted to 2.5 ml/min, and the outlet perfused samples were collected at pre-determined time intervals (0.25, 0.5, 0.75, 1.0, 1.5, 2.0, 2.5, 3.0, 4.0, 5.0, and 6.0 h) for 6 h. During 6 h perfusion period, at each time point, 1 ml of the sample solution was taken out, the solution volume in the cylinder was recorded, and 1 ml K-R perfusate solution with the same temperature was added in. Samples were frozen immediately and stored at −20°C until analysis. At the end of the experiment, the perfused intestinal segment was measured without stretching.
Before analysis, the samples were thawed at 25°C and treated with 9 ml methanol, and the mixture was treated in an ultrasonic water bath for 3 min and then centrifuged at 10,000 rpm for 10 min; 20 μl of the supernatant that was filtered through a 0.22 µm Phenomenex filter was injected into the RP-HPLC system for the determination of TFu.
Effects of different intestinal site on intestinal absorption
SPIP was performed in different intestinal segments (duodenal, jejunal, ileal, and colon segment) to test if the intestinal absorption of TFu-loaded nanocarriers could exhibit the characteristics of intestinal site dependence. The study followed the method described in CitationLi et al. (2009) and CitationDahan and Amidon (2009). Each 10 cm-long intestinal segment was isolated as follows: duodenum segment beginning from 1 cm distal to pylorus, jejunum segment beginning from 15 cm away from pylorus, ileum segment beginning at the site 20 cm upwards caecum, while segment colon beginning at the site 2 cm distal to cecum. The above segments were carefully cannulated and ligated with both the ends. Then, each segment was perfused with solution containing different TFu-loaded nanocarriers for 2 h. The experimental operation was carried out as described above.
Data analysis
The remaining drug (Xr) in each sample was calculated. The absorption rate constant (Ka) and the absorption half-life (t1/2) for the samples were calculated by the following equations:
where X0 is the amount of TFu in the perfusate at 0 h, and t was the pre-determined time-point.
Calculations were based on outlet perfusate steady-state concentrations achieved after ∼ 10 min. The steady-state intestinal effective permeability (Peff, cm/s) was calculated according to a parallel tube model (6) (CitationSong et al., 2006):
where Qin is the perfusion flow rate (0.25 ml/min), A is the mass transfer surface area within the intestinal segment assumed to be the area of a cylinder (2 πrL) with the length (L) (measured after 2 h) and radius (r) of 0.18 cm, Cin and Cout are the inlet and outlet solution concentrations, respectively.
Statistical analysis
Results were expressed as mean values ± SD. A Student’s t-test was used for statistical comparison/analysis. P < 0.05 was considered statistically significant.
Results
Physicochemical characteristics of TFu-loaded nanocarriers
The resulting physicochemical characteristics of different nanocarriers containing TFu are presented in . As shown in , similar particles size (150∼200 nm) and EE% (70∼85%) of different nanocarriers was obtained, the main difference was in the zeta potential.
Table 1. Physicochemical characteristics of different TFu-loaded nanocarriers.
Oral bioavailability of TFu-loaded nanocarriers
In order to elucidate the role of lipid-based nanocarriers in their ability to enhance the intestinal absorption of TFu, the mean concentration in the plasma of TFu following the oral administration of the different nanocarriers and the corresponding control (TFu aqueous suspension) were determined. The mean concentration in the plasma vs time profile of the TFu of these three different formulations and suspension at a dose of 100 mg/kg for mice are shown in . The results indicate that cationic SLNs, anionic SLNs, and liposomes could significantly enhance the relative bioavailability significantly in comparison with TFu aqueous suspension by up to 202.2 ± 3.5%, 209.2 ± 7.2%, and 211.4 ± 9.6%, respectively.
Cytotoxicity of the nanocarriers
Before the transport studies, the cellular toxicity of the different types of nanocarriers was evaluated. The evaluation of the cytotoxicity of the nanocarriers had two objectives: (1) to determine the concentrations that could interfere with the cellular metabolism by further studies in Caco-2, and (2) to obtain a preliminary estimation of the safety of these new formulations. The cytotoxicity result was shown in .
Figure 3. Cytotoxicity of TFu-loaded nanocarriers with different concentrations after 24 h of incubation with Caco-2 cells using the CCK-8 method (compared to the negative control). Each data was presented as mean ± SD (n = 6 in each group).
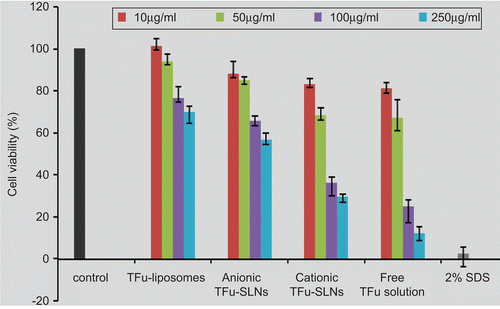
As shown in , the cytotoxicity of all the formulations showed concentration-dependent behaviors. TFu-liposomes showed high viabilities, even at concentrations as high as 250 μg/ml. On the other hand, TFu-SLNs exhibited certain toxicity at higher concentrations, especially the cationic one. This result can be attributed to the involvement of the surfactants, in the case of cationic TFu-SLNs the utilization of the CTAB resulted in higher cytotoxicity, which is in agreement with the findings of Liu et al. (2008). At concentration of 10 μg/ml, the cell viability of all the formulations was up to 80%. Therefore, 10 μg/ml was selected as the threshold of the nanocarrier concentrations for further Caco-2 experiments to ensure safety.
Caco-2 permeability studies
During the Caco-2 permeability studies, the apical to the basolateral transport of the TFu-free solution and the nanocarrier suspensions across the Caco-2 cell monolayers was shown in . As shown, the TFu-free solution exhibited a better linear fit (r = 0.993) over 120 min of the experiment, which indicated the transport process was a free diffusion. This may be attributed to keeping a soluble state in the free TFu solution with the help of 1% DMSO. Thus, the TFu molecule was small enough to diffuse through the aqueous pores at the tight junctions between cells, which are referred to as paracellular absorption. In contrast, TFu-loaded nanocarriers exhibited significantly different behaviors which, beginning with a fast rise then following a flat, was much closer to that of active transport. These results led us to the hypothesis that the TFu-loaded nanocarriers may exploit the way of transcellular absorption.
Figure 4. TFu transport across Caco-2 cell monolayers from the apical to basolateral from different formulations, the data were presented as mean ± SD (n = 6 in each group).
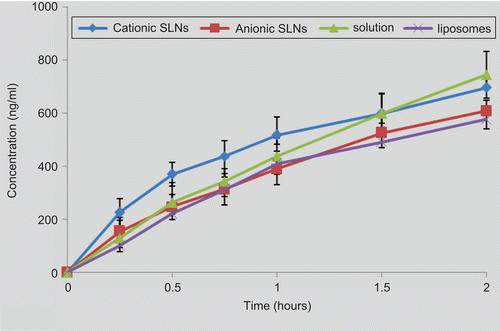
In addition, the Papp values were calculated and shown in . It was observed that both cationic SLNs (8.49 ± 1.6 × 10−6 cm/s) (p < 0.01) and anionic SLNs (7.89 ± 3.0 × 10−6 cm/s) (p < 0.05) could significantly enhance the transport of TFu compared with liposomes (5.99 ± 3.2 × 10−6 cm/s). Especially, cationic SLNs present much more effective capacity. Depending on their positively charged surface, cationic SLNs have a great affinity with the epithelial cells and could enhance the adsorption on the cell surface and the following endocytosis. This transport-enhancing effect was in good agreement with the finding that chitosan could enhance the permeability of the epithelial cells (Citationde Salamanca et al., 2006; CitationPrego et al., 2006). However, all nanocarrers presented significantly lower Papp values compared to the free TFu solution (10.62 ± 1.9 × 10−6 cm/s) (p <0.05), which probably transport the Caco-2 cell monolayers by free diffusion through the paracellular pathways.
Absorption property of TFu-loaded nanocarriers in rat intestine
The high correlation of rat Peff values with those of human was verified in the studies of CitationZakeri-Milani et al. (2007), in our present study, the SPIP of the TFu-loaded nanocarriers in rats was conducted to predict the human intestinal permeability and fraction absorbed. The SPIP procedure assumes that loss of drug during the perfusion is due to permeation of the intestine. Therefore, determination of the non-absorptive loss of perfused drug is required. Results of preliminary studies indicated that TFu is stable in the blank intestinal circulating solution, because the TFu concentration was 100.7 ± 2.1% of the original solution after incubation with the blank perfusion solution for 6 h at 37°C (n = 5). In addition, the rat’s intestine seems not to physically adsorb, as the TFu concentration was still 98.2 ± 2.8% of the original solution after it was incubated with a segment-cleaned blank intestine for 6 h at 37°C (n = 5). These results confirmed that the loss of TFu in the SPIP study could be attributed to the intestinal absorption.
