Abstract
Cell cycle progression is dependent on the intracellular iron level and chelators can lead to iron depletion and decrease cell proliferation. This antiproliferative effect can be inhibited by exogenous iron. In this work, we present the synthesis of some new synthetic calix[4]arene podands bearing diamino-tetraesters, diamino-tetraalcohols, diamino-tetraacid and tetraaryloxypentoxy groups at the lower rim, designed as potential iron chelators. We report their effect on cell proliferation, in comparison with the new oral chelator ICL670A (4-[3,5-bis-(2-hydroxyphenyl)-1,2,4-triazol-1-yl]-benzoic acid). The antiproliferative effect of these new compounds was studied in the human hepatocarcinoma HepaRG cell cultures using cell nuclei counting after staining with the DNA intercalating fluorescence dye, Hoechst 33342. Their cytotoxicity was evaluated by the extracellular LDH activity. Preliminary results indicated that their antiproliferative effect was mainly due to their cytotoxicity. The efficiency of these compounds, being comparable to that of ICL670, was independent of iron depletion. This effect remains to be further explored. Moreover, it also shows that the new substituted calix[4]arenes could open the way to valuable new approaches for medicinal chemistry scaffolding.
Abbreviations: | ||
MEM, | = | minimum essential medium; |
LDH, | = | lactate dehydrogenase; |
DMSO, | = | dimethyl sulphoxide; |
PBS, | = | Phosphate-buffered saline. |
Introduction
Higher life forms such as humans have developed a highly efficient iron management system in which we absorb and only excrete about 1 mg of the metal daily and there is no mechanism for the excretion of excess iron [Citation1–2]. As a result, a progressive accumulation of iron in the body, particularly in the liver, leads to iron overload which is toxic and can induce hepatocellular carcinoma development as observed in genetic and secondary haemochromatosis [Citation3–4]. Iron plays a critical role in a variety of metabolic processes, as iron-containing proteins catalyse the key reactions involved in energy production and DNA synthesis. In particular, iron is critical for ribonucleotide reductase (RR) activity which is the rate-limiting step in DNA synthesis [Citation5]. RR comprises two subunits, R1 and R2. Iron is essential for the catalytic activity of RR and stabilises the tyrosyl radical located within the R2 subunit, making iron an obvious target for chemotherapeutic agents [Citation6]. Iron depletion by different iron chelators has been shown to inhibit the proliferation of various cell lines and normally activated lymphocytes in vitro [Citation7–9]. The iron depletion induced by iron chelators such as desferrioxamine (DFO) or O-Trensox decreases DNA synthesis in both normal and transformed hepatocytes [Citation10–11]. Numerous cancer cell types are more susceptible to the effects of chelators than normal cells due to a higher iron requirement for DNA synthesis and metabolism than the more slowly growing normal cells [Citation12]. Several studies have shown that iron is implicated in tumour cell growth and some tumour cells are sensitive to iron chelation therapy [Citation13–15]. The role of iron chelators has previously been reviewed [Citation16]. The effect of iron chelators is complex due to the numerous molecular targets in addition to RR and the molecular mechanisms involved in the G1/S arrest after iron deprivation remain poorly understood. Recent chelators such as 2-hydroxy-1-naphtylaldehyde isonicotinoyl hydrazone its analogues [Citation17–18] and Triapine [Citation19] have shown a greater anti-proliferative activity than DFO in vitro. However, DFO used for the treatment of iron overload, neuroblastoma and other diseases such as malaria [Citation15–16] is poorly absorbed by the gastrointestinal tract; furthermore, continuous exposure to DFO causes a dose and time-dependent cytotoxicity [Citation22]. Other disadvantages of DFO are its short plasma half-life and its poor permeability, which lead to poor antitumour activity [Citation23].Therefore, various new iron chelators have been designed for clinical use. Among them, the bidentate hydroxypyridinone deferiprone (CP20) which is the major molecule used for the treatment of secondary iron overload [Citation24–26], but this chelator has been shown to induce a severe neutropaenia [Citation27]. The orally active tridentate ICL670A (I, ) is of special interest [Citation21–22], because it induces a cell cycle arrest in the S phase associated with a decrease of the polyamine levels which could result from an inhibition of polyamine biosynthesis probably by ODC inactivation [Citation23].
Figure 1. Structures of ICL670 (I) and of our calix[4]arene derivatives (II–IV) previously described as antiproliferative compounds.
![Figure 1. Structures of ICL670 (I) and of our calix[4]arene derivatives (II–IV) previously described as antiproliferative compounds.](/cms/asset/c23411c3-ae66-49a5-88e7-f0433c8f65a7/ienz_a_489898_f0001_b.gif)
Clinically useful antitumour agents must show a significant therapeutic index (ie, they possess little effect on normal cells while inhibiting tumour cell growth). Richardson et al. have identified, in the case of pyridoxal isonicotinoyl hydrazone analogues, a significant chelator structure-activity relationship dependant on lipophilicity [Citation28]. Within a series or family of ligands, the compounds with the most marked lipophilic properties generally have the better iron-clearing efficiency. The lipophilicity has a profound effect on organ distribution of the chelator [Citation29]. Hider et al. have synthesised a family of hydroxypyridinone ester analogues which have been designed especially to target the hepatocellular low molecular weight iron pool [Citation30]. Chelators with high lipophilicity can easily enter cells and subsequently deplete iron from the intracellular pools necessary for iron incorporation into the R2 subunit. Another design consideration to identify an ideal iron chelator for antiproliferative activity is the metal selectivity [Citation31]. The chelators should be selective for iron to minimise chelation of other biologically metals or cations such as Zn(II) and Cu(II).
Calix[4]arenes or their derivatives have been tested as bioactive compounds such as antitumoural, antiviral, antimicrobial, anti-thrombotic and antifungal agents [Citation33–34]. Calix[4]arenes present some interesting lipophilicity properties and are selectively capable of binding cationic, anionic or neutral species. Consequently, some calixarene derivatives possess affinity and selectivity for Fe(III) and have a much lower affinity for Mg(II), Ca(II) and Zn(II) [Citation35–36]. Thus, taking into account our experience in the field of the synthesis of new compounds of the type calix[4]arenes [Citation37–39], we have previously prepared new calix[4]arene podands bearing at the lower rim two aspartic/glutamic acid or ornitine groups, mono hydrazidocalixarene and alkyl (ester or acid) [Citation40,Citation41]. In these series, three compounds II–IV exhibited an interesting antiproliferative activity in the rat hepatoma cell line Fao ().
In continuation of our work, we present here the synthesis and the antiproliferative activity in the HepaRG cell line culture of some new diamino-tetraesters, diamino-tetraalcohols, diamino-tetraacid, tetraaryloxypentoxy substituted calix[4]arene derivatives 1–8 ().
These functions were selectively introduced into the calix[4]arenes and locked in the cone conformation to improve their antiproliferative activity, as well as their chelator behaviour towards iron. The HepaRG cell line was isolated from a liver tumour of a patient suffering from a hepatocarcinoma [Citation42]. This bipotent cell line progressively differentiates in both hepatocyte-like cells expressing highly differentiated functions and also biliary-like cells. This cell line which was characterised through a transcriptomic approach exhibited an ability to store iron within the hepatocytes, when differentiated [Citation43]. Such a model gives us the opportunity to evaluate the impact of iron chelators on hepatocyte metabolism at various phases of their proliferation/differentiation process.
Materials and methods
Chemistry
Instrumentation
Column chromatography was performed on a Kielselgel 60 (40–63 μm) ASTM system(Merck). Reactions were analysed on precoated silica gel 60 F254 plates (Merck) and the compounds were visualised with a UV lamp (254 nm) or iodine vapour. Melting points were determined with an SM-LUX-POL Leitz hot-stage microscope and reported uncorrected. NMR spectra were recorded on a Bruker Avance 300 spectrometer. Chemical shifts refer to tetramethylsilane which was used as an internal reference. The J values are given in Hertz. Signal assignment was made using COSY, DEPT and heteronuclear single quantum coherence (HSQC) experiments when necessary.
N-3-Bromopropyl-N,N-bis(methoxycarbonylmethyl)amine 9
To a stirred solution of 3-bromopropylamine hydrobromide (5 g, 22.8 mmol) in acetonitrile (200 mL) cooled at −12°C, was added diisopropylethylamine (9.13 g, 70 mmol). Stirring was continued for five minutes before addition of methyl bromoacetate (7.3 g, 48 mmol) and the solution was kept at −10°C for two hours and then at room temperature for 18 hours. After concentration under reduced pressure, the resulting white solid residue was dissolved in water and extracted with methylene chloride. The organic phase was dried (MgSO4), filtered and concentrated under reduced pressure. The resulting oil was chromatographed over silica gel using dichloromethane/methanol (97/3) as eluant to give the title compound (5.58 g) as a light yellow liquid. Yield: 86%; 1H NMR (CDCl3): δ 2 (tt, J 6.6 Hz, 2H, BrCH2CH2), 2.9 (t, J 6.6 Hz, 2H, CH2N), 3.52 (t, J = 6.6 Hz, 2H, BrCH2), 3.67 (s, 4H, CH2CO2), 3.72 (s, 6H, CH3); 13C NMR (CDCl3): δ 30.93 (BrCH2CH2), 31.36 (BrCH2), 51.55 (CH3), 52.46 (CH2N), 54.87 (CH2CO2), 171.5 (CO2Me). Anal. Calcd. for C9H16BrNO4: C, 38.31; H, 5.72; N, 4.96. Found: C, 38.44; H, 5.97; N, 5.08.
25-[3-[Bis(methoxycarbonylmethyl)amino]propoxy]calix[4]arene, cone 1
To a mixture of calix[4]arene (1 g, 2.35 mmol), N-3-Bromopropyl-N,N-bis(methoxycarbonylmethyl)amine 9 (1.39 g, 4.94 mmol), moist potassium carbonate (0.39 g, 2.82 mmol based on anhydrous K2CO3) in acetonitrile (30 mL), was added a few mg of KI. The stirred mixture was boiled under reflux for 48 hours. The cooled mixture was concentrated under reduced pressure, and water was added to the resulting residue. The aqueous phase was then extracted with methylene chloride. The organic extracts were combined, dried (MgSO4) and the solvent removed in vacuo. The crude product was purified by column chromatography on silica gel eluting with hexane/ethyl acetate (1:1) to give 1 as oil which slowly crystallised. Yield: 17%, colourless crystals, mp = 164°C; 1H NMR (CDCl3): δ 2.31–2.4 (m, 2H, CH2CH2N), 3.3 (t, J = 6.9 Hz, 2H, CH2N), 3.49 (d, J = 13.2 Hz, 2H, ArCH2Ar), 3.51 (d, J = 13 Hz, 2H, ArCH2Ar), 3.75 (s, 6H, CH3), 3.76 (s, 4H, CH2CO2), 4.26 (t, J = 6.6 Hz, 2H, CH2O), 4.3 (d, J = 13 Hz, 2H, ArCH2Ar), 4.4 (d, J = 13.2 Hz, 2H, ArCH2Ar), 6.72 (t, J = 7.4 Hz, 3H, Ar-H para), 6.91 (t, J = 7.6 Hz, 1H, Ar-H para), 7.03–7.12 (m, 8H, Ar-H meta), 9.42 (s, 2H, OH), 9.71 (s, 1H, OH); 13C NMR (CDCl3): δ 28.25 (CH2CH2N), 31.31 and 31.81 (ArCH2Ar), 50.83 (CH2N), 51.55 (CH3), 54.66 (CH2CO2), 74.8 (CH2O), 120.82, 121.93, 126.13, 128.34, 128.7, 129.26, 128.22, 134.12 (Ar), 149.02 (Ar ipso), 150.74 (Ar ipso), 151.24 (Ar ipso), 171.7 (CO2Me). Anal. Calcd. for C37H39NO8: C, 71.02; H, 6.28; N, 2.24. Found: C, 71.33; H, 6.01; N, 2.18.
25,27-Bis-[3-[Bis(methoxycarbonylmethyl)amino]propoxy]calix[4]arene, cone 2
A mixture of calix[4]arene (1 g, 2.35 mmol), N-3-Bromopropyl-N,N-bis(methoxycarbonylmethyl)amine 9 (1.41 g, 5 mmol), dry potassium carbonate (0.41 g, 3 mmol), and a few mg of potassium iodide in acetonitrile (30 mL), was heated under reflux for 7 days. The white suspension was cooled to room temperature, filtered and the filtrate concentrated under reduced pressure. The crude residue was dissolved in a minimum amount of dichloromethane and applied to a silica gel column eluted with hexane/ethyl acetate (1:1) to give 2 as oil which slowly crystallised. Yield: 55%, colourless crystals, mp = 116°C; 1H NMR (CDCl3): δ 2.19 (m, 4H, CH2CH2N), 3.65 (dd, J = 6.3 Hz, 4H, CH2N), 3.4 (d, J = 13 Hz, 4H, ArCH2Ar), 3.66 (s, 12H, CH3), 3.71 (s, 12H, CH2CO2), 4.14 (dd, J = 6 Hz, 4H, CH2O), 4.34 (d, J = 13 Hz, 4H, ArCH2Ar), 6.68 (t, J = 7.5 Hz, 2H, Ar-H para), 6.7 (t, J = 7.5 Hz, 2H, Ar-H para), 6.89 (d, J = 7.5 Hz, 4H, Ar-H meta), 7.08 (d, J = 7.5 Hz, 4H, Ar-H meta), 8.09 (s, 2H, OH); 13C NMR (CDCl3): δ 28.17 (CH2CH2N), 31.37 (ArCH2Ar), 50.57 (CH2N), 51.45 (CH3), 54.38 (CH2CO2), 73.75 (CH2O), 119.04 (Ar para), 125.33 (Ar para), 128.17 (Ar ortho), 128.47 (Ar meta), 128.93 (Ar meta), 133.34 (Ar ortho), 151.81 (Ar ipso), 153.19 (Ar ipso), 171.78 (CO2Me). Anal. Calcd. for C46H54N2O12: C, 66.81; H, 6.58; N, 3.39. Found: C, 67.05; H, 6.72; N, 3.56.
25-[3-[Bis(2-hydroxyethyl)amino]propoxy]calix[4]arene, cone 3
The calixarene derivative 1 (140 mg, 0.22 mmol) in anhydrous THF (10 mL) was slightly warmed until complete dissolution. To the stirred solution was added lithium aluminohydride (42 mg, 1.11 mmol), and the mixture was heated at 90°C for 12 hours. After cooling to room temperature, the mixture was hydrolysed with water (5 mL) then filtered and the filtrate concentrated under reduced pressure. The resulting white solid was dissolved in water and extracted with methylene chloride. The organic phase was dried (MgSO4), filtered and concentrated under reduced pressure. The resulting oil was chromatographed over silica gel using dichloromethane/methanol (95/5) as eluant to give 3. Yield: 70%, white amorphous solid, mp = 122°C; 1H NMR (CDCl3 + MeOD): δ 2.29 (m, 2H, CH2CH2N), 2.86 (dd, J = 5.1 Hz, 4H, NCH2CH2OH), 3.11 (dd, J = 7.3 Hz, 2H, CH2N), 3.41 (d, J = 13.2 Hz, 4H, ArCH2Ar), 3.71 (m, 4H, NCH2CH2OH ), 3.87 (large s, 5H, OH), 4.11 (m, 2H, CH2O), 4.14 (dd, J = 6 Hz, 4H, CH2O), 4.18 (d, J = 13.6 Hz, 2H, ArCH2Ar), 4.26 (d, J = 13.2 Hz, 2H, ArCH2Ar), 6.6 (m, 3H, Ar-H para), 6.77 (t, J = 7.6 Hz, 1H, Ar-H para), 6.92–7.02 (m, 8H, Ar-H meta). Anal. Calcd. for C35H43NO6: C, 73.27; H, 7.55; N, 2.44. Found: C, 73.46; H, 7.27; N, 2.4.
25,27-Bis-[3-[Bis(2-hydroxyethyl)amino]propoxy]calix[4]arene, cone 4
The calixarene derivative 2 (0.8 g, 0.96 mmol) was dissolved in anhydrous THF (20 mL) and cooled in an ice bath. To the stirred solution, lithium aluminohydride (0.18 g, 4.8 mmol) was added in small portions over 20 minutes, and stirring continued at room temperature for 30 minutes, then heated under reflux for a further 3 hours. After cooling to room temperature, the mixture was hydrolysed with ice water, then filtered and the filtrate concentrated under reduced pressure. The resulting solid was dissolved in ethyl acetate (30 mL), the solution washed with water and the product extracted with ethyl acetate. The organic phase was treated with HCl 1M (20 mL), and extracted again with ethyl acetate. The combined organic extract were washed with brine, dried (MgSO4) and the solvent removed in vacuo to give a white amorphous solid (0.63 g) crystallised from methanol/chloroform (1:1), and then chromatographed over silica gel using dichloromethane/methanol (9/1) as eluant to give a mixture of compounds 3 (Rf = 0.4) and 4 (Rf = 0.2). Yield: 14%, colourless oil; 1H NMR (CDCl3): δ 2.27–2.31 (m, 4H, CH2CH2N), 2.82–2.85 (m, 8H, NCH2CH2OH), 3.1–3.12 (m, 4H, NCH2), 3.41 (d, J = 12.9 Hz, 4H, ArCH2Ar), 3.62–3.79 (m, 8H, NCH2CH2OH), 3.81 (large s, 4H, OH), 4.05–4.08 (m, 4H, CH2O), 4.27 (d, J = 12.9 Hz, 4H, ArCH2Ar), 6.69 (t, J = 7.5 Hz, 2H, Ar-H para), 6.74 (t, J = 7.5 Hz, 2H, Ar-H para), 6.88 (d, J = 7.5 Hz, 4H, Ar-H meta), 7.09 (d, J = 7.5 Hz, 4H, Ar-H meta), 7.72 (s, 2H, OH); Anal. Calcd. for C42H54N2O8: C, 70.56; H, 7.61; N, 3.92. Found: C, 70.34; H, 7.84; N, 3.76.
25,27-Bis-[3-[Bis(hydroxycarbonylmethyl)amino]propoxy]calix[4]arene, cone 5
To a stirred mixture of calix[4]arene derivative 3 (0.5 g, 0.6 mmol) in methanol (25 mL) was added a 4M solution of aqueous sodium hydroxide (1.8 mL). The mixture was boiled under reflux for 24 hours. After cooling to room temperature, the solvent was removed under reduced pressure. The resulting residue was dissolved in a few mL of water which was acidified to pH 1 with an ice-cold solution of 1M HCl to give a white precipitate. After filtration and washing with water until neutrality, the residue was dried in an oven overnight (50°C) to give 5. Yield: 83%, light green amorphous solid; mp = 246°C; 1H NMR (DMSO-d6): δ 2.04–2.11 (m, 2H, OCH2CH2), 3.14 (t, J = 5.4 Hz, 2H, CH2N), 3.12–3.16 (m, 2H, CH2N), 3.41 (d, J = 12.9 Hz, 4H, ArCH2Ar), 4.04 (t, J = 5.4 Hz, 4H, CH2O), 4.21 (d, J = 12.9 Hz, 4H, ArCH2Ar), 6.58 (t, J = 7.2 Hz, 2H, Ar-H para), 6.77 (t, J = 7.8 Hz, 2H, Ar-H para), 7.04 (d, J = 7.8 Hz, 4H, Ar-H meta), 7.13 (d, J = 7.8 Hz, 4H, Ar-H meta), 8.49 (s, 2H, OH). Anal. Calcd. for C42H50N2O12: C, 65.1; H, 6.5; N, 3.61. Found: C, 64.95; H, 6.32; N, 3.52.
25,26,27,28-Tetra[5-(4-cyanophenoxy)pentoxy]calix[4]arene, cone 6
To a solution of calix[4]arene (1.31 g, 3 mmol) in DMF (60 mL) was slowly added NaH (0.74 g of 60% dispersion in mineral oil, 18.5 mmol). The reaction mixture was then stirred at room temperature for 1 h. Then 4-[(5-bromopentyl)oxy]benzonitrile (5 g, 18.5 mmol) was added and stirring was continued for 4 days. After that acetic acid (0.8 mL) was added to neutralise the excess of NaH. The reaction mixture was diluted with water (60 mL), and the product was extracted with dichloromethane (2 × 60 mL). The organic layer was washed with water, dried over Na2SO4 and the solvent was evaporated under reduced pressure. The residue was purified by column chromatography in dichloromethane to give 6. Yield: 66%, white crystals, mp = 71°C; IR νmax (KBr)/cm−1 2220 (C≡N); 1H NMR (CDCl3): δ 1.56–1.62 (m, 8H, CH2), 1.85–1.91 (m, 8H, CH2), 1.98–2.05 (m, 8H, CH2), 3.21 (d, J = 13.35 Hz, 4H, ArCH2Ar), 3.93–4.02 (m, 16H, OCH2), 4.45 (d, J = 13.35 Hz, 4H, ArCH2Ar), 6.62–6.64 (m, 12H, Ar-H meta and Ar-H para), 6.89 (d, J = 9 Hz, 8H, H-2' or H-6'), 7.54 (d, J = 9 Hz, 8H, H-3' and H-5'). Anal. Calcd. for C76H76N4O8: C, 77.79; H, 6.53; N, 4.77. Found: C, 77.92; H, 6.64; N, 4.63.
25,26,27,28-Tetra[5-(4-amidinophenoxy)pentoxy]calix[4]arene tetrahydrochloride, cone 7
Into a 50 mL dry reaction flask charged with 1M LiN(SiMe3)2 in anhydrous THF (3.5 mmol) was added calix[4]arene 6 (0.85 g, 0.72 mmol) in 10 mL of THF, and the reaction mixture was kept stirring at room temperature overnight. The reaction mixture was then cooled to 0°C, to which was added HCl saturated ethanol (100 mL) whereupon a precipitate started forming. The crude mixture was kept at 4°C overnight. The precipitated product was then filtered, washed with diethyl ether to yield 7. Yield: 82%, white crystals, mp = 81°C; IR νmax (KBr)/cm−1 1640 (C=N); 1H NMR (DMSO-d6): δ 1.51–1.54 (m, 8H, CH2), 1.74–1.77 (m, 8H, CH2), 1.90–1.93 (m, 8H, CH2), 3.15 (d, J = 13.65 Hz, 4H, ArCH2Ar), 3.82–3.89 (m, 8H, OCH2), 3.95–4.01 (m, 8H, OCH2), 4.31 (d, J = 13.65 Hz, 4H, ArCH2Ar), 6.55–6.62 (m, 12H, Ar-H meta and Ar-H para), 6.95 (d, J = 8.7 Hz, 8H, H-prime or H-6'), 7.65 (d, J = 8.7 Hz, 8H, H-3' and H-5'), 9.23 (bs, 12H, NH2/NH.HCl). Anal. Calcd. for C76H92Cl4N8O8: C, 65.79; H, 6.68; N, 8.08. Found: C, 65.95; H, 6.76; N, 8.29.
25,26,27,28-Tetra{5-[4-(N-hydroxyamidinophenoxy)pentoxy]}calix[4]arene, cone 8
A mixture of hydroxylamine hydrochloride (0.24 g, 3.41 mmol) in anhydrous DMSO (5 mL) was cooled to 5°C under nitrogen and potassium t-butoxide (0.38 g, 3.41 mmol) was added in portions. The mixture was stirred for 30 min, then calix[4]arene 6 (0.2 g, 0.17 mmol) was added. The reaction mixture was stirred overnight at room temperature and then poured slowly onto ice-water. The precipitate was filtered and washed with water, ethanol then petroleum ether to afford 8. Yield: 67%, white crystals, mp = 180°C; IR νmax (KBr)/cm−1 3460 (NOH), 3360 and 3230 (NH2), 1640 (C=N); 1H NMR (DMSO-d6): δ 1.48–1.51 (m, 8H, CH2), 1.7–1.72 (m, 8H, CH2), 1.88–1.9 (m, 8H, CH2), 3.12 (d, J = 12.75 Hz, 4H, ArCH2Ar), 3.82–3.88 (m, 16H, OCH2), 4.31 (d, J = 12.75 Hz, 4H, ArCH2Ar), 5.7 (bs, 8H, NH2), 6.5 (t, J = 7.6 Hz, 4H, Ar-H para), 6.57 (d, J = 7.6 Hz, 8H, Ar-H meta), 6.78 (d, J = 8.4 Hz, 8H, H-2' or H-6'), 7.51 (d, J = 8.4 Hz, 8H, H-3' and H-5'), 9.45 (s, 4H, OH). Anal. Calcd. for C76H88N8O12: C, 69.92; H, 6.79; N, 8.58. Found: C, 70.11; H, 6.58; N, 8.74.
Pharmacology
Cell cultures
The HepaRG cells which were obtained from a liver tumour (hepatocarcinoma) from a female patient were cultured as previously described [Citation42]. They were maintained in William’s E medium supplemented with 10% fetal bovine serum, 100 U/ ml penicillin, 100 µg/ml streptomycin, 5 × 10−5 M hydrocortisone hemisuccinate and 5 µg/ml insulin. Cells were seeded at 2 × 104 cells/cm2 in 96-well microplates for the LDH and cell nuclei counting measurements.
Cell treatments
The various derivatives were compared to the tridentate hydroxyphenyltriazole ICL670 (Deferasirox, Exjade™) (I, ) [Citation44]. Stock solutions of each molecule (10 mM) were prepared in DMSO. The solubility of each derivative in the culture medium for the concentration range 0–400 µM was preliminarily verified by turbidimetry measurement. Three controls were used for each experiment: one with the standard culture medium, and the other with the culture medium supplemented with DMSO at the concentration used for ICL670 and calix[4]arenes tests. The DMSO supplemented controls lower than at a concentration of 1% DMSO did not show any differences with the standard medium controls. Therefore only the results obtained with controls without the DMSO have been reported.
Solubility of the chelators
The solubility of the new calix[4]arenes were estimated in phosphate buffer saline (PBS) solution and in the cell culture medium containing 10% FCS. Solutions of the various compounds (400, 200, 100, 50, 25 μM) were prepared in 96-well microplates by diluting the 10 mM stock solutions in DMSO in 200 μL of PBS and culture medium. The absorbance (turbidity) of the solutions was measured at 590 nm, out of the absorption range of the chromophores. The results were expressed as percentage of absorbance (turbidity) with respect to the values in the absence of test compound.
Comparison of chelator efficiency in aqueous phase
The cellular labile iron pool (LIP) is a pool of chelatable and redox-active iron, which plays a key role as a crossroads of cell iron metabolism. The ability of iron chelators to mobilise this temporary iron pool bound to low-molecular weight and low-iron affinity chelators (citrate, ascorbate, phosphate and adenosine triphosphate) is an essential factor influencing their biological efficiency. So, an acellular calcein test was performed to compare the potential ability of the various compounds and ICL670 as a reference, to compete for this chelatable iron pool in a physiological medium. In solution, calcein, a fluoresceinated analog of EDTA, binds Fe(II) and more slowly Fe(III). The pFeIII value of calcein, which corresponds to the negative logarithm of the free Fe(III) concentration at pH 7.4 and in the presence of Fe(III) and calcein concentrations of 1 and 10µM, respectively, was previously reported to be 20.3 [Citation45]. The fluorescence of this metallo-sensor dye is quenched during its interaction with iron and conversely is restored during the removal of iron from the [calcein-Iron] complex by various chelators. The rate and extent of the fluorescence recovery depends on the chelator concentration, the kinetic and stoichiometry of iron binding and the relative binding affinity. This metal chelating dye, mainly used to estimate cellular iron level was previously shown to be oxidatively degraded by Fe(II) in a H2O2-dependant pathway [Citation46]. Therefore we checked first that the calcein-Fe(III) complex, which probably involves also ferric hydroxide and ferric oxide interactions, is not degraded in our experiment conditions (4 hour in HEPES buffer at pH 7.3, data not shown). Fluorescence (λExc=485nm, λEm=520nm) of calcein (100 nM) in an HEPES buffer (20 mM HEPES, 150 mM NaCl, pH 7.3) was measured at room temperature in a microplate fluorescence reader (Packard, Fusion™ ), equipped with an orbital stirring. The Fe(III) (1 µM) slowly reacted with calcein and the maximal quenching of its fluorescence was observed for a time in excess of 6 hours. The fluorescence recovery was monitored after 4 hours incubation in the presence of various chelator concentrations. The results were expressed as a percentage of the fluorescence recovery with respect to the free calcein fluorescence intensity measured in the absence of iron.
Cytostatic and cytotoxic effects measurements
The chelator exposure experiments were performed one day after the cell seeding in the proliferating HepaRG cells. After 72h incubation at 37°C, the cell supernatants were collected for cytotoxicity measurement by measuring the extracellular LDH activity (cytotoxicity detection kit (LDH), Roche, Penzberg, Germany). The extracellular LDH activity was measured as described by the manufacturer on a 20 μL aliquot of the cell free medium obtained by centrifugation (2500 rpm/min during 5 min). The LDH activities were detected by reading the absorbance at 485 nm. They were reported as a percentage of extracellular LDH activity with respect to the control value. The data were the mean of three independent measurements.
The cell viability was determined by counting the cell nuclei after staining with the fluorescent DNA intercalating dye Hoechst 33342. The treated HepaRG cells were washed with PBS (50 mM pH 7) and fixed with ethanol/acetic acid during 20 min at 4°C. The cells were counterstained for 10 min with Hoechst 33342 dye diluted by 1/1000 (5µg/mL) in PBS and images were taken with an upright microscope (AxioImager M1, Zeiss, France). The image analysis and cell nuclei counting were performed with the simple PCI software (C Imaging® Image Analysis System, Compix, USA). The data were the mean of three independent measurements. The number of cell nuclei was reported as a percentage of the control value. Parameters of the dose-response curves were deduced from a 4-parameter curve fit according to Rodbar [Citation47].
in which y is the percentage of cell nuclei number with respect to the control, ACmin and ACmax are the y values observed for minimal and maximal chelator concentrations respectively, C is the chelator concentration (Cip at the inflection point) and Pip is the slope at inflection point of the sigmoid curve. Due to their biphasic feature, the dose-response curves were fitted as the sum of two sigmoids (double 4-parameter fit). The percentages of the HepaRG cells involved in each viability response were deduced from the ACmin, ACmax and half maximal inhibitory concentration (IC50) values of each sigmoid, which were obtained from these fits.
Results and discussion
Chemistry
The synthesis of the new calix[4]arenes 1-8 have been accomplished in one or two step(s) starting from calix[4]arene ( and ).
Scheme 1. Synthesis of calix[4]arenes 1-5. Reagents: (i) wet K2CO3, N-3-Bromopropyl-N,N-bis(methoxycarbonylmethyl)amine 9, CH3CN, KI, reflux; (ii) anhydrous THF, LiAlH4, 0°C then 90°C; (iii) anhydrous K2CO3, N-3-Bromopropyl-N,N-bis(methoxycarbonylmethyl)amine 9, CH3CN, KI, reflux; (iv) 1) aqueous solution of NaOH 4M, MeOH; 2) HCl, H2O.
![Scheme 1. Synthesis of calix[4]arenes 1-5. Reagents: (i) wet K2CO3, N-3-Bromopropyl-N,N-bis(methoxycarbonylmethyl)amine 9, CH3CN, KI, reflux; (ii) anhydrous THF, LiAlH4, 0°C then 90°C; (iii) anhydrous K2CO3, N-3-Bromopropyl-N,N-bis(methoxycarbonylmethyl)amine 9, CH3CN, KI, reflux; (iv) 1) aqueous solution of NaOH 4M, MeOH; 2) HCl, H2O.](/cms/asset/6f71cfb1-e829-4cb3-8388-cce88bbaf32d/ienz_a_489898_f0003_b.gif)
Scheme 2. Synthesis of N-3-Bromopropyl-N,N-bis(methoxycarbonylmethyl)amine 9. Reagents: (i) BrCH2COOMe, DIEA, MeCN, 0°C.
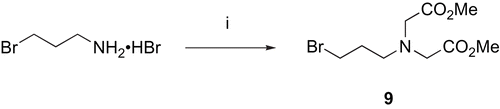
Scheme 3. Synthesis of calix[4]arenes 6–8. Reagents: (i) NaH, Br(CH2)5OC6H4CN, DMF; (ii) 1) LiN(TMS)2, THF; 2) HCl, EtOH; (iii) NH2OH.HCl, t-Bu-OK, DMSO.
![Scheme 3. Synthesis of calix[4]arenes 6–8. Reagents: (i) NaH, Br(CH2)5OC6H4CN, DMF; (ii) 1) LiN(TMS)2, THF; 2) HCl, EtOH; (iii) NH2OH.HCl, t-Bu-OK, DMSO.](/cms/asset/5fb5fdc3-9c0c-485f-800f-fac9e4ac88ff/ienz_a_489898_f0005_b.gif)
The commercially available 3-bromopropylamine hydrobromide can be considered as a useful precursor of bi-functional compounds when reacted with various α-haloesters [Citation48,Citation49] since the ester moiety may be easily derivatised later on. Therefore, the reaction of 3-bromopropylamine.HBr with methyl bromoacetate in cold acetonitrile in the presence of DIEA in excess, gave dimethyl 2,2'-[(3-bromopropyl)imino]diacetate 9 ().
Calix[4]arene was prepared from tert-butyl-calix[4]arene according to already known procedures [Citation50]. Its reaction in refluxing acetonitrile with wet K2CO3 as a base, gave a mixture of the mono 1 and the diametrically substituted cone 2 which could be easily isolated by column chromatography (17% and 12% yield, respectively). On the other hand, using anhydrous potassium carbonate only gave the 25,27-bis functionalised calix[4]arene 2 in a 75% yield without the presence of the mono substituted calixarene 1. The calixarene diol derivative 3 was synthesised from the corresponding ester 1 by reduction with lithium aluminum hydride in freshly distilled THF at 0°C, then refluxed overnight to give a 70% yield. In an analogous manner to that described above, the bis functionalised analogue 4 was obtained from the ester derivative 2, although in a very low (14%) yield. Surprisingly, the mono diol derivative 3 was isolated as the major product, meaning that an unexpected chain cleavage had occurred in the course of the reduction process. The saponification of 2 with an aqueous 4M sodium hydroxide solution in methanol under reflux gave, after acidification, the 25,27-bis-[3-[bis(hydroxycarbonylmethyl)amino]propoxy] calix[4]arene 5 in a 83% yield (). These reactions selectively led to calix[4]arene derivatives in the cone conformation, as substantiated by the characteristic chemical shift (δ = 4.09–4.38 and 3.39–3.47 ppm) and the coupling constants (J = 12.9–13.8 Hz) of the two types of diastereotopic proton signals of the methylene bridges.
The cone conformation of the monoalkylated 3 was confirmed by the 1H NMR spectrum. A typical pattern represented by one 4H doublet at 3.41 ppm for the equatorial protons, and two 2H doublets at 4.18 and 4.26 ppm for the axial protons of the bridging methylene (ArCH2Ar) were observed. Indeed, the monoalkylated 1 exhibited a similar pattern whereas its cone conformation was unambiguously proven by X-ray crystallography. Contrary to the NMR spectrum of compound 1, the OH phenolic groups were indifferenciated and appeared in a large singlet centered to 3.87 ppm along with the two aliphatic alcohols. The spectrum of the monoalkylated 1 showed two sharp signals for the OH groups at 9.44 (2H) and 9.72 ppm (1H). This indicated that two H bonds were weaker than the other one and were probably formed by the two opposite phenolic OH groups with the neighbouring alkoxy oxygen atom, which bore less negative charge than the hydroxyl oxygen atom. [Citation51] Finally, 1 and 3 exhibited a similar pattern for the aromatic protons with 3 H para at 6.6 ppm, 1 H para for the substituted phenyl ring at 6.77 ppm and the remaining 8 H meta in the range 6.92–7.02 ppm for 3, and 6.74, 6.92 and 7.04–7.14 respectively for compound 1.
The reaction of the calix[4]arene with 6 equiv. 4-[(5-bromopentyl)oxy]benzonitrile [Citation52] in dry DMF and in the presence of NaH as a base gave the desired tetra alkylated calix[4]arene 6 () [Citation53–55]. The use of NaH in DMF ensures the formation of the calix[4]arene in the cone conformation. The 1H NMR spectrum of 6 showing the characteristic two doublets at 3.21 and 4.45 ppm (J = 13.35 Hz) for the bridging ArCH2Ar protons indicates a cone structure for compound 6. The tetra-nitrile calix[4]arene 6 was then converted to the tetra-amidine calix[4]arene 7 by the action of lithium trimethylsilylamide (LiN(TMS)2) in THF following by acid hydrolysis [Citation56,Citation57]. The reaction of hydroxylamine in DMSO solution on the tetra nitrile 6 resulted in the formation of the desired tetra-amidoxime calix[4]arene 8 [Citation58].
The 3D structures of the calix[4]arenes 1, 2 and 3 were established by X-ray crystallography analysis [Citation59] and confirmed the cone conformation in the solid state () as anticipated on the basis of 1H NMR data. The molecules adopt a “pinched-cone” conformation commonly found in calix[4]arenes in the cone conformation. With respect to the reference methylene plane C8, C16, C38, C46, the interplanar angles of C1-C6, C9-C14, C17-C22 and C39-C44 rings in 1 are 47.94(9)°, 68.61(9)°, 58.69(8)° and 57.57(9)°, respectively. In 2, the same angles are noticed at 67.17(7)°, 48.41(9)°, 68.6(8)° and 53.56(5)°, respectively. In compound 3, the interplanar angles between C8, C26, C34, C42 and the C1-C6, C9-C14, C27-C32 and C35-C40 rings are noticed at 45.04(9)°, 75.19(8)°, 44.86(9)° and 55.02(9)°, respectively. The angles between the aromatic units of the calix[4]arene skeleton through the methylene carbons are 111.3(2)°, 114.5(2)°, 110.6(2)° and 111.4(2)° for 1, 112.5(2)°, 110(2)°, 113.6(2)° and 110.8(2)° for 2, and 115.9(3)°, 110(3)°, 111.6(3)° and 114.8(3)° for 3, respectively. Not surprisingly, in the three cone calix[4]arenes, the [(2-methylethanoate)amino]propoxy or [(2-hydroxyethyl)amino]propoxy chains adopt various conformations. Moreover, they do not present a regular conformation.
Pharmacology
Comparison of chelator efficiency in the aqueous phase
An efficient iron chelator such as ICL670, is able to remove and to interact with iron complexed to calcein (totally quenched fluorescence) and consequently, to release fluorescent free calcein. The ICL670 concentration inducing 50% of the maximal dequenching of calcein (ED50, ) is close to 0.23µM. The chelating efficiency of ICL670, deduced from this calcein assay, can be compared to that of other iron chelators such as O-Trensox (ED50 = 0.6µM) or desferal (DFO, ED50 = 0.2µM). The various calix[4]arene (1, 3, 4 and 7) were inefficient to restore the calcein fluorescence in this range of concentration (ED50>30µM), while in contrast the compound 5 (ED50 = 0.46µM) exhibited a higher chelating efficiency.
Table 1. 50% of the maximal dequenching of calcein (ED50).Comparison of iron(III) chelating efficiency by using calcein fluorescence measurements in a cell-free system. Fluorescence of 100 nM calcein (λExc = 485nm, λEm = 520nm) in Hepes buffer (20 mM HEPES, 150 mM NaCl, pH 7.3) was detected in a microplate fluorescence reader (free calcein). Iron(III) (1 µM) totally quenched the calcein fluorescence and addition of compounds including ICL670 used as a chelator reference led to a fluorescence recovery depending on the chelator concentration, the kinetic and stoichiometry of their iron binding affinity.
Biological effects in the human hepatocarcinoma HepaRG cell cultures
In the proliferating HepaRG cells, a dose-dependent decrease of cell viability, measured by counting cell nuclei number after Hoechst staining, was observed after a 72 h cell treatment in the presence of increasing concentrations of all compounds (, 0 up to 400 µM). The dose-response curves were biphasic for ICL670 and compound 7 and to a lesser extent for compounds 3 and 5. These curves were fitted as a double four-parameters fit to calculate the percentage of cells involved in each viability component as well as their corresponding IC50 values (). The low concentration components involved 65% of the HepaRG cells treated with ICL670 (IC50 = 4µM), 20% with compound 3 (IC50 = 6µM), 30% with compound 5 (IC50 = 30µM) and 70% with compound 7 (IC50 = 27µM). As deduced from the low LDH leakage in the supernatants ( and ), the chelator treatments in this range of concentration (<20µM) were not cytotoxic for ICL670 and compound 5. In contrast, the decrease in cell viability induced by compound 4 at low concentration (IC50 = 8µM) corresponded to a cytotoxic effect, associated with membrane damage and LDH leakage. The decrease in cell viability observed at the low chelator concentration of compounds 3 and ICL670 (<20µM), was totally reversed by addition of 20µM exogenous Fe(III). The cytostatic effect induced at the low concentrations of these two compounds on proliferating HepaRG cells was not associated with membrane damage. The decrease in cell viability for chelator concentrations higher than 100µM () was associated with LDH leakage in the cell supernatants () and was probably due to the cytotoxic effects of both the chelators and DMSO. In this range of concentrations, the addition of 20µM exogenous Fe(III) was inneffective to reverse the cytotoxic effect of the various compounds.
Table 2. Biological effect of a 72 h treatment of HepaRG cells with various calix[4]arene compounds. Cell viability was evaluated by cell nuclei counting after Hoechst stain. Parameters of the biphasic dose-response curves, including the IC50 values, were deduced from a four parameters fit (see methods). LDH leakage in cell supernatant for compound concentration 100µM was used as an index of membrane damages (cytotoxicity). Data expressed as a percent of the control (absence of compound) are the mean of three independent experiments.
Figure 4. Effect of chelators on cell viability (A, cell nuclei counting after Hoechst stain) and cytotoxicity (B, LDH release in supernatant) in proliferating HepaRG cell cultures. Four days after seeding HepaRG cells were maintained in culture for 72 hours with various concentrations of compounds 1 (__♦__ ), 3 (__▪__), 4 (__▴__), 5 (__•__ ), 7 (__▵__) and ICL670 (−.-◊−.-)
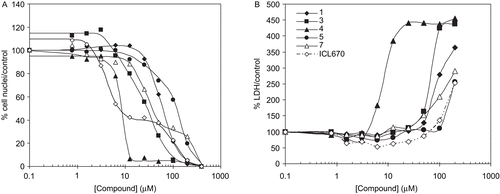
Conclusion
The low solubility of the methyl calix[4]arene ester 2, tetra nitrile calix[4]arene 6 and tetra amidoxime calix[4]arene 8 in aqueous solvents and more particulary in cell culture medium, prevented us from studying their biological efficiency.
With the exception of compound 5 (ED50 = 0.46µM), the Fe(III)-chelating efficiency of these new calix[4]arene derivatives, deduced from the calcein fluorescence recovery in solution, remained low (ED50>30µM) compared to that of ICL670 (ED50 = 0.23µM). On the basis of their inability to remove and interact with iron complexed to calcein, we deduced that the compounds were not efficient iron chelators. The calix[4]arene derivatives were shown to reduce viability of the proliferating HepaRG cells. Low concentrations of compound 4 ((IC50 = 8µM) induced a cytotoxicity leading to membrane damage and LDH leakage in the cell supernatants. As deduced from the absence of an effect of exogenous iron, the effect of calix[4]arene on cell viability was not correlated to iron depletion, with the exception of the cytostatic contribution of compound 3 and ICL670, which were observed at low concentration (<20µM). This effect, dependent on iron depletion, remains to be further explored in order to understand the cytostatic effect of compound 3. Finally, the new substituted calix[4]arenes could open the way to new valuable medicinal chemistry scaffolding.
Acknowledgements
The authors would like to thank Novartis Pharma Laboratories (Basel, Switzerland) for supplying ICL670A.
Declaration of interest
The authors have no competing interests as defined by Informa Healthcare journals Publishing Group, or other interests that might be perceived to influence the results and/or discussion reported in this paper.
References
- Brittenham, GM. Disorders of iron metabolism: iron deficiency and overload. In: Hoffman R, Benz EJ, Shattil SJ, Furie B, Cohen HJ, et al., eds. Hematology: Basic Principles and Practise, 3rd ed New York; Churchill Livingstone, 2000:397–428.
- McCance RA, Widdowson EM. Absorption and excretion of iron. Lancet 1937;ii:680–684.
- Deugnier Y, Charalambous P, Le Quilleuc D, Turlin B, Searle J, Brissot P, Powell LW, Halliday JW. Preneoplastic significance of hepatic iron-free foci in genetic hemochromatosis: a study of 185 patients. Hepatology 1993;18:1363–1369.
- Hsing AW, McLaughlin JK, Olsen JH, Mellemkjar L, Wacholder S, Fraumeni JF. Cancer risk following primary hemochromatosis: a population-based cohort study in Denmark. Int J Cancer 1995;60:160–162.
- Andrews NC. Disorders of iron metabolism. N Engl J Med 1999;341:1986–1995.
- Kalinowski DS, Richardson DR, The evolution of iron chelators for the treatment of iron overload disease and cancer. Pharmacol Rev 2005;57:547–583.
- Soyano A, Chinea M, Romano EL. The effect of desferrioxamine on the proliferative response of rat lymphocytes stimulated with various mitogens in vitro. Immunopharmacol 1984;8:163–169.
- Polson RJ, Jenkins R, Lombard M, Williams AC, Roberts S, Nouri-Aria K, Williams R, Bomford A. Mechanisms of inhibition of mononuclear cell activation by the iron-chelating agent desferrioxamine. Immunology 1990;71:176–181.
- Richardson DR, Milnes K. The potential of iron chelators of the pyridoxal isonicotinoyl hydrazone class as effective antiproliferative agents II: the mechanism of action of ligands derived from salicylaldehyde benzoyl hydrazone and 2-hydroxy-1-naphthylaldehyde benzoyl hydrazone. Blood 1997;89:3025–3038.
- Chenoufi N, Baffet G, Drénou B, Cariou S, Desille M, Clément B, Brissot P, Lescoat G, Loréal O. Deferoxamine arrests in vitro the proliferation of porcine hepatocyte in G1 phase of the cell cycle. Liver 1997;18:60–67.
- Rakba N, Loyer P, Gilot D, Delcros JG, Glaise D, Baret P, Pierre JL, Brissot P, Lescoat G. Antiproliferative and apoptotic effects of O-Trensox, a new synthetic iron chelator, on differentiated human hepatoma cell lines. Carcinogenesis 2000;21:943–51.
- Richardson DR. Potential of iron chelators as effective antiproliferative agents. Can J Physiol Pharmacol 1997;75:1164–1180.
- Hann HWL, Stahlhut MW, Blumberg BS. Iron mutation and tumor growth: decreased tumor growth in iron deficient mice. Cancer Res 1988;48:4168–4170.
- Le NTV, Richardson DR, The role of iron in cell cycle progression and the proliferation of neoplastic cells. Biochim Biophys Acta 2002;1603:31–46.
- Chaston TB, Richardson DR. Iron chelators for the treatment of iron overload disease: relationship between structure, redox activity and toxicity. Am J of Hematology 2003;73:200–210.
- Richardson DR. Iron chelators as therapeutic agents for the treatment of cancer. Crit Rev Oncol/Hematol 2002;42:267–281.
- Richardson DR, Sharpe PC, Lovejoy DB, Senaratne D, Kalinowski DS, Islam M, Bernhardt PV. Dipyridyl thiosemicarbazone chelators with potent and selective antitumor activity form iron complexes with redox activity. J Med Chem 2006;49:6510–6521.
- Gao J, Richardson DR. The potential of iron chelators of the pyridoxal isonicotinoyl hydrazone class as effective antiproliferative agents: IV. The mechanisms involved in inhibiting cell-cycle progression. Blood 2001;98:842–850.
- Finch RA, Liu M, Grill SP, Rose WC, Loomis R, Vasquez KM, Cheng Y, Sartorelli AC. Triapine (3-aminopyridine-2-carboxaldehyde-thiosemicarbazone):a potent inhibitor of ribonucleotide reductase activity with broad spectrum antitumor activity. Biochem Pharmacol 2000;59:938–91.
- Brodie C, Siriwardana G, Lucas J, Schleicher R, Terada N, Szepesi A, Gelfand E, Seligman P. Neuroblastoma sensitivity to growth inhibition by desferrioxamine: evidence for a block in G1 phase of the cell cycle. Cancer Res 1993;53:3968–3975.
- Glickstein H, Breuer W, Loyevsky M, Konijn AM, Shanzer A,Cabantchik ZI. Differential cytotoxicity of iron chelators on malaria-infected cells versus mammalian cells. Blood 1996;87:4871–4878.
- a-Valle P, Timeus F, Piglione M, Rosso P, di Montezemolo LC, Crescenzio N, Marranca D, Ramenghi U. Effect of different exposures to desferrioxamine on neuroblastoma cell lines. Pediatr Hematol Oncol 1995;12:439–446.
- Cappellini MD, Cohen A, Piga A, Bejaoui M, Perrotta S, Agaoglu L, Aydinok Y, Kattamis A, Kilinc Y, Porter J, et al. A phase 3 study of deferasirox (ICL670), A once-daily oral iron chelator, in patients with beta-thalassemia. Blood 2006;107:3455–3462.
- Selig RA, White L, Gramacho C, Sterlinglevis K, Fraser IW, Naidoo D. Failure of iron chelators to reduce tumor growth in human neuroblastoma xenografts. Cancer Res 1998;58:473–478.
- Olivieri NF, Koren G, Matsui D, Liu PP, Blendis L, Cameron R, McClelland RA, Templeton DM. Reduction of tissue iron stores and normalization of serum ferritin during treatment with the oral iron chelator L1 in thalassemia intermedia. Blood 1992;79:2741–2748.
- Al-Refaie FN, Wonke B, Hoffbrand AV, Wickens DG, Nortey P, Kontoghiorges GJ. Efficacy and possible adverse effects of the oral iron chelator 1,2-dimethyl-3-hydroxypyrid-4-one (L1) in thalassemia major. Blood 1992;80:593–599.
- Collins AF, Fassos FF, Stobie S, Lewis N, Shaw D, Fry M, Templeton DM, McClelland RA, Koren G, Olivieri NF. Iron-balance and dose-response studies of the oral iron chelator 1,2-dimethyl-3-hydroxypyrid-4-one (L1) in iron-loaded patients with sickle cell disease. Blood 1994;83:2329–2333.
- Porter JB, Davis BA. Monitoring chelation therapy to achieve optimal outcome in the treatment of thalassaemia. Best Pract Res Clin Haematol 2002;15:329–368.
- Lovejoy D, Richardson DR. Novel “,hybrid”iron chelators derived from aroylhydrazones and thiosemicarbazones demonstrate high anti-proliferative activity that is selective for tumor cells. Blood 2002;100:666–676.
- Bergeron RJ, Wiegand J, McManis JS, Bharti N. The design, synthesis and evaluation of organ-specific iron chelators. J Med Chem 2006;49:7032–7073.
- Liu ZD, Hider RC. Design of iron chelators with therapeutic application. Coord Chem Rev 2002; 232:151–171.
- Liu ZD, Hider RC. Design of clinically useful iron-selective chelators. Medicinal Res Rev 2002;22:26–64.
- Sansone F, Segura M, Ungaro R. Calixarenes in Bioorganic and Biomimetic Chemistry. In: Asfari Z, Böhmer V, Harrowfield J, Vicens J, eds. Calixarenes 2001. Dordrecht, The Netherlands: Kluwer Academic Publishers, 2001:496–512.
- Mutihac L. Functionalized calix[n]arenes as membrane transporters for biological compounds. A minireview. Curr Drug Discov Technol 2008;5:98–104.
- Jose P, Menon S. Lower-rim substituted calixarenes and their applications. Bioinorg Chem Appl 2007:65815.
- Gutsche CD. Calixarenes: monographs in supramolecular chemistry, Royal society of Chemistry. London, 1989.
- Asfari Z, Böhmer V, Harrowfield J, Vicens J, Calixarene. Dordrecht: Kluwer Academic, 2001.
- Guillon J, Léger JM, Sonnet P, Jarry C, Robba M. Synthesis of cone, partial-cone and 1,3-alternate 25,27-bis[1-(2-ethyl)hexyl]- and 25,27-bis[1-(2-tert-butoxy)ethyl]calix[4]arene-crown-6 conformers as potential selective cesium extractants. J Org Chem 2000;65:8283–8289.
- Guillon J, Sonnet P, Malval JP, Massip S, Gosse I, Léger JM, Lapouyade R, Rochette J, Monti JP, Jarry C. Synthesis and cesium binding affinity of new 25,27-bis(alkyloxy)calix[4]arene-crown-6 conformers in relation to the alkyl pendent moiety. Supramol Chem 2002;14:437–450.
- Guillon J, Léger JM, Dapremont C, Denis LA, Sonnet P, Massip S, Jarry C. First synthesis of 1,3-alternate 25,27-dialkyloxy-5,17-diarylcalix[4]arenes-crown-6 as new cesium selective extracants by Suzuki cross-coupling reaction. Supramol Chem 2004;16:319–329.
- Pires VS, Gaboriau F, Guillon J, Nascimento S, Dassonville A, Lescoat G, Desplat V, Rochette J, Jarry C, Sonnet P. Modulation of cell proliferation in rat liver cell cultures by new calix[4]arenes. J Enzyme Inhib Med Chem 2006;21:261–70.
- Rouge P, Silva Pires V, Gaboriau F, Dassonville-Klimpt A, Guillon J, Da Nascimento S, Léger JM, Lescoat G, Sonnet P. Antiproliferative effect on HepaRG cell cultures of new calix[4]arenes. J Enzyme Inhib Med Chem 2009;25:216–227.
- Gripon P, Rumin S, Urban S, Le Seyec J, Glaise D, Cannie I, Guyomard C, Lucas J, Trepo C and Guguen-Guillouzo C. Infection of a human hepatoma cell line by hepatitis B virus. Proc Natl Acad Sci USA 2002;99:15655–15660.
- Troadec MB, Glaise D, Lamirault G, Le Cunff M, Guerin E, Le Meur N, Detivaud L, Zindy P, Leroyer P, Guisle I, Duval H, Gripon P, Theret N, Boudjema K, Guguen-Guillouzo C, Brissot P, Leger JJ and Loreal O. Hepatocyte iron loading capacity is associated with differentiation and repression of motility in the HepaRG cell line. Genomics 2006;87:93–103.
- Rouan MC, Marfil F, Mangoni P, Sechaud R, Humbert H and Maurer G. Determination of a new oral iron chelator, ICL670, and its iron complex in plasma by high-performance liquid chromatography and ultraviolet detection. J Chromatogr B Biomed Sci Appl 2001;755:203–213.
- Thomas F, Serratrice G, Beguin C, Aman ES, Pierre JL, Fontecave M, Laulhere JP. Calcein as a fluorescent probe for ferric iron. Application to iron nutrition in plant cells. J Biol Chem 1999;274:13375–13383.
- Hasinoff BB. The intracellular iron sensor calcein is catalytically oxidatively degraded by iron(II) in a hydrogen peroxide-dependent reaction. J Inorg Biochem 2003;95:157–164.
- Rodbard D, McClean SW. Automated computer analysis for enzyme multiplied immunological techniques. Clin Chem 1977;23:112–115.
- Bonache MC, Chamorro C, Velasquez S, De Clercq E, Balzarini J, Rodriguez Barrios F, Gago F, Camarasa MJ, San-Felix A. Improving the antiviral effocoency and selectivity of HIV-1 reverse transcriptase inhibithor TSAO-T by the introduction of functional groups at the N-3 position. J Med Chem 2005;48:6653–6660.
- Mallia MB, Subramanian S, Mathur A, Sarma HD, Venkatesh M, Banerjee S. On the isolation and evaluation of a novel unsubstituted 5-nitroimidazole derivative as an agent to target tumor hypoxia. Bioorg Med Chem Lett 2008;18:5233–5237.
- Gutsche CD, Lee-Gin L. Calixarenes 12. The synthesis of functionalized calixarenes. Tetrahedron 1986;42:1633–1640.
- Casnati A, Arduini A, Ghidini E, Pochini A, Ungaro R. A general synthesis of calix[4]arene monoalkyl ethers. Tetrahedron 1991;47:2221–2228.
- Berg SS, Newbery G. Chemotherapeutic amidines. X. Substituted 4,4'-diamidino-ω,ω-diphenoxyalkanes and –diphenylethers. J Chem Soc 1949;642–648.
- Barboso S, Garcia Carrera A, Matthews SE, Arnaud-Neu F, Böhmer V, Dozol JF, Rouquette H, Schwing-Weill MJ. Calix[4]arenes with CMPO functions at the narrow rim. Synthesis and extraction properties. J Chem Soc, Perkin Trans 2 1999;4:719–723.
- Rudzevich Y, Rudzevich V, Moon C, Schnell I, Fischer K, Böhmer V. Self-assembly of programmed building blocks into structurally uniform dendrimers. J Am Chem Soc 2005;127:14168–14169.
- Clark TE, Makha M, Raston CL, Sobolev AN. Supersized bilayers based on an O-alkyl substituted calