Abstract
Currently, repeated routine subcutaneous injections of insulin are the standard treatment for insulin-dependent diabetic patients. However, patients’ poor compliance for injections often fails to achieve the stable concentration of blood glucose. As a protein drug, the oral bioavailability of insulin is low due to many physiological reasons. Several carriers, such as macromolecules and liposomes have been used to deliver drugs in vivo. In this review article, the gastrointestinal barriers of oral insulin administration are described. Strategies for increasing the bioavailability of oral insulin, such absorption enhancers, enzyme inhibitors, enteric coatings are also introduced. The potential absorption mechanisms of insulin-loaded nanoparticles across the intestinal epithelium, including intestinal lymphatic route, transcellular route and paracellular route are discussed in this review. Natural polymers, such as chitosan and its derivates, alginate derivatives, γ-PGA-based materials and starch-based nanoparticles have been exploited for oral insulin delivery; synthetic polymers, such as PLGA, PLA, PCL and PEA have also been developed for oral administration of insulin. This review focuses on recent advances in using biodegradable natural and synthetic polymers for oral insulin delivery along with their future prospects.
Introduction
To be effective, an orally administered protein drug must transit along the gastrointestinal (GI) tract, pass through the mucus layer, traverse across the intestinal epithelium, enter into the portal vein and finally reach the systemic circulation (Chen et al., Citation2011).
However, oral administration of pure insulin showed less effect due to the following factors. Firstly, insulin can be easily degraded by the acidic conditions in stomach and the proteases existing in the GI tract. Secondly, insulin is a kind of hydrophilic protein with a molecular weight of about 6000 Da, and thus it is difficult for insulin to be encapsulated in hydrophobic carriers and the permeation of insulin through the intestinal epithelium is limited. Thirdly, because of the liver first-pass effect, the bioavailability of untreated insulin is extremely low (Sonaje et al., Citation2010a,Citationb,Citationc).
In order to solve these problems, a lot of work has been carried out to develop new oral insulin delivery systems. The loading efficiency of insulin on hydrophobic carriers could be improved by the addition of distearyldimethylammonium bromide or soybean phospholipid (Cui et al., Citation2006; Li et al., Citation2013). Other studies used enteric coatings (Zhao et al., Citation2013), enzyme inhibitors (Werle et al., Citation2007) and permeation enhancers (Degim et al., Citation2004; Zhang et al., Citation2011a,Citationb) to enhance the absorption of insulin. But most of the recent literatures concentrate on the novel drug delivery systems made of biodegradable macromolecular materials, which could be used to carry insulin. In this review, the attention has been focused on the use of biodegradable natural and synthetic polymers (Alonso, Citation2004).
The absorption of oral insulin
After the oral administration of insulin preparations, the drugs should firstly transport along the GI tract. However, the pH of stomach environment is 1.2–3.0 and the pH in intestine is 6.5–8.0. Such variations make it difficult for protein drugs, such as insulin to remain activity in GI tract (Wang & Zhang, Citation2012). Some pH-sensitive materials, including hydroxypropyl methylcellulose phthalate (HPMCP) (Maroni et al., Citation2009; Wang & Zhang, Citation2012; Wu et al., Citation2012a,Citationb,Citationc), polycarboxylates, polyaminomethacrylates (Eudragit®) (Wu et al., Citation2012a,Citationb,Citationc; Marais et al., Citation2013; Viehof et al., Citation2013) were used as enteric coatings. A previous study showed that HP55 (one kind of HPMCP) modifications were capable of protecting insulin against acidic gastrointestinal conditions and then dissolving in the small intestinal environment. This modification reduced the cumulative release rate of insulin in simulated gastric fluid from 33.24 to 15.87%, while cumulative release rates of insulin in simulated intestinal fluid increased from 15 to 58.06% after this modification (Zhao et al., Citation2013).
In addition to the acidic environment, insulin could also be hydrolyzed by the pepsins and other proteases in the GI tract. In a previous study, trypsin/chymotrypsin inhibitor aprotinin was used to protect insulin from degradation. Eight hours after oral administration of insulin-loaded chitosan–aprotinin conjugate, the mean blood glucose level decreased to 84 ± 6%, while the mean blood glucose level in the control group (rats received no treatment) increased to 121 ± 8% of the initial blood glucose level (Werle et al., Citation2007). However, using enzyme inhibitors may lead to serious side effects, such as pancreatic hypertrophy, impairs dietary protein digestion and some potentially harmful changes in body functions (Rekha & Sharma, Citation2013).
Before traveling across the intestinal epithelium, the drugs should firstly pass through the intestinal mucus layer. As a selective barrier which enables the exchange of nutrients, water, and small molecules while being impermeable to pathogens, mucus layer is negatively charged and renewed continuously (Cone, Citation2009). Generally speaking, molecules with neutral surfaces could cross the mucus layer easily, while positively charged molecules have a stronger attraction with mucus (des Rieux et al., Citation2013). Some bioadhesive materials were used to increase the intestinal retention time. Chitosan, a cationic polymer, was the most widely used due to its electrostatic interaction with negatively charged mucins (Sung et al., Citation2012). Some weakly anionic carboxyl-containing polymers, including poly(acrylic acid), poly(methacrylic acid), carboxymethylcellulose, sodium alginate were capable of forming hydrogen-bonds with mucins by binding their carboxylic segments to the oligosaccharide branches of mucins, which contribute to their mucoadhesiveness (Khutoryanskiy, Citation2011).
Three possible uptake routes in the small intestine
Mostly, there are three possible routes for the absorption of nanoparticles-based oral insulin delivery system (): the first, via the densely clustered M cells located on the surface of Peyer's patches (Lopes et al., Citation2014); the second, via transcellular route, which mainly absorbs lipophilic particles through the cell membrane of enterocytes; and the last, via paracellular avenues, through the tight junctions (TJs) between two adjacent enterocytes (Shahbazi & Santos, Citation2013).
Intestinal lymphatic route
As an alternative way for oral insulin delivery, the intestinal lymphatic pathway transports drugs directly to the vena cava, thus bypassing the first-pass effects and improving the bioavailability (Yun et al., Citation2013). Peyer’s patches act as an entrance for drug to the lymphatic vessels, via the M cell transportation of peptides from the intestinal lumen to the underlying lymphoid cells. Lymphatic pathway has been shown to function in the absorption of highly lipophilic compounds. According to a previous study, the liposomal formulation could facilitate the uptake of cefotaxime (a hydrophilic drug) through lymphatic absorption. The drug concentration in the lymph was higher than that in the plasma when administered via liposomal formulation. The bioavailability of oral cefotaxime-loaded liposomes increased about 2.7 and 2.3 times compared with its oral administration via aqueous solution and the physical mixture, respectively (Ling et al., Citation2006).
