Abstract
This article describes the development and preliminary testing of a mobile robotic device to facilitate minimally invasive beating-heart intrapericardial intervention. The HeartLander robot will be introduced beneath the pericardium via subxiphoid incision, adhere to the epicardium, navigate to any location, and administer therapy under the control of the physician. As compared to current robotic cardiac surgical techniques, this novel paradigm obviates immobilization of the heart and eliminates access limitations. Furthermore, it does not require lung deflation and differential ventilation and thus could enable outpatient cardiac surgery. The current HeartLander prototypes use suction to maintain prehension of the epicardium and wire actuation to perform locomotion. A fiber optic videoscope displays visual feedback to the physician, who controls the device through a joystick interface. The initial prototype demonstrated successful prehension, turning, and locomotion on open-chest, beating-heart porcine models where the pericardium was removed (N = 3). A smaller second-generation prototype with an injection system demonstrated locomotion and myocardial injection of dye, both performed with the pericardium intact (N = 3). These trials illustrate the feasibility of using a miniature mobile robot to navigate upon the beating heart and perform intrapericardial therapy.
Introduction
Minimally invasive cardiac surgery is aimed at reducing morbidity by avoiding measures such as median sternotomy and cardiopulmonary bypass. The small incisions used in minimally invasive approaches make it difficult to manipulate with high dexterity and to gain access to certain areas of the heart. To date, much of the available manual instrumentation for minimally invasive surgery offers rather limited solutions to these problems in that it involves rigid endoscopes and end-effectors with a small number of degrees of freedom. Expensive multi-arm robot systems such as da Vinci (Intuitive Surgical, Sunnyvale, CA, USA) provide much of the needed dexterity for endoscopic heart surgery, but access remains problematic for certain areas such as the posterior wall of the left ventricle Citation[1].
The goal of avoiding cardiopulmonary bypass requires surgery on the beating heart, complicating matters even further. Instrumentation is needed that can provide stable manipulation of an arbitrary location on the beating epicardium Citation[2]. The most common approach is to locally immobilize the heart by pressure or suction, using devices such as the Endostab and endo-Octopus Citation[3], Citation[4]. However, the resulting forces exerted on the myocardium can cause changes in the electrophysiological and hemodynamic performance of the heart, and the need to avoid this risk has occasioned some discussion in the literature Citation[3]. As an alternative, several researchers in robot-assisted endoscopic surgery are investigating active compensation for heartbeat motion by visually tracking the epicardium and moving the tool tips accordingly, but this remains an open research problem Citation[5–8]. The motion of the beating heart is complex, and in addition to the challenges of modeling or tracking the heart surface, active compensation will require considerable expense for high-bandwidth actuation to manipulate in at least three degrees of freedom over a relatively large workspace Citation[5].
The need for immobilization or stabilization exists only because the tools are held by a surgeon or robot that is fixed to the table or standing on the floor. We have taken a different approach: rather than trying to immobilize the heart surface to stabilize it in the fixed frame of reference of a table-mounted device, the device itself is mounted on the moving reference frame of the beating heart. This is accomplished using a miniature two-footed crawling robotic device (HeartLander), designed to be introduced into the pericardium through a port, attach itself to the epicardial surface, and then, under the direct control of the physician, travel to the desired location for treatment (). The problem of the beating-heart motion is thus largely avoided by attaching the device directly to the epicardium, and the problem of access is resolved by incorporating the capability for locomotion.
Figure 1. The tethered crawling robot is introduced into the thoracic cavity through a small port below the sternum (green circle), avoiding the area occupied by the lungs (outlined by the dashed blue line). It attaches to the epicardial surface of the heart and travels to the desired location for therapy under control of the physician. [Color version available online]
![Figure 1. The tethered crawling robot is introduced into the thoracic cavity through a small port below the sternum (green circle), avoiding the area occupied by the lungs (outlined by the dashed blue line). It attaches to the epicardial surface of the heart and travels to the desired location for therapy under control of the physician. [Color version available online]](/cms/asset/b6c9be11-05b9-4dda-b3f7-d273a48f9abd/icsu_a_123002_f0001_b.jpg)
Improved access and precise manipulation are not the only benefits of this approach. Port access for minimally invasive cardiac surgery has usually been transthoracic, largely to facilitate the use of rigid endoscopes. This requires the deflation of the left lung, necessitating general endotracheal anesthesia and differential lung ventilation. However, there is a variety of procedures—some well established, others more innovative—that conceivably could be performed transpericardially, without invading the pleural space, if appropriate instrumentation were available. Some examples are cell transplantation Citation[9], gene therapy for angiogenesis Citation[10], epicardial electrode placement for resynchronization Citation[11], epicardial atrial ablation Citation[12], intrapericardial drug delivery Citation[13], and ventricle-to-coronary-artery bypass Citation[14].