Because some common used non-absorbed markers such as phenol red or radioactive isotope was demonstrated to be incompetent (CitationSutton et al., 2001), in order to overcome the disadvantages of the drug absorption in GI tract, we adopted a method of recording directly volume to determine the volume change of perfusate in intestinal perfusing experiment.
The intestinal absorption kinetics of TFu-loaded SLNs and liposomes were as follows: ln Xr-anionic SLN = 2.327–0.633t; ln Xr-cationic SLN = 1.872–0.429t, and ln Xr-liposome = 2.550–0.337t, respectively. The relative absorption parameters were calculated and shown in . It was found that both Ka and uptake percentage of these three different formulations follow a rank order: anionic SLN > cationic SLN > liposomes.
Table 2. The absorption parameters of different formulations following in situ single-pass rat intestinal perfusion after perfusing for 6 h.
Absorption of TFu-loaded nanocarriers in various intestinal segments of rats
In the present study, SPIP was performed in four different intestinal segments to test whether the intestinal absorption of the different TFu-loaded formulations exhibited site-dependent changes. The absorption percentages of TFu in different formulations at various intestine regions were shown in . In addition, The Peff values for each intestinal segment for different formulations were calculated from the steady-state concentrations of compounds in the perfusate collected from the outlet and summarized in . For the three formulations, different Peff values were obtained following the perfusion to the different segments, with the lowest permeability in the colon part. The results indicated that all the TFu-loaded formulations could be permeated and absorbed in all the segments of the intestine. The best absorbed segment in the intestine of the cationic SLNs, anionic SLNs, and liposomes was the jejunum, ileum, and jejunum, respectively.
Table 3. The permeability coefficient values (Peff, cm/s) following in situ single-pass rat intestinal perfusion to the different small intestinal regions.
Discussion
The measurement of the TEER is a common way to determine the paracellular permeability to ions and their changes upon exposure to a permeation enhancer. Reductions in the TEER can be correlated with partial disruption of the tight junction complex that restricts the paracellular route for the hydrophilic compounds. In the present study, the TEER values were measured before and after the examination and the result showed that there were no significant changes during the experiment (data not shown), which indicated that the Caco-2 monolayer was almost intact during the experiment and that none of the formulations studied containing TFu affected the integrity of the cell monolayer. It also suggested that the nanocarriers are non-toxic to the cells, which is in agreement with the results of the cytotoxicity studies considering the excipients employed in their preparation. Furthermore, the drug absorption by the paracellular pathway cannot be significant under these conditions. It is accepted that TFu-loaded nanocarriers could be transported across the monolayer by transcytosis mechanism, entering the Caco-2 cells at the apical surface of the monolayer by endocytosis (CitationPonchel et al., 1997). Thus, we can conclude that the main transport pathway of TFu across the Caco-2 monolayer could be attributed to the endocytic uptake of TFu-loaded nanocarriers and exocytic release of the nanoparticles by the Caco-2 cells rather than the enhanced diffusion of TFu through the perturbed tight junction between the cells (CitationYoo & Park, 2004).
The oral bioavailability results indicated the lipid-based nanocarriers including cationic SLNs, anionic SLNs, and liposomes could efficiently enhance the bioavailability of TFu in comparison with its aqueous suspension. However, there are no significant differences among them (p > 0.05), which is a little in contradiction with our original scheme that cationic SLNs should be more efficient than the anionic ones depending on their positive charged surface. It implicated that it is probably because the surface charge is not the key factor that determines their fate through the gastrointestinal tract because of its harsh environment. In order to understand how formulation design influences physicochemical properties and associated function in vivo, further investigations in both cell and animal levels were conducted.
Recent studies performed in the Caco-2 model cell lines have provided evidence on the ability of lipid-based nanocarriers to cross epithelia. Our present study focused on evaluation of TFu-loaded nanocarriers transport using different formulations of TFu across the Caco-2 cell culture model. We hypothesized that the increase of bioavailability provided by lipid based nanocarriers was mostly due to their capacity to enhance permeability across the intestinal barrier. Regarding the experiments carried out with nanoparticle lower Papp values were demonstrated for TFu from nanoparticle formulations compared to the free drug solution. These results are in agreement with the previous reports for famotidine-loaded microparticles (CitationDegim et al., 2005) and dexamethasone-loaded nanoparticle-coated microparticles (CitationBeck et al., 2007). In the previous studies, it was reported that overall ranking of compounds with Papp < 1 × 10−6 cm/s, between 1–10 × 10−6 cm/s, and > 10 × 10−6 cm/s can be classified as poorly (0–20%), moderately (20–70%) and well (70–100%) absorbed compounds, respectively (CitationYee, 1997). Based on this observation, it is suggested that TFu is moderately absorbed from the Caco-2 monolayers using lipid-based carriers. Cationic SLNs tend towards the higher range of absorption suggesting a moderate-to-high absorption behavior compared to anionic SLNs and liposomes. On the other hand, the Papp for anionic SLNs also demonstrated to be higher than that of liposomes, which was statistically significant (p < 0.05). This might be attributed to the lipid composition of SLNs, which could interact with the cell membrane and induce a series of reactions thereby increasing the paracellular permeation (CitationLindmark et al., 1998). Over all, in our study, SLNs exhibited more effective permeability than liposomes for TFu on Caco-2 cell monolayer.
However, although the Caco-2 cell model was widely used with in vitro assays to predict the absorption rate of candidate drug compounds across the intestinal epithelial cell barrier, lacking the function of secretion leads to the deficiency of the mucus layer unlike the rat intestine, even human intestine. There are still a great number of differences between the cell culture and intestine. In addition, the activity of the most important metabolizing enzyme in the human gut, CYP3A4, is low or absent in the Caco-2 monolayer (CitationUngell, 2004). Thus, in the interpretation of these results, we should be conscious of the fact that in these cell culture experiments an important amount of nanoparticles is forced to be directly in contact with the cells, a situation that differs substantially from that expected following oral in vivo administration (CitationGarcia-Fuentes et al., 2005).
In order to examine which SLNs formulation was more effective and to identify whether the above conclusion was also suitable in animal level meanwhile, we have conducted the SPIP study in rat intestine. Actually, SLNs could be absorbed more and faster in the rat intestine than liposomes, which is in accordance with the results of our previous studies on the Caco-2 cells and CitationMorishita and Peppas (2006). However, what contradicts the results obtained in Caco-2 cells study is that the anionic SLNs exhibited higher Ka and uptake percentage than cationic SLNs (p < 0.05) and liposomes (p < 0.05). It can be attributed to the structure of carriers and the physiological differences between cell culture and rat intestine. The cationic SLNs exhibit enhanced permeability in cellular level because nanoparticles could directly interact with cell membrane depending on their positive charge on surface and improve the following intracellular transport; however, in vivo intestine, thick mucus layer covering the whole GI tract, or other physiological barriers prevented nanocarriers interacted with cell membrane directly and hampered the absorption of the drug molecules after oral administration (CitationHunter and Hirst, 1997; CitationLennernäs, 1998; CitationGabor et al., 2004). By comparing the transmucosal behavior of these nanocarriers in vitro and in vivo, it was demonstrated that SLNs, including cationic and anionic SLNs, are capable of more enhanced transportation of TFu than liposomes both in vitro and in vivo. However, considering the limitation of the Caco-2 cell model, we have a conclusion that anionic SLNs were more effective in the transport of TFu and are worthy of being further researched and developed. We hope these results in our present study can provide evidence of the nanocarrier designation for their effective clinical application.
The occurrences of site-specific absorption in the GI tract also can be attributed to the differences in the composition of the carriers and the thickness of the mucus layer, pH, surface area, and enzyme activity (CitationHamman et al. 2005; Citation2007). In general, drug permeability is accepted to be higher in the upper region of the gastrointestinal tract compared to the lower parts because more intestinal villus is present in the duodenum and the jejunum than the other segments, thereby making them the best absorbed segments (CitationMasaoka et al., 2006). In our study, all three nanocarriers have good absorption in the jejunum (as shown in ). However, they have respective characters of absorption due to the differences in their composition and the intestine environment.
For the anionic SLNs and liposomes, the main absorption segments are the jejunum and ileum. The smaller size and inherent hydrophobicity of the nanoparticles favor absorption by the Peyer’s patches and Microfold Cells (M-cells) enriched in the jejunum and the ileum. In this way, they can be transported into lymphoid circulation without the addition of absorption enhancers (CitationKhoo et al., 2003; CitationPukanud et al., 2009). In addition, the regional membrane is lower than the upper parts of the gastrointestinal tract, which caused further improvement in the SLNs absorption. The duodenum showed a slightly lower absorption percentage in this study, which suggested the epithelial surface area was not a determinant of the intestinal permeability of the nanoparticles, as reported in the previous study (CitationMasaoka et al. 2006).
For the cationic SLNs, the main absorption segments were duodenum and jejunum. Although there are Peyer’s patches and M-cells in the ileum, the absorbed amount of cationic SLNs in the duodenum was more than double of that in the ileum. Therefore, the enhanced absorption of the cationic SLNs in the duodenum and the jejunum was attributed to the redundant policae circulane and the intestinal villi that greatly increase the surface area. These results suggest that the main mechanism of absorption for the cationic SLNs is the enhanced bioadhesion (CitationLiu et al., 2009).
Conclusion
In the present study, the potential of three different nanocarriers as oral delivery systems for N3-o-toluyl-fluorouracil (TFu) were evaluated. The interaction of the TFu-loaded nanocarriers with the Caco-2 cell model and the absorption in rat GI tract were investigated to define which formulation is worthy of being further researched and developed. By comparing the transmucosal behavior of these nanocarriers in vitro and in vivo, it was demonstrated that SLNs, including cationic and anionic SLNs, are capable of more enhanced transportation of TFu than liposomes. Furthermore, considering the results of the cytotoxicity study, the anionic SLNs were identified to be more effective in the transport of TFu and were worthy of being further researched and developed. We hoped these results in our present study could provide the evidence of the nanocarrier designation for their effective clinical application. However, due to the extreme complexities inherent in the study of the particle-uptake phenomenon and the mechanisms of interaction with the epithelium, studies that are more detailed are needed to fully understand the in vivo behavior of these lipid-based nanocarriers.
Declaration of interest
The authors report no conflicts of interest. The authors alone are responsible for the content and writing of the paper.
References
- Alonso, M.J. (2004). Nanomedicines for overcoming biological barriers. Biomed Pharmacother. 58:168–72.
- Alonso, M.J., Sánchez, A. (2004). Biodegradable nanoparticles as new transmucosal drug carriers. Chapter 20, pp 283–295. In: Sönke Svenson, ed. Carrier-based drug delivery. ACS Symposium Series. Washington, DC. Dendritic Nanotechnologies, Inc.
- Bai, F., Liu, C.X., Dai, L.P., Liu, L., Zhang, N. (2009). Preparation and pharmacokinetics in mice of N3-O-toluyl-flulorouracil solid lipid nanoparticles. Chin J New Drugs Clin Remedies. 28:185–90.
- Beck, R.C., Pohlmann, A.R., Hoffmeister, C., Gallas, M.R., Collnot, E., Schaefer, U.F., Guterres, S.S., Lehr, C.M. (2007). Dexamethasone-loaded nanoparticle-coated microparticles: correlation between in vitro drug release and drug transport across Caco-2 cell monolayers. Eur J Pharm Biopharm. 67:18–30.
- Dahan, A., Amidon, G.L. (2009). Segmental dependent transport of low permeability compounds along the small intestine due to P-glycoprotein: the role of efflux transport in the oral absorption of BCS class III drugs. Mol Pharm. 6:19–28.
- de Salamanca, A.E., Diebold, Y., Calonge, M., Garcia-Vazquez, C., Callejo, S., Vila, A., Alonso, M.J. (2006). Chitosan nanoparticles as a potential drug delivery system for the ocular surface: toxicity, uptake mechanism and in vivo tolerance. Invest Ophthalmol Vis Sci. 47:1416–25.
- Degim, Z., Unal, N., Essiz, D., Abbasoglu, U. (2005). Caco-2 cell culture as a model for famotidine absorption. Drug Deliv. 12:27–33.
- Desai, M.P., Labhasetwar, V., Walter, E., Levy, R.J., Amidon, G.L. (1997). The mechanism of uptake of biodegradable microparticles in Caco-2 cells is size dependent. Pharm Res. 4:1568–73.
- El-Shabouri, M.H. (2002). Positively charged nanoparticles for improving the oral bioavailability of cyclosporin-A. Int J Pharm. 249:101–8.
- Florence, A.T. (1997). The oral absorption of micro- and nanoparticulates: neither exceptional nor unusual. Pharm Res. 14:259–66.
- Gabor, F., Bogner, E., Weissenboeck, A., Wirth, M. (2004). The lectin-cell interaction and its implications to intestinal lectin-mediated drug delivery. Adv Drug Deliv Rev. 56:459–80.
- Garcia-Fuentes, M., Prego, C., Torres, D., Alonso, M.J. (2005). A comparative study of the potential of solid triglyceride nanostructures coated with chitosan or poly (ethylene glycol) as carriers for oral calcitonin delivery. Eur J Pharm Sci. 25:133–43.
- Hamman, J.H., Demana, P.H., Olivier, E.I. (2007). Targeting receptors, transporters and site of absorption to improve oral drug delivery. Drug Target Insights. 2:71–81.
- Hamman, J.H., Enslin, G.M., Kotze, A.F. (2005). Oral delivery of peptide drugs. BioDrugs. 19:165–77.
- Hunter, J., Hirst, B.H. (1997). Intestinal secretions of drugs: the role of P-glycoprotein and related drug efflux systems in limiting oral drug absorption. Adv Drug Deliv Rev. 25:129–57.
- Khoo, S.M., Shackleford, D.M., Porter, C.J.H., Edwards, G.A., Charman, W.N. (2003). Intestinal lymphatic transport of halofantrine occurs after oral administration of a unit-dose lipid-based formulation to fasted dogs. Pharm Res. 20:1460–5.
- Kim, J.S., Mitchell, S., Kijek, P., Tsume, Y., Hilfinger, J., Amidon, G.L. (2006). The suitability of an in situ perfusion model for permeability determinations: utility for BCS class I bio-waiver requests. Mol Pharm. 3:686–94.
- Korjamo, T., Heikkinen, A.T., Waltari, P., Monkkonen, J. (2008). The asymmetry of the unstirred water layer in permeability experiments. Pharm Res. 25:1714–22.
- Lennernäs, H. (1998). Human intestinal permeability. J Pharm Sci. 87:403–10.
- Li, H.L., Zhao, X.B., Ma, Y.K., Zhai, G.X., Li, L.B., Lou, H.X. (2009). Enhancement of gastrointestinal absorption of quercetin by solid lipid nanoparticles. J Contr Rel. 133:238–44.
- Lindmark, T., Kimura, Y., Artursson, P. (1998). Absorption enhancement through intracellular regulation of tight junction permeability by medium chain fatty acids in Caco-2 cells. J Pharmacol Exp Ther. 284:362–9.
- Liu, D.H., Liu, C.X., Zou, W.W., Zhang, N. (2010). Enhanced gastrointestinal absorption of N3-O-Toluyl-Fluorouracil by Cationic solid lipid nanoparticles. J Nanopart Res. 12:975–84.
- Liu, J., Xu, W.F., Zhou, Y., Yuan, Y.X., Qu, X.J. (2006). Effect of growth inhibition on tumour cells induced by N3-O-toluyl-fluorouracil. Chin Pharm J. 37:252–5.
- Luo, Y., Chen, D., Ren, L., Zhao, X., Qin, J. (2006). Solid lipid nanoparticles for enhancing vinpocetine’s oral bioavailability. J Contr Rel. 114:53–9.
- Masaoka, Y., Tanaka, Y., Kataoke, M., Sakuma, S., Yamashita, S. (2006). Site of absorption after oral administration: assessment of membrane permeability and luminal concentration of drugs in each segment of gastrointestinal tract. Eur J Pharm Sci. 29:240–50.
- Morishita, M., Peppas, N.A. (2006). Is the oral route possible for peptide and protein drug delivery? Drug Discov Today. 11:905–10.
- Muller, R.H., Runge, S., Ravelli, V., Mehnert, W., Thunemann, A.F., Souto, E.B. (2006). Oral bioavailability of cyclosporine: solid lipid nanoparticles (SLN) versus drug nanocrystals. Int J Pharm. 317:82–9.