Transcellular route
Uptake of NPs via the transcellular pathway depends on their particle size and hydrophobicity. It is known that particles smaller than 300 nm could be uptaken by enterocytes (He et al., Citation2012), while particles larger than 500 nm were more likely to be absorbed in jejuna Peyer's patches (Bakhru et al., Citation2013). Han et al. (Citation2012) used insulin-loaded hyaluronic acid (HA) nanoparticles for insulin delivery through transcellular route. The plasma glucose levels of diabetic rats receiving oral administration of insulin-loaded HA nanoparticles (50 IU/kg) reduced by 24% in 2 h and 32–39% in during 3–8 h, while the group treated with the same dose of insulin solution showed no change in blood glucose (Han et al., Citation2012).
In general, bioadhesive materials showed non-specific interactions with mucus layers. Receptor-mediated transport via receptor-specific ligands modifications of insulin delivery carriers were used to enhance the absorption of oral insulin. Promising targets, such as enterocytes, mucus layers and M cells have been studied (des Rieux et al., Citation2013). Biotin (vitamin B7) (Zhang et al., Citation2014) and lectins, such as wheat germ agglutinin (WGA) (capable for binding to N-acetylglucosamine and sialic acid), tomato lectin, Ulex europaeus agglutinin1 have been exploited to modify the protein drug carriers (Kim et al., Citation2005; Zhang et al., Citation2005). Vitamin B12-coated nanospheres could also be used as target delivery carrier through VB12-IF-IF receptor mediated endocytosis of villous epithelium. Insulin-loaded dextran nanoparticles conjugated with VB12 derivatives showed profound (70–75% blood glucose reductions) and prolonged (54 h) anti-diabetic effects in STZ-diabetic rats (Chalasani et al., Citation2007a,Citationb). Goblet cell-targeting ligands, such as CSKSSDYQC peptide, which identified from a random phage-peptide library was found to have affinity with the goblet cells. After oral administration in diabetic rats, the CSKSSDYQC peptide modified insulin-loaded nanoparticles showed a better hypoglycemic effect with a 1.5-fold higher relative bioavailability compared with unmodified ones (Jin et al., Citation2012).
Paracellular route
As to hydrophilic molecules, the paracellular pathway is the only way for their uptake. However, the tight junctions are difficult for large molecules to pass through. Drugs are generally excluded from this pathway without the use of permeation enhancer (Chuang et al., Citation2013). As the decreasing of calcium ion concentration leads to the opening of the tight junctions, Ca2+ chelating agents, such as ethylene glycol tetraacetic acid (EGTA) (Chuang et al., Citation2013), diethylene triamine pentaacetic acid (DTPA) (Su et al., Citation2012), could enhance the paracellular permeability by reversible opening of the tight junctions. Caco-2 cell monolayers treated with EGTA or γ PGA–EGTA nanoparticles produced a significantly larger reduction in TEER compared to the control cells (Chuang et al., Citation2013). Since calcium ions are also very crucial to sustain the activity of many intestinal proteases, Ca2+ chelating agents could also be used as an enzyme inhibitor to protect insulin against intestinal proteases.
Bile salts, such as sodium glycocholate (Niu et al., Citation2011), sodium taurocholate (Degim et al., Citation2004) and sodium deoxycholate (Niu et al., Citation2012) were most widely used to modify insulin-loaded liposomes as a penetration enhancer. These might be attributed to the good deformability of liposomes containing bile salts (Hu et al., Citation2013). Niu et al. (Citation2012) compared the differences between liposomes containing sodium glycocholate, sodium taurocholate and sodium deoxycholate, and the highest oral bioavailability was observed in rats administrated with insulin-loaded sodium glycocholate (8.5 and 11.0% in non-diabetic and diabetic rats, respectively).
Other biocompatible polymers, including poly(acrylic acid) and chitosan, are capable of opening the tight junctions reversibly (Woitiski et al., Citation2011; Gao et al., Citation2013a,Citationb). Poly(acrylic acid) and its derivatives have shown ability in binding Ca2+ (Gao et al., Citation2013a,Citationb). Oral administration of insulin-loaded carboxymethyl cellulose/poly(acrylic acid) hydrogels (60 IU/kg) to diabetic rats reduced fasting plasma glucose levels to 72.4% within 6 h post-administration. The relative bioavailability of oral administration of the insulin-loaded poly(acrylic acid) hydrogels (6.59%) was 10 times higher than oral administration of free insulin solution (less than 0.5%) (Gao et al., Citation2013a,Citationb). However, the opening of the tight junctions might cause the absorption of bacterial toxins, leading to some safety problems (Chen et al., Citation2011). The approaches to improve the bioavailability of insulin are summarized in .
Table 1. Approaches to improve the bioavailability of insulin.
Natural polymers
Chitosan
Chitosan (CS) is a natural polysaccharide derived from the partial deacetylation of chitin, a biopolymer generally found in crustacean shells and insects. The primary building blocks of CS are glucosamine and N-acetyl-glucosamine. It is biocompatible, biodegradable and protective (Mukhopadhyay et al., Citation2013). The utilization of chitosan for oral insulin delivery is based on the mucoadhesive property and the ability to mediate the opening of the tight junctions reversibly (Jose et al., Citation2012, Citation2013) ().
Figure 2. Schematic illustrations of the opening of chitosan-mediated reversible tight junction (TJ) (Sung et al., Citation2012).
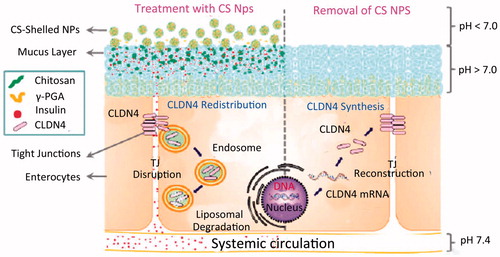
A recently published report studied an iron oxide–CS nanocomposite loaded with insulin. The iron oxide nanoparticles were obtained in chitosan solution by eco-friendly laser ablation technique. The insulin-loaded iron oxide-chitosan nanoparticles were found to effectively reduce the blood glucose level when administered orally in diabetic rats. About 51% blood glucose level reductions were achieved in severely diabetic rats (Kebede et al., Citation2013). This approach is promising, since the nanoparticles can be used in site-directed drug delivery due to its magnetic properties.
CS derivatives
The pKa of CS is approximately 6.5. CS is insoluble in neutral environment, where CS starts to lose charge, and this leads to the loss of its mucoadhesive properties and TJ opening activities (Qian et al., Citation2006). To overcome these drawbacks, several CS derivatives, including quaternized chitosan, thiolated chitosan, carboxylated chitosan and amphiphilic chitosan have been synthesized and evaluated for their potential in oral insulin delivery (Chen et al., Citation2013).
Quaternized chitosan can reserve its positive charge in neutral condition, which leads to a residence time increase and enhanced bioavailability (Sun & Wan, Citation2007). Sonia et al. (Citation2011) explored N-(2-hydroxyl) propyl-3-trimethyl ammonium chitosan chloride (HTCC) for oral insulin delivery. The results showed that quaternized chitosan had a stronger electrostatic interaction with mucus, and the percentage of adhesion was 95% for HTCC (the percentage of free amino groups was 36%) as compared to 72% for naive chitosan (the percentage of free amino groups was 78%). However, the high positive charge of quaternized CS derivatives may cause toxicity to cell membranes. Poly(ethylene glycol) (PEG) was used to modify N-Trimethyl chitosan chloride (TMC) in order to reduce the cytotoxicity. The hemolysis assay showed that TMC modified with PEG has a better biocompatibility than TMC without PEG modification (Prego et al., Citation2006; Zhu et al., Citation2007).
Thiolated chitosan have been proved to be more mucoadhesive compared with unmodified CS (Chen et al., Citation2013). The thiolated TMC, trimethyl chitosan-cysteine (TMC-Cys) was prepared to combine the mucoadhesion of TMC and the permeation enhancing abilities of thiolated polymers for oral insulin delivery. Compared to TMC nanoparticles, TMC-Cys nanoparticles significantly increased insulin absorption through rat intestine by 1.7 to 2.6-fold, and mucoadhesion and permeation enhancing effects of TMC-Cys nanoparticles were improved significantly (Yin et al., Citation2009). When comparing the mucoadhesion of different thiolated chitosans, it was found that Chitosan–thiobutylamidine exhibited the highest mucoadhesion, followed by Chitosan–4-mercaptobenzoic acid, Chitosan–glutathione, Chitosan–6-mercaptonicotinic acid, Chitosan–N-acetyl cysteine, Chitosan–thioglycolic acid and Unmodified chitosan (Mueller et al., Citation2011).
Previous research studied carboxylated chitosan by modifying chitosan with negatively charged carboxyl groups, in order to increase the water solubility of chitosan (Cui et al., Citation2007; Liu et al., Citation2013). In a previous study, carboxylated chitosan-grafted poly(methyl methacrylate) nanoparticles (CCGN) were prepared. The pharmacological bioavailability after oral administration of insulin-loaded CCGN at 25 IU/kg was 9.7%, while a long-lasting hypoglycemic effect could be observed after oral administration of insulin-loaded CCGN (Cui et al., Citation2009).
Amphiphilic CS derivatives, such as lauroyl sulphated chitosan (LSCS) were used for oral insulin delivery. It was showed that LSCS were non-toxic and lauroyl sulfated modification on chitosan improves mucoadhesion of CS greatly. Moreover, LSCS could also protect insulin from enzymatic degradation and reversely open the tight junctions (Shelma & Sharma, Citation2011).
Alginate derivatives
Alginate, an anionic mucoadhesive polysaccharide consisting of various ratios of β-d-mannuronopyranosyl and α-l-guluronopyranosyl units linked by (1→4)-O-glycosidic bonds, has always been used for preparing microparticles (Chan & Neufeld, Citation2010). It shows a property of mild gel-formation by ionically cross-linked with multivalent cations, such as calcium ions, thus enabling drug retention within the gel matrix (Sarmento et al., Citation2007a,Citationb). The large porosity of alginate beads often leads to low encapsulation efficiency and rapid release of the drug. Low drug encapsulation efficiency of alginates can be improved by the addition of chitosan and dextran sulfate (Martins et al., Citation2007; Reis et al., Citation2007; Sonia & Sharma, Citation2012).
Alginate/chitosan capsules were widely used for controlled release of oral protein drugs (Sarmento et al., Citation2007a,Citationb; Čalija et al., Citation2011; Zhang et al., Citation2011a,Citationb). Zhang et al. (Citation2011a,Citationb) encapsulated insulin in alginate–chitosan microspheres via different loading methods, and it was shown that the highest loading efficiency (56.7%) and remarkable activity maintenance (99.4%) were achieved when the insulin was loaded during the chitosan solidification process, while the network formation between chitosan and alginate-Ca microspheres greatly reduced the porosity and decreased the leakage of insulin.
γ-PGA-based materials
Poly-γ-glutamic acid (γ-PGA) is a water-soluble, biodegradable and non-toxic polymer. Many studies used nanoparticles composed of poly-γ-glutamic acid (γ-PGA) and CS for oral insulin delivery. With a smaller size and a higher loading efficiency compared with purely CS nanoparticles (Lin et al., Citation2007), the pH-sensitive CS/γ-PGA nanoparticles could resist gastric acid while released rapidly in certain area of the small intestine (Sonaje et al., Citation2010a,Citationb,Citationc). γ-PGA conjugated covalently by diethylene triamine pentaacetic acid (DTPA) could further prevent enzymolysis and prolong the residence time of the CS/γ-PGA-DTPA system for oral insulin delivery (Su et al., Citation2012).
Tripolyphosphate (TPP) and MgSO4 were added into CS/γ-PGA nanoparticles to form an ionically cross-linked network. Comparing with CS/γ-PGA nanoparticles, the multi-ion-cross-linked nanoparticles could retain more insulin. With the addition of MgSO4 and TPP, the cumulative amount of insulin released were significantly reduced at pH 2.5 and pH 7.0, while a fast release of insulin was observed at pH 7.4 (simulating the pH in the body fluid at intercellular spaces), which means insulin were more likely to be released from the multi-ion-cross-linked nanoparticles after they were infiltrated into mucus layer (Lin et al., Citation2008). Since SC injection of insulin often produces peripheral hyperinsulinemia in individuals. In another study, it was found that oral administration of aspart-insulin-loaded chitosan/γ-PGA nanoparticles could achieve a slower but longer hypoglycemic effect without producing hyperinsulinemia. Oral administration of aspart-insulin-loaded chitosan/γ-PGA nanoparticles at insulin doses of 30 IU/kg produced a hypoglycemic effect similar to subcutaneous injections of neutral protamine Hagedorn insulin (NPH-insulin) at insulin doses of 5 IU/kg. Moreover, the SC injection of aspart-insulin solution (5 IU/kg) produced a better hypoglycemic effect than SC injection of NPH-insulin solution with the same dose (Sonaje et al., Citation2010a,Citationb,Citationc) ().
Figure 3. (a) Blood glucose level versus time profiles and (b) plasma insulin level versus time profiles of test diabetic rats following the administration of different insulin formulations (Sonaje et al., Citation2010a,Citationb,Citationc).
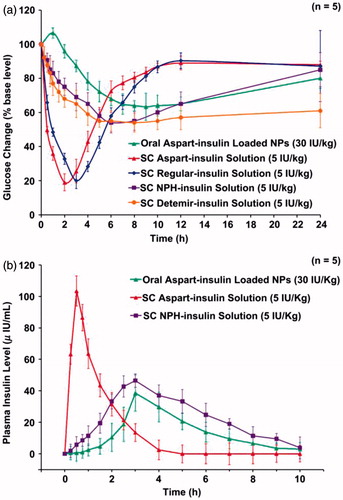
Starch-based nanoparticles
As one of the better known natural and biodegradable biopolymers, starch has attracted much attention because of its gelling and film forming properties. Zhang et al. (Citation2013) obtained a pH-responsive copolymer made of starch nanoparticles as backbone and poly(l-glutamic acid) (PGA) as graft chains. The result of in vitro insulin release experiment showed that the grafted copolymer had excellent pH-responsive property due to the introduction of pH-responsive PGA chain. Insulin released from the copolymer in artificial gastric juice (pH = 1.2) more slowly than that in artificial intestinal liquid (pH = 6.8). In another study, hydrophobic starch acetate was conjugated with polyethylene glycol (PEG) to from an amphiphilic polymeric derivative. Being pH-sensitive, the PEGylated starch acetate nanoparticles are capable of opening the tight junctions. Moreover, work of adhesion for PEGylated starch acetate nanoparticles was also significantly higher at 595.7 mN mm than starch acetate (179.1 mN mm) and chitosan (144.4 mN mm), which means PEGylated starch acetate nanoparticles showed a high mucoadhesiveness (Minimol et al., Citation2013).
Synthetic polymers
Since the natural polymers come from different preparation technologies and different sources, which may lead to a variety of physicochemical properties (Sonia & Sharma, Citation2012). However, the structure and physicochemical properties of synthetic polymeric carriers could be well-controlled, and thus their drug release properties and biological behaviors could be modified to change.
PLGA
Poly(lactide-co-glycolide) (PLGA) is widely studied for biological applications owing to their biodegradability and biocompatibility (Cui et al., Citation2007; Lassalle & Ferreira Citation2010; Reix et al., Citation2012). Generally speaking, the PLGA copolymers were synthesized from lactic acid and glycolic acid through the ring-opening polymerization. The hydrophobic interactions between insulin and PLGA appear to be responsible for the entrapment of insulin in PLGA nanoparticles (Lassalle & Ferreira, Citation2010). The contact between insulin and the short model of PLGA is illustrated in . However, the hydrophilicity of insulin makes them incapable of being entrapped by hydrophobic PLGA matrices. In a previous study, insulin was encapsulated in PLGA nanoparticles via double-emulsion solvent evaporation method to improve the loading capacity. 11.31% insulin loading capacity was obtained for the insulin-loaded PLGA nanoparticles. After oral administration of nanoparticles with an insulin concentration of 30 mg/kg to diabetic rats, a 50% reduction in blood glucose level was observed within 8 h (Yang et al., Citation2012).
Figure 4. (a) Short model of PLGA. (b) Interaction of the insulin with a PLGA model of near 1500 Da. In this figure the histidine are shown in space filling, the glutamate in ball and stick (Lassalle & Ferreira, Citation2010).
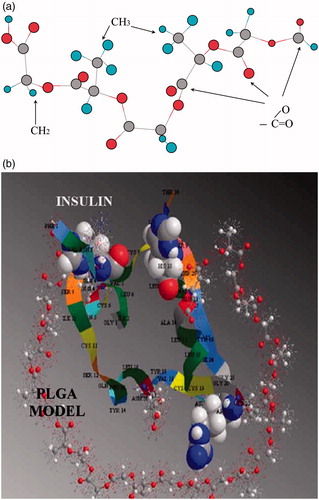
However, the slight negative surface charge of PLGA nanoparticles exhibit weaker bioadhesive abilities compared with positive-charged nanoparticles. Cationic modifications, such as chitosan-coat had been used to improve the bioavailability of insulin-loaded PLGA nanoparticles. As a result, compared with the PLGA nanoparticles, CS-coated PLGA nanoparticles reduced the initial burst, and showed a stronger mucosal adhesion, thus prolonged the resistance time and increased the bioavailability of insulin (Zhang et al., Citation2012). Concanavalin A was used as a target ligand to enhance the lymphatic absorption of oral insulin formulations. After oral administration of Concanavalin A-conjugated PLGA nanoparticles (20 IU/kg), the blood glucose lever reduced from about 260.17 to 196.33 mg/dL in 3 h, and hypoglycemic effect lasted for the next 24 h, while oral PLGA nanoparticles loaded with the same dose of insulin cannot reach the same effect (Hurkat et al., Citation2012).
PLA
Vesicles self-assembled from amphiphilic polymers are capable of encapsulating both hydrophilic and hydrophobic drugs due to their hydrophilic shell and hydrophobic core in aqueous solutions (Kita-Tokarczyk et al., Citation2005; Xiong, Citation2006). Xiong et al. explored the use of Pluronic/poly(lactic acid) (PLA) vesicles as oral insulin carriers. Pluronic/PLA copolymer was obtained by attaching PLA segments to both ends of Pluronic copolymer by a ring-opening polymerization (). PLA was highly hydrophobic, while the hydrophobicity of PPO was weaker and PEO was hydrophilic, so the Pluronic/PLA copolymer could self-assemble to vesicles in water ().