The ability of HeartLander to move to any desired location on the epicardium from any starting point enables minimally invasive cardiac surgery to become independent of the location of the pericardial incision, allowing a subxiphoid transpericardial approach to be used for any intrapericardial procedure, regardless of the location of the treatment site. As a result, deflation of the left lung is no longer needed and it becomes feasible to use local or regional rather than general anesthetic techniques. This has the potential to open the way to ambulatory outpatient cardiac surgery Citation[15].
Two HeartLander prototypes have been constructed and preliminary tests have been performed with each device. During initial testing, the first prototype demonstrated its ability to navigate on the surface of an exposed beating porcine heart with the pericardium excised Citation[16]. The second prototype was tested on beating porcine hearts with intact pericardia. In addition to locomotion, the second prototype also performed myocardial injections of dye. This paper describes the design of the two prototypes, reviews the initial tests performed with the first prototype, and presents the results of the latest experiments performed with the new prototype.
Design
Under direct control of the surgeon, HeartLander is designed to attach itself to the epicardial surface and navigate to any desired location to administer therapy. The current HeartLander design is a crawling robot connected to supporting tabletop instrumentation via a tether 1 meter long. The crawling robot () consists of two body sections that can independently adhere to the epicardium and translate during locomotion. The supporting instrumentation includes motors for actuation, a pump to supply vacuum pressure, and a computer for visual feedback and control. This tethered design allows the therapeutic portion of the robot to be passive, lightweight, inexpensive, and therefore disposable in clinical use.
Figure 2. The first crawling robot prototype (upper: 18 mm tall, 14 mm wide) and second prototype (lower: 11 mm tall, 10 mm wide). The second prototype features a needle for myocardial injection, which is retracted during locomotion. The lines mark a 25.4-mm grid, and a standard pencil is included for scale.
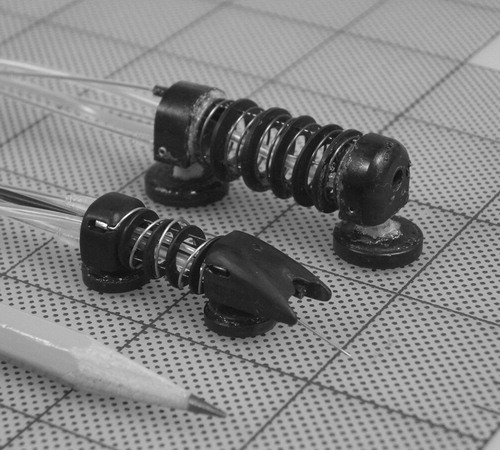
Prototypes
Thus far, two prototypes have been constructed and tested (). The first prototype has body sections that are 18 mm tall, which are mounted on suction pads of 14 mm diameter. The second prototype was designed to maneuver beneath the pericardium and was accordingly reduced in size and given a tapered front end. The body sections of this device are each 11 mm tall, with 10-mm diameter suction pads. Additionally, the second HeartLander prototype has the ability to perform myocardial injections using a custom 27 gauge needle, which passes through a sheath in the working channel. When the target area is located using video feedback, the physician advances the needle manually, through the sheath, into the tissue. The physician relies on visual feedback from the video monitor to judge the location and depth of the puncture. The injection is then performed using a syringe attached to the proximal end of the needle.
Insertion
A rigid subxiphoid videopericardioscope (SVP) will be used to access the heart and make a small opening in the pericardium Citation[17]. HeartLander will then be inserted directly onto the epicardial surface through the SVP working channel. The reduced size of the second prototype allows it to pass through a 14-mm channel. Once the treatment is complete, HeartLander will be retrieved by walking backwards or manually retracting the tether back through the SVP. Manual retraction also serves as the recovery method should the device become dislodged during the procedure.
Prehension
HeartLander adheres to the epicardium using suction. Suction has proven to be effective for epicardial prehension in surgical stabilizers such as the Octopus™ and Starfish™ (Medtronic, Minneapolis, MN) and in mobile robotic applications Citation[18]. Vacuum pressure from the external pump is supplied to the suction pads of the body sections through two vacuum lines that pass through the tether. The pump provides a vacuum pressure no greater than 0.053 N/mm2 (400 mmHg), which has been found to be effective and safe for use in FDA-approved cardiac stabilizers Citation[19]. Suction-based stabilizers are routinely used on ischemic epicardial tissue; nevertheless, should it become desirable to avoid traveling across certain regions of the epicardium, the visual feedback would allow the physician to make the necessary judgment and navigate accordingly. The bottom of each suction pad is covered with a mesh grate to protect the system from any biological matter that might clog the suction lines. During locomotion, the vacuum pressure is monitored by external pressure sensors and regulated by computer-controlled solenoid valves, both located in the supporting instrumentation.
Locomotion mechanics
The crawling robot of the current design is a hyperredundant continuum robot, meaning that it bends continuously along its length rather than at discrete joints. One translational and two rotational degrees of freedom are provided by three nitinol wires that pass through the tether and are actuated by motor belts in the supporting instrumentation. Each wire is enclosed by a low-friction plastic sheath, similar to those used in cable-driven transmissions. Rigid spacers between the front and rear body sections, uniformly separated by short spring segments, prevent the wires from bowing outward during turning (). The super-elasticity of nitinol allows the use of small-diameter wire (e.g., 0.15 mm) while avoiding permanent deformation. It also eliminates the need for additional shape-restoring components (e.g., springs) that are required in many cable-driven transmissions Citation[20]. The thermal shape memory property of nitinol is not used for actuation in this design. This remotely actuated continuum design allows HeartLander to be small, lightweight, and inexpensive. Additionally, the inherent compliance perpendicular to the longitudinal axis increases as the distance between the body sections increases during locomotion. This allows the advancing body section to safely contact and conform to the volatile surface of the heart without the need for expensive and complicated force feedback mechanisms Citation[20], Citation[21]. When the body sections are brought close together, this compliance diminishes greatly, thus providing a stable platform to administer therapy.
Figure 3. (a) Forward locomotion cycle of the robot (the bold ring indicates the body that is locked to the surface via suction). (b) Steering the robot. (c) The rigid spacer disks allow HeartLander to make sharp turns without the wires bowing. Two rotational degrees of freedom allow it to steer as well as to conform to curved surfaces (a 40-mm diameter table tennis ball shown here).
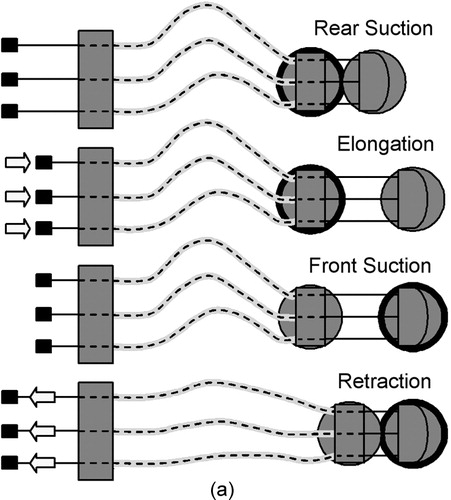
The locomotion of HeartLander is a cyclic, inchworm-like process controlled by the supporting computer with input from a joystick interface. One cycle of the process is schematically illustrated in . During elongation, the front body is advanced by pushing on the wires while the rear body is locked down via suction. During retraction, the rear body is advanced to meet the front body by pulling on the wires after the suction grip is transferred from the rear to front body. This locomotion scheme requires that some amount of slack be maintained in the tether and thus the tether must be made sufficiently long. Turning is achieved by differentially changing the lengths of the side wires, as illustrated in and .
Control
HeartLander is controlled by the physician using a computer-based graphical user interface (GUI) with a joystick input device and video feedback display ().