- Nassar, T., Rom, A., Nyska, A., Benita, S. (2008). A novel nanocapsule delivery system to overcome intestinal degradation and drug transport limited absorption of P-glycoprotein substrate drugs. Pharm Res. 25:2019–29.
- Norris, D.A., Puri, N., Sinko, P.J. (1998). The effect of physical barriers and properties on the oral absorption of particulates. Adv Drug Deliv Rev. 34:135–54.
- Peira, E., Carlotti, M.E., Trotta, C., Cavall, R., Trotta, M. (2008). Positively charged microemulsions for topical application. Int J Pharm. 346:119–23.
- Ponchel, G., Montisci, M.J., Dembri, A., Durrer, C., Duchene, D. (1997). Mucoadhesion of colloidal particulate systems in the gastrointestinal tract. Eur J Pharm Biopharm. 44:25–31.
- Porter, C.J.H., Charman, W.N. (2001). Lipid-based formulations for oral administration: opportunities for bioavailability enhancement and lipoprotein targeting of lipophilic drugs. J Recept Signal Transduct Res. 21:215–57.
- Prego, C., Torres, D., Fernandez-Megia, E., Novoa-Carballal, R., Quinoa, E., Alonso, M.J. (2006). Chitosan-PEG nanocapsules as new carriers for oral peptide delivery-Effect of chitosan pegylation degree. J Contr Rel. 111:299–308.
- Pukanud, P., Peungvicha, P., Sarisuta, N. (2009). Development of mannosylated liposomes for bioadhesive oral drug delivery via M cells of Peyer’s patches. Drug Deliv. 16:289–94.
- Song, N.N., Li, Q.S., Liu, C.X. (2006). Intestinal permeability of metformin using single-pass intestinal perfusion in rats. World J Gastroenterol. 12:4064–70.
- Stewart, B.H., Chan, O.H., Lu, R.H., Reyner, E.L., Schmid, H.L., Hamilton, H.W., Steinbaugh, B.A., Taylor, M.D. (1995). Comparison of intestinal permeabilities determined in multiple in vitro and in situ models: relationship to absorption in humans. Pharm Res. 12:693–9.
- Sun, W.T., Huang, G.H., Ye, J.S., Zhang, N. (2006). Determination of encapsulation efficiencies of liposomes and nanoliposomes by protamine aggregation method. Chin Pharm J. 41:1716–20.
- Sun, W.T., Zhang, N., Li, A.G., Zou, W.W., Xu, W.F. (2008). Preparation and evaluation of N3-O-toluyl-fluorouracil-loaded liposomes. Int J Pharm. 353:243–50.
- Sutton, S.C., Rinaldi, M.T., Vukovinsky, K.E. (2001). Comparison of the gravimetric, phenol red, and 14C-PEG-3350 methods to determine water absorption in the rat single-pass intestinal perfusion model. AAPS Pharm Sci. 3:E25.
- Szakács, T., Veres, Z., Vereczkey, L. (2001). In vitro–in vivo correlation of the pharmacokinetics of vinpocetine. Pol J Pharmacol. 53:623–8.
- Ungell, A.B. (2004). Caco-2 replace or refine? Drug Discov Today Technol. 1:423–30.
- Yee, S. (1997). In vitro permeability across caco-2 cells (colonic) can predict in vivo (small intestinal) absorption in man—fact or myth. Pharm Res. 14:763–6.
- Yoo, H.S., Park, T.G. (2004). Biodegradable nanoparticles containing protein-fatty acid complexes for oral delivery of salmon calcitonin. J Pharm Sci. 93:488–95.
- Yuan, H., Chen, J., Du, Y.Z., Hu, F.Q., Zeng, S., Zhao, H.L. (2007). Studies on oral absorption of stearic acid SLN by a novel fluorometric method. Colloids Surf B Biointerfaces. 58:157–64.
- Zakeri-Milani, P., Valizadeh, H., Tajerzadeh, H., Azarmi, Y., Islambolchilar, Z., Barzegar, S., Barzegar-Jalali, M. (2007). Predicting human intestinal permeability using single-pass intestinal perfusion in rat. J Pharm Pharm Sci. 10:368–79.
- Zou, W.W., Sun, W.T., Zhang, N., Xu, W.F. (2008). Enhanced oral bioavailability and absorption mechanism study of N3-O-toluyl-fluorouracil-loaded liposomes. J Biomed Nanotechnol. 4: 90–8.