Figure 5 The synthesis scheme of PLA-P85-PLA block copolymer (Xiong et al., Citation2013).
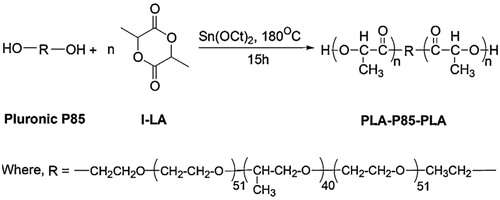
Figure 6. (a) TEM micrograph of PLA-P85-PLA nanoparticle vesicles in water. (b) The possible schematic microstructure of PLA-P85-PLA vesicles (Xiong et al., Citation2013).
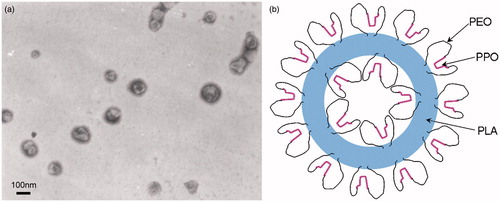
According to their studies, after orally treating with insulin-loaded PLA-F127-PLA vesicles at insulin doses of 50 IU/kg, the blood glucose of the diabetic mice reduced from 18.5 to 5.3 mmol within 4.5 h, and this effect lasted for an additional 18.5 h (Xiong et al., Citation2007). Pluronic P85/PLA (PLA-P85-PLA) vesicles were also explored to carry insulin for oral administration. After oral administration of insulin-loaded PLA-P85-PLA vesicles at insulin doses of 200 IU/kg to the diabetic mice, the glucose level reached the minimum after 2.5 h. The blood glucose level increased slowly after 10.5 h and was maintained at a low level (32.5% of initial blood glucose concentration) for at least an additional 14 h () (Xiong et al., Citation2013).
Figure 7. The blood glucose level versus time profiles following oral administration of physiological saline to control animals, insulin free PLA-P85-PLA vesicles, insulin-loaded PLA-P85-PLA vesicles (50, 100 and 200 IU/kg), and subcutaneous injection of free insulin (5 IU/kg). Results are means ± S.D. of five animals per group. Significant difference from negative physiological saline control (*p < 0.05; **p < 0.01) (Xiong et al., Citation2013).
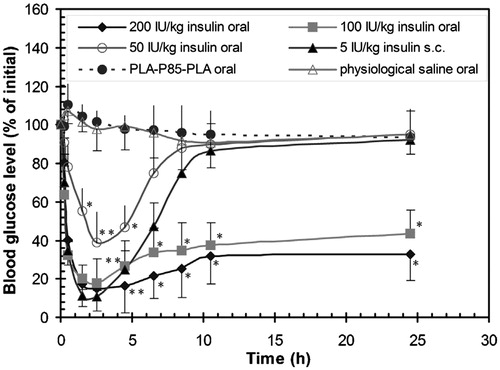
PCL
Poly(ɛ-caprolactone) is a biodegradable polyester synthesized fromɛ-caprolactone by a ring-opening polymerization (Jugminder & Chawla, Citation2002). Polymeric nanoparticles composed of biodegradable poly(ɛ-caprolactone) and polycationic non-biodegradable acrylic polymer (Eudragit® RS) were used for oral regular human insulin and aspart-insulin delivery. When aspart-insulin-loaded PCL/Eudragit® RS nanoparticles were administered orally to diabetic rats at the dose of 50 IU/kg, the maximum plasma glucose level reduction (−52%) was observed at 8 h after administration (Damge et al., Citation2010). Comparing with oral administration of regular insulin-loaded PCL/Eudragit® RS nanoparticles, which working only for 6–8 h, the hypoglycemic effects of aspart-insulin-loaded PCL nanoparticles lasted for 12–24 h (Damge et al., Citation2007). This may be because the monomeric aspart-insulin can be taken up by the intestinal mucosa more easily than regular human insulin (Damge et al., Citation2010).
PEA
l-Lysine/l-Leucine-based poly(ester amide) with pendant COOH groups was synthesized by He et al. (Citation2012) for oral insulin delivery. The PEA copolymer with benzyl ester pendants was prepared by solution polycondensation of three monomers, and the structure of l-Lysine/l-Leucine-based PEA-COOH is illustrated in (He et al., Citation2012). Insulin was encapsulated in PEA microspheres by a solid-in-oil-in-oil technique using N,N-dimethyformamide(DMF)/corn oil as solvent pairs. As a result, the release of insulin was pH-dependent and the hydrophobicity of PEA-COOH microspheres can be adjusted by the level of leucine components, thus improving the stability of the drug delivery system and enhancing the overall absorption of insulin. Oral administration of insulin-loaded PEA-COOH microspheres to streptozotocin-induced diabetic rats at a dose of 60 IU/kg, the plasma glucose levels reduced to 49.4% within the first 5 h of administration, and this effect lasted for 8 h (He et al., Citation2012). Arginine-based PEA (Arg-PEA) was also introduced in their research; the structure of Arg-PEA is shown in . By adding Arg-PEA to form PEA-COOH/Arg-PEA blend microspheres, the hypoglycemic effects could be further improved (He et al., Citation2013).
Figure 8 Chemical structures of the poly(ester amide)s. (a) l-Lysine/l-Leucine-based PEA-COOH. (b) Arginine-based Arg-PEA (He et al., Citation2013).
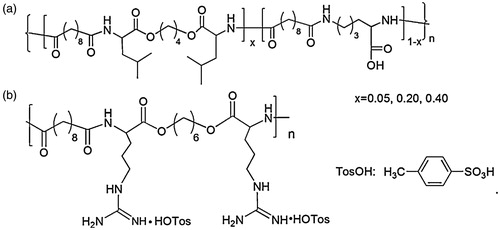
Conclusions and future perspectives
Though oral insulin delivery systems show promise in recent years, this work needs further study before clinical testing. Since insulin is a hydrophilic carrier, the insulin has been modified to enhance its physicochemical and biological stability. The loading efficiency of insulin should also be improved via different method (Li et al., Citation2013). However, the bioavailability of orally administered insulin remains low compared with SC injections. In addition, maintaining the physicochemical and biological stability of insulin in the GI tract is necessary, especially when mucoadhesive systems were used to prolong the residence time (Wong, Citation2010). Furthermore, potential insulin alternatives, such as psyllium polysaccharides (Singh & Chauhan, Citation2009), chalcone (Hsieh et al., Citation2012), glucagon-like peptide-1 (Ahn et al., Citation2013), can be added to the oral dosage form in order to avoid the risk of insulin overdose. A successful oral insulin delivery system should be biocompatible and biodegradable, keeping the stability of insulin during the absorption, and ultimately improving the bioavailability of insulin.
Declaration of interest
The authors would like to acknowledge the financial support from the National Natural Science Foundation of China (No. 21264009 and 31360376), the Natural Science Foundation of Jiangxi Province (No. 20132BAB206034), the Scientific and Technological Landing Project of Higher Education of Jiangxi Province (No. KJLD13071) and the Cultivation Project for Academic and Technical Leaders of Major Disciplines of Jiangxi Province.
References
- Ahn S, Lee IH, Lee E, et al. (2013). Oral delivery of an anti-diabetic peptide drug via conjugation and complexation with low molecular weight chitosan. J Control Release 170:226–32
- Alonso MJ. (2004). Nanomedicines for overcoming biological barriers. Biomed Pharmacother 58:168–72
- Bakhru SH, Furtado S, Morello AP, et al. (2013). Oral delivery of proteins by biodegradable nanoparticles. Adv Drug Delivery Rev 65:811–21
- Čalija B, Cekić N, Savić S, et al. (2011). An investigation of formulation factors affecting feasibility of alginate-chitosan microparticles for oral delivery of naproxen. Arch Pharmacal Res 34:919–29
- Chalasani KB, Russell-Jones GJ, Jain AK, et al. (2007a). Effective oral delivery of insulin in animal models using vitamin B12-coated dextran nanoparticles. J Control Release 122:141–50
- Chalasani KB, Russell-Jones GJ, Yandrapu SK, et al. (2007b). A novel vitamin B12-nanosphere conjugate carrier system for peroral delivery of insulin. J Control Release 117:421–9
- Chan AW, Neufeld RJ. (2010). Tuneable semi-synthetic network alginate for absorptive encapsulation and controlled release of protein therapeutics. Biomaterials 31:9040–7
- Chen MC, Mi FL, Liao ZX, et al. (2013). Recent advances in chitosan-based nanoparticles for oral delivery of macromolecules. Adv Drug Deliv Rev 65:865–79
- Chen MC, Sonaje K, Chen KJ, et al. (2011). A review of the prospects for polymeric nanoparticle platforms in oral insulin delivery. Biomaterials 32:9826–38
- Chuang EY, Lin KJ, Su FY, et al. (2013). Calcium depletion-mediated protease inhibition and apical-junctional-complex disassembly via an EGTA-conjugated carrier for oral insulin delivery. J Control Release 169:296–305
- Cone RA. (2009). Barrier properties of mucus. Adv Drug Delivery Rev 61:75–85
- Cui F, He C, Yin L, et al. (2007). Nanoparticles incorporated in bilaminated films: a smart drug delivery system for oral formulations. Biomacromolecules 8:2845–50
- Cui F, Shi K, Zhang L, et al. (2006). Biodegradable nanoparticles loaded with insulin-phospholipid complex for oral delivery: preparation, in vitro characterization and in vivo evaluation. J Control Release 114:242–50
- Cui FD, Tao AJ, Cun DM, et al. (2007). Preparation of insulin loaded PLGA-Hp55 nanoparticles for oral delivery. J Pharm Sci 96:421–7
- Cui FY, Qian F, Zhao Z, et al. (2009). Preparation, characterization, and oral delivery of insulin loaded carboxylated chitosan grafted poly(methyl methacrylate) nanoparticles. Biomacromolecules 10:1253–8
- Damge C, Maincent P, Ubrich N. (2007). Oral delivery of insulin associated to polymeric nanoparticles in diabetic rats. J Control Release 117:163–70
- Damge C, Socha M, Ubrich N, et al. (2010). Poly(epsilon-caprolactone)/eudragit nanoparticles for oral delivery of aspart-insulin in the treatment of diabetes. J Pharm Sci 99:879–89
- Degim Z, Unal N, Essiz D, et al. (2004). The effect of various liposome formulations on insulin penetration across Caco-2 cell monolayer. Life Sci 75:2819–27
- des Rieux A, Pourcelle V, Cani PD, et al. (2013). Targeted nanoparticles with novel non-peptidic ligands for oral delivery. Adv Drug Deliv Rev 65:833–44
- Gao X, Cao Y, Song X, et al. (2013a). Biodegradable, pH-responsive carboxymethyl cellulose/poly(acrylic acid) hydrogels for oral insulin delivery. Macromol Biosci 14:1–11
- Gao X, He C, Xiao C, et al. (2013b). Biodegradable pH-responsive polyacrylic acid derivative hydrogels with tunable swelling behavior for oral delivery of insulin. Polymer 54:1786–93
- Han L, Zhao Y, Yin L, et al. (2012). Insulin-loaded pH-sensitive hyaluronic acid nanoparticles enhance transcellular delivery. AAPS PharmSciTech 13:836–45
- He C, Yin L, Tang C, et al. (2012). Size-dependent absorption mechanism of polymeric nanoparticles for oral delivery of protein drugs. Biomaterials 33:8569–78
- He P, Liu H, Tang Z, et al. (2013). Poly(ester amide) blend microspheres for oral insulin delivery. Int J Pharm 455:259–66
- He P, Tang Z, Lin L, et al. (2012). Novel biodegradable and pH-sensitive poly(ester amide) microspheres for oral insulin delivery. Macromol Biosci 12:547–56
- Hsieh CT, Hsieh TJ, El-Shazly M, et al. (2012). Synthesis of chalcone derivatives as potential anti-diabetic agents. Bioorg Med Chem Lett 22:3912–15
- Hu S, Niu M, Hu F, et al. (2013). Integrity and stability of oral liposomes containing bile salts studied in simulated and ex vivo gastrointestinal media. Int J Pharm 441:693–700
- Hurkat P, Jain A, Jain A, et al. (2012). Concanavalin A conjugated biodegradable nanoparticles for oral insulin delivery. J Nanopart Res. doi: http://dx.doi.org/10.1007/s11051-012-1219-4
- Jin Y, Song Y, Zhu X, et al. (2012). Goblet cell-targeting nanoparticles for oral insulin delivery and the influence of mucus on insulin transport. Biomaterials 33:1573–82
- Jose S, Fangueiro JF, Smitha J, et al. (2012). Cross-linked chitosan microspheres for oral delivery of insulin: taguchi design and in vivo testing. Colloids Surf B 92:175–9
- Jose S, Fangueiro JF, Smitha J, et al. (2013). Predictive modeling of insulin release profile from cross-linked chitosan microspheres. Eur J Med Chem 60:249–53
- Jugminder S, Chawla MMA. (2002). Biodegradable poly(o-caprolactone) nanoparticles for tumor-targeted delivery of tamoxifen. Int J Pharm 249:127–38
- Kebede A, Singh AK, Rai PK, et al. (2013). Controlled synthesis, characterization, and application of iron oxide nanoparticles for oral delivery of insulin. Lasers Med Sci 28:579–87
- Khutoryanskiy VV. (2011). Advances in mucoadhesion and mucoadhesive polymers. Macromol Biosci 11:748–64
- Kim BY, Jeong JH, Park K, et al. (2005). Bioadhesive interaction and hypoglycemic effect of insulin-loaded lectin-microparticle conjugates in oral insulin delivery system. J Control Release 102:525–38
- Kita-Tokarczyk K, Grumelard J, Haefele T, et al. (2005). Block copolymer vesicles – using concepts from polymer chemistry to mimic biomembranes. Polymer 46:3540–63
- Lassalle V, Ferreira ML. (2010). PLGA based drug delivery systems (DDS) for the sustained release of insulin: insight into the protein/polyester interactions and the insulin release behavior. J Chem Technol Biotechnol 85:1588–96
- Li P, Nielsen HM, Fano M, et al. (2013). Preparation and characterization of insulin-surfactant complexes for loading into lipid-based drug delivery systems. J Pharm Sci 102:2689–98
- Lin YH, Chen CT, Liang HF, et al. (2007). Novel nanoparticles for oral insulin delivery via the paracellular pathway. Nanotechnology 18:1–11
- Lin YH, Sonaje K, Lin KM, et al. (2008). Multi-ion-crosslinked nanoparticles with pH-responsive characteristics for oral delivery of protein drugs. J Control Release 132:141–19
- Ling SS, Magosso E, Khan NA, et al. (2006). Enhanced oral bioavailability and intestinal lymphatic transport of a hydrophilic drug using liposomes. Drug Dev Ind Pharm 32:335–45
- Liu Y, Kong M, Feng C, et al. (2013). Biocompatibility, cellular uptake and biodistribution of the polymeric amphiphilic nanoparticles as oral drug carriers. Colloids Surf B 103:345–53
- Lopes MA, Abrahim BA, Cabral LM, et al. (2014). Intestinal absorption of insulin nanoparticles: contribution of M cells. Nanomedicine 10:1139–51
- Marais E, Hamman J, Plessis L, et al. (2013). Eudragit(R) L100/N-trimethylchitosan chloride microspheres for oral insulin delivery. Molecules 18:6734–47
- Maroni A, Del Curto MD, Serratoni M, et al. (2009). Feasibility, stability and release performance of a time-dependent insulin delivery system intended for oral colon release. Eur J Pharm Biopharm 72:246–51
- Martins S, Sarmento B, Souto EB, et al. (2007). Insulin-loaded alginate microspheres for oral delivery – effect of polysaccharide reinforcement on physicochemical properties and release profile. Carbohydr Polym 69:725–731
- Minimol PF, Paul W, Sharma CP. (2013). PEGylated starch acetate nanoparticles and its potential use for oral insulin delivery. Carbohydr Polym 95:1–8
- Mueller C, Verroken A, Iqbal J, et al. (2011). Thiolated chitosans: in vitro comparison of mucoadhesive properties. J Appl Polym Sci 126:5046–55
- Mukhopadhyay P, Sarkar K, Chakraborty M, et al. (2013). Oral insulin delivery by self-assembled chitosan nanoparticles: in vitro and in vivo studies in diabetic animal model. Mater Sci Eng C 33:376–82
- Niu M, Lu Y, Hovgaard L, et al. (2011). Liposomes containing glycocholate as potential oral insulin delivery systems: preparation, in vitro characterization, and improved protection against enzymatic degradation. Int J Nanomed 6:1155–66
- Niu M, Lu Y, Hovgaard L, et al. (2012). Hypoglycemic activity and oral bioavailability of insulin-loaded liposomes containing bile salts in rats: the effect of cholate type, particle size and administered dose. Eur J Pharm Biopharm 81:265–72
- Prego C, Torres D, Fernandez-Megia E, et al. (2006). Chitosan-PEG nanocapsules as new carriers for oral peptide delivery. Effect of chitosan pegylation degree. J Control Release 111:299–308
- Qian F, Cui FY, Ding J, et al. (2006). Chitosan graft copolymer nanoparticles for oral protein drug delivery: preparation and characterization. Biomacromolecules 7:2722–7
- Reis CP, Ribeiro AJ, Houng S, et al. (2007). Nanoparticulate delivery system for insulin: design, characterization and in vitro/in vivo bioactivity. Eur J Pharm Biopharm 30:392–7
- Reix N, Parat A, Seyfritz E, et al. (2012). In vitro uptake evaluation in Caco-2 cells and in vivo results in diabetic rats of insulin-loaded PLGA nanoparticles. Int J Pharm 437:213–20
- Rekha MR, Sharma CP. (2013). Oral delivery of therapeutic protein/peptide for diabetes – future perspectives. Int J Pharm 440:48–62
- Sarmento B, Ribeiro A, Veiga F, et al. (2007a). Alginate/chitosan nanoparticles are effective for oral insulin delivery. Pharm Res 24:2198–206
- Sarmento B, Ribeiro AJ, Veiga F, et al. (2007b). Insulin-loaded nanoparticles are prepared by alginate ionotropic pre-gelation followed by chitosan polyelectrolyte complexation. J Nanosci Nanotechnol 7:2833–41
- Shahbazi MA, Santos HA. (2013). Improving oral absorption via drug-loaded nanocarriers: absorption mechanisms, intestinal models and rational fabrication. Currt Drug Metab 14:28–56
- Shelma R, Sharma CP. (2011). Submicroparticles composed of amphiphilic chitosan derivative for oral insulin and curcumin release applications. Colloids Surf B 88:722–8
- Singh B, Chauhan N. (2009). Modification of psyllium polysaccharides for use in oral insulin delivery. Food Hydrocolloid 23:928–35
- Sonaje K, Chen YJ, Chen HL, et al. (2010a). Enteric-coated capsules filled with freeze-dried chitosan/poly(gamma-glutamic acid) nanoparticles for oral insulin delivery. Biomaterials 31:3384–94
- Sonaje K, Lin KJ, Wang JJ, et al. (2010b). Self-assembled pH-sensitive nanoparticles: a platform for oral delivery of protein drugs. Adv Funct Mater 20:3695–700
- Sonaje K, Lin KJ, Wey SP, et al. (2010c). Biodistribution, pharmacodynamics and pharmacokinetics of insulin analogues in a rat model: oral delivery using pH-responsive nanoparticles vs. subcutaneous injection. Biomaterials 31:6849–58
- Sonia TA, Sharma CP. (2011). In vitro evaluation of N-(2-hydroxy) propyl-3-trimethyl ammonium chitosan for oral insulin delivery. Carbohydr Polym 84:103–9
- Sonia TA, Sharma CP. (2012). An overview of natural polymers for oral insulin delivery. Drug Discov Today 17:784–92
- Su FY, Lin KJ, Sonaje K, et al. (2012). Protease inhibition and absorption enhancement by functional nanoparticles for effective oral insulin delivery. Biomaterials 33:2801–11
- Sun Y, Wan A. (2007). Preparation of nanoparticles composed of chitosan and its derivatives as delivery systems for macromolecules. J Appl Polym Sci 105:552–61
- Sung HW, Sonaje K, Liao ZX, et al. (2012). pH-Responsive nanoparticles shelled with chitosan for oral delivery of insulin: from mechanism to therapeutic applications. Acc Chem Res 45:619–29
- Viehof A, Javot L, Beduneau A, et al. (2013). Oral insulin delivery in rats by nanoparticles prepared with non-toxic solvents. Int J Pharm 443:169–74
- Wang XQ, Zhang, Q. (2012). pH-sensitive polymeric nanoparticles to improve oral bioavailability of peptide/protein drugs and poorly water-soluble drugs. Eur J Pharm Biopharm 82:219–29
- Werle M, Loretz B, Entstrasser D, et al. (2007). Design and evaluation of a chitosan-aprotinin conjugate for the peroral delivery of therapeutic peptides and proteins susceptible to enzymatic degradation. J Drug Target 15:327–33
- Woitiski CB, Sarmento B, Carvalho RA, et al. (2011). Facilitated nanoscale delivery of insulin across intestinal membrane models. Int J Pharm 412:123–31
- Wong TW. (2010). Design of oral insulin delivery systems. J Drug Target 18:79–92
- Wu ZM, Guo XD, Zhang LJ, et al. (2012a). Solvent mediated microstructures and release behavior of insulin from pH-sensitive nanoparticles. Colloids Surf B 94:206–12
- Wu ZM, Ling L, Zhou LY, et al. (2012b). Novel preparation of PLGA/HP55 nanoparticles for oral insulin delivery. Nanoscale Res Lett 7:299. doi:10.1186/1556-276X-7-299
- Wu ZM, Zhou L, Guo XD, et al. (2012c). HP55-coated capsule containing PLGA/RS nanoparticles for oral delivery of insulin. Int J Pharm 425:1–8
- Xiong XY, Li QH, Li YP, et al. (2013). Pluronic P85/poly(lactic acid) vesicles as novel carrier for oral insulin delivery. Colloids Surf B 111:282–8
- Xiong XY, Li YP, Li ZL, et al. (2007). Vesicles from pluronic/poly(lactic acid) block copolymers as new carriers for oral insulin delivery. J Control Release 120:11–17
- Xiong XY, Tam KC, Gan LH. (2006). Polymeric nanostructures for drug delivery applications based on pluronic copolymer systems. J Nanosci Nanotechnol 6:2638–50
- Yang J, Sun H, Song C. (2012). Preparation, characterization and in vivo evaluation of pH-sensitive oral insulin-loaded poly(lactic-co-glycolicacid) nanoparticles. Diabetes Obes Metab 14:358–64
- Yin L, Ding J, He C, et al. (2009). Drug permeability and mucoadhesion properties of thiolated trimethyl chitosan nanoparticles in oral insulin delivery. Biomaterials 30:5691–700
- Yun Y, Cho YW, Park K. (2013). Nanoparticles for oral delivery: targeted nanoparticles with peptidic ligands for oral protein delivery. Adv Drug Deliv Rev 65:822–32
- Zhang N, Ping QN, Huang GH, et al. (2005). Investigation of lectin-modified insulin liposomes as carriers for oral administration. Int J Pharm 294:247–59
- Zhang X, Ma GH, Su ZG, et al. (2011a). Novel poly(L-lysine) particles for gene delivery. J Control Release 152:184–6
- Zhang X, Qi J, Lu Y, et al. (2014). Biotinylated liposomes as potential carriers for the oral delivery of insulin. Nanomedicine 10:167–76
- Zhang X, Sun M, Zheng A, et al. (2012). Preparation and characterization of insulin-loaded bioadhesive PLGA nanoparticles for oral administration. Eur J Pharm Biopharm 45:632–8
- Zhang Y, Wei W, Lv P, et al. (2011b). Preparation and evaluation of alginate-chitosan microspheres for oral delivery of insulin. Eur J Pharm Biopharm 77:11–19
- Zhang Z, Shan H, Chen L, et al. (2013). Synthesis of pH-responsive starch nanoparticles grafted poly(l-glutamic acid) for insulin controlled release. Eur Polym J 49:2082–91
- Zhao X, Shan C, Zu Y, et al. (2013). Preparation, characterization, and evaluation in vivo of Ins-SiO(2)-HP55 (insulin-loaded silica coating HP55) for oral delivery of insulin. Int J Pharm 454:278–84
- Zhu S, Qian F, Zhang Y, et al. (2007). Synthesis and characterization of PEG modified N-trimethylaminoethylmethacrylate chitosan nanoparticles. Eur Polym J 43:2244–53