Figure 4. (a) The HeartLander control interface, including the joystick to control locomotion (and eventually therapy), and the monitor to display video from the device camera. (b) View of the left anterior descending artery (LADA) through the device camera. [Color version available online]
![Figure 4. (a) The HeartLander control interface, including the joystick to control locomotion (and eventually therapy), and the monitor to display video from the device camera. (b) View of the left anterior descending artery (LADA) through the device camera. [Color version available online]](/cms/asset/71736021-1f90-46e2-bb6c-f2ffdb1166e3/icsu_a_123002_f0004_b.jpg)
The joystick allows the physician to control the direction, length of step, and speed during locomotion. The four primary directions of the joystick (north, south, east, and west) correspond to hardcoded commands to move forward, move backward, turn right, and turn left. As the joystick is held in a single direction, the corresponding command is repeatedly issued until the mechanical constraints for elongation have been exceeded. At this point, retraction is automatically performed and control is returned to the user. This iterative, fine-stepping control mode can be bypassed by holding the trigger before moving the joystick, thus causing the robot to move the maximum distance for the corresponding command in a single motion. This allows the physician to travel in coarse steps when fine position control is not desired. Finally, the frequency (i.e., speed) of the stepper motor signals can be adjusted on the GUI, effectively adjusting the speed of the robot. The mechanical details of the locomotive process described in the previous section are completely controlled by the software, and thus are transparent to the physician.
The control software also ensures that at least one suction pad has a good grip of the epicardium at all times throughout the locomotive cycle. The vacuum seal at each suction pad is monitored using pressure sensors located in the supporting instrumentation. If the active suction pad does not achieve a good seal, likely due to the curvature of the heart surface, the software automatically adapts by ‘dithering’ the position of the top center wire until a seal is formed. This step-ensuring process is completely transparent to the user, except that joystick commands are ignored until it is safe to proceed with locomotion.
Visual feedback from the front body is relayed to an external video camera by a 1.6-mm-diameter flexible fiber optic endoscope running through the tether and displayed to the user on the monitor (). A mirror was used to angle the view of the scope toward the heart surface in the first prototype, but design modifications to the second prototype eliminated this requirement.
Testing
Large (30–45 kg) crossbred swine were used for all trials. After standard single-lumen endotracheal intubation, a surgical plane of anesthesia was maintained using isoflurane, 1–3%. Each animal was placed in the supine position, and median sternotomy was performed. Invasive hemodynamic and arterial blood gas monitoring was performed throughout the procedure.
Excised pericardium
The first prototype was tested during three porcine trials in which the pericardium was excised following median sternotomy Citation[16]. HeartLander was then placed directly on the epicardium manually. In each test, the device was able to maintain prehension of the exposed epicardium without being dislodged by the natural beating motion of the heart. Successful locomotion and steering were demonstrated across several surfaces with the heart held in various positions. Video recorded from the device fiberscope was displayed for the surgeon on the computer monitor and proved sufficient to identify certain landmarks. Locomotion was also captured on a handheld video camera as the device traveled across the surface of the heart, as shown in . Following all tests, the cardiac surgeon reported that no significant epicardial damage was detected; specifically, there were no signs of ischemia based on electrocardiographic monitoring. Superficial ecchymosis was noted after removal of the robot but it resolved within minutes, consistent with the clinical experience with FDA-approved suction-based positioners and stabilizers.
Intact pericardium
The second-generation prototype was tested during three porcine trials in which the pericardium was left intact following median sternotomy. HeartLander was introduced into the thoracic cavity through a 15-mm port (), simulating a subxiphoid incision, and applied to the epicardial surface through a 10-mm slit in the pericardium. The device was able to maintain prehension of the epicardium despite constant overhead contact with the pericardium. Locomotion was achieved across several surfaces including the anterior wall of the beating right ventricle, the anterolateral wall of the beating left ventricle, and the anterior wall of the left atrial appendage. The trials were recorded through the translucent pericardium using an external handheld video camera ().
Figure 6. A time sequence showing the second prototype walking on a beating porcine heart with the pericardium intact. The lower arrow indicates the location of the pericardial incision, and the upper arrow indicates the trailing edge of the device. The 15-mm port (green) can be seen in the upper-right corner. [Color version available online]
![Figure 6. A time sequence showing the second prototype walking on a beating porcine heart with the pericardium intact. The lower arrow indicates the location of the pericardial incision, and the upper arrow indicates the trailing edge of the device. The 15-mm port (green) can be seen in the upper-right corner. [Color version available online]](/cms/asset/6b4d05c9-f287-4945-aa17-959920579297/icsu_a_123002_f0006_b.jpg)
Myocardial injections of tissue-marking dye (0.5 cc) were performed at two locations: (1) the bifurcation of the left anterior descending coronary artery and the takeoff of the diagonal branch, and (2) the diagonal coronary artery. In each case, HeartLander walked to the planned site and locked down with suction on both pads, then the surgeon advanced the needle into the myocardium and performed the injection. The targets were visually located and recorded using the SVP, because of malfunctioning of the HeartLander visual system ( and ). The force required to advance the needle into the epicardium was 0.16 N, which is far less than the 4.48 N that are required to tangentially dislodge HeartLander from the heart surface (). No bleeding was observed following needle withdrawal. Confirmation of successful injection was made during postoperative examination of the excised porcine hearts ().
Figure 7. The second prototype (viewed beneath the pericardium by the SVP) (a) positioning for myocardial injection (needle highlighted by the arrow) and (b) performing a myocardial injection of tissue dye. (c) The injection sites viewed on the excised porcine heart. [Color version available online]
![Figure 7. The second prototype (viewed beneath the pericardium by the SVP) (a) positioning for myocardial injection (needle highlighted by the arrow) and (b) performing a myocardial injection of tissue dye. (c) The injection sites viewed on the excised porcine heart. [Color version available online]](/cms/asset/a161914a-0991-4a9b-8278-c616e592d11b/icsu_a_123002_f0007_b.jpg)
Table I. Force required to dislodge HeartLander from epicardium in porcine model in vivo when pulling on tether.
The force necessary to tangentially dislodge the device by pulling on the tether was recorded using a force gage. Three trials were performed for each of the following conditions: front suction only, rear suction only, and suction on both pads (). These measurements provide estimates of the shear forces that would be required for the device to become dislodged through contact with the pericardium.
During these experiments, we also demonstrated the feasibility of using manual reattachment if HeartLander should accidentally become rotated onto its side. With all suction turned off, the device was intentionally positioned on its side so the suction pads had no contact with the epicardium. The suction was then turned back on, and the proximal portion of the tether was rotated such that the device flipped back into its upright configuration. The suction pads regained contact with the epicardium and the device became securely reattached.
Following all procedures, as earlier, the cardiac surgeon confirmed that no damage was done to the epicardium or pericardium.
Discussion
The results presented herein demonstrate the feasibility of using a mobile crawling device to adhere to and maneuver on the epicardium of an exposed beating heart with the pericardium intact. Additionally, the injection of tissue-marking dye illustrates the ability to perform myocardial injections using the device.
Future research will focus on improvements in miniaturization and flexibility to allow the device to travel anywhere effectively on the heart during closed-chest testing. The size, shape, and rigidity of the first two prototypes prohibited travel to the posterior side of the heart, where the weight of the heart itself greatly increases the difficulty of mobility. To eliminate the rigidity of the fiberscope, an alternative video system will soon be implemented using an onboard camera. As new prototypes are developed, porcine tests will proceed from open-heart testing to closed-chest testing using a subxiphoid approach.
The body sections of each prototype include a 2-mm working port through which tools can be deployed for a variety of interventions. By employing a modular design for end-effector attachment and deployment, HeartLander will be capable of performing a variety of surgical treatments. The actuation for the end effectors will either be provided directly by on-board motors or transmitted from an external motor through the tether. Ultimately, we envision adoption of HeartLander-based intrapericardial therapies not only by cardiac surgeons but also by interventional cardiologists and electrophysiologists Citation[22], Citation[23].
Acknowledgements
Funding was provided by the US National Institute of Health (grant no. R01 HL078839), the National Science Foundation (grant no. EEC-9731748), and the National Aeronautics and Space Administration (grant no. NNG05GL63H). The authors wish to thank to Chee Kiat Ng and Eric Haas for engineering and programming assistance.
References
- Falk V, Diegler A, Walther T, Autschbach R, Mohr F W. Developments in robotic cardiac surgery. Curr Opin Cardiol 2000; 15: 378–387
- Zenati M A. Robotic heart surgery. Cardiol Rev 2001; 9: 287–294
- Falk V, Diegeler A, Walther T, et al. Endoscopic coronary artery bypass grafting on the beating heart using a computer enhanced telemanipulation system. Heart Surg Forum 1999; 2: 199–205
- Gründeman P F, Budde R, Beck H M, van Boven W-J, Borst C. Endoscopic exposure and stabilization of posterior and inferior branches using the endo-Starfish cardiac positioner and the endo-Octopus stabilizer for closed-chest beating heart multivessel CABG: hemodynamic changes in the pig. Circulation 2003; 108: 11–34
- Çavuşoğlu M C, Williams W, Tendick F, Sastry S S. Robotics for telesurgery: Second generation Berkeley/UCSF laparoscopic telesurgical workstation and looking towards the future applications. Ind Robot 2003; 30(1)22–29
- Ortmaier T J. Motion compensation in minimally invasive robotic surgery. Technical University of Munich, Germany 2003, Ph.D. dissertation
- Ginhoux R, Gangloff J, de Mathelin M, Soler L, Sanchez M A, Marescaux J. Active filtering of physiological motion in robotized surgery using predictive control. IEEE Trans Rob 2005; 21(1)67–79
- Nakamura Y, Kishi K, Kawakami H. Heartbeat synchronization for robotic cardiac surgery. Proceedings of ICRA 2001. IEEE International Conference on Robotics and Automation. 2001; 2: 2014–2019
- Li R-K, Jia Z-Q, Weisel R D, Merante F, Mickle D A G. Smooth muscle cell transplantation into myocardial scar tissue improves heart function. J Mol Cell Cardiol 1999; 31: 513–522
- Losordo D W, Vale P R, Isner J M. Gene therapy for myocardial angiogenesis. Am Heart J 1999; 138(2 Pt 2)S132–S141
- Leclercq C, Kass D A. Retiming the failing heart: principles and current clinical status of cardiac resynchronization. J Am Coll Cardiol 2002; 39: 194–201
- Lee R, Nitta T, Schuessler R B, Johnson D C, Boineau J P, Cox J L. The closed heart MAZE: A nonbypass surgical technique. Ann Thorac Surg 1999; 2: 1696–1702
- Gleason J D, Nguyen K P, Kissinger K V, Manning W J, Verrier R L. Myocardial drug distribution pattern following intrapericardial delivery: an MRI analysis. J Cardiovasc Magn Reson 2002; 4(3)311–316
- Boekstegers P, Raake P, Al Ghobainy R, Horstkotte J, Hinkel R, Sandner T, Wichels R, Meisner F, Thein E, March K, Boehm D, Reichenspurner H. Stent-based approach for ventricle-to-coronary artery bypass. Circulation 2002; 106: 1000–1006
- Zenati M A, Paiste J, Williams J P, Strindberg G, Dumouchel J, Griffith B P. Minimally invasive coronary bypass without general endotracheal anesthesia. Ann Thorac Surg 2002; 72: 1380–1382
- Riviere C N, Patronik N A, Zenati M A. A prototype epicardial crawling device for intrapericardial intervention on the beating heart. Heart Surg Forum 2004; 7(6)E639–E643
- Zenati M, Chin A, Schwartzman D (2004) Subxiphoid videopericardioscopy: a new minimally invasive approach to the pericardial cavity for epicardial therapies. Heart Surg Forum. Proceedings of ISMICS 7th Annual Scientific Meeting. June 23–26, 2004. 7: S26, (Suppl. 1)
- Siegel M, Gunatilake P, Podnar G. Robotic assistants for aircraft inspectors. Instrumentation Meas Mag 1998; 1(1)16–30
- Knight G, Fox W D, Schulze D R. Cardiac stabilizer device having multiplexed vacuum ports and method of stabilizing a beating heart. 2002, U.S. Patent 6,589,166
- Hirose S. Biologically Inspired Robots. Oxford Press;. 1993
- Gravagne I, Walker I D. On the kinematics of remotely-actuated continuum robots. IEEE Int'l Conf. on Robotics and Automation (ICRA), San Francisco, May, 2000
- Schweikert R A, Saliba W I, Tomassoni G, Marrouche N F, Cole C R, Dresing T J, Tchou P J, Bash D, Beheiry S, Lam C, Kanagaratna L, Natale A. Percutaneous pericardial instrumentation for endo-epicardial mapping of previously failed ablations. Circulation 2003; 108: 1329–1335
- Sosa E, Scanavacca M, D'Avila A, Oliveira F, Ramires J AF. Nonsurgical transthoracic epicardial catheter ablation to treat recurrent ventricular tachycardia occurring late after myocardial infarction. J Am Coll Cardiol 2000; 35: 1442–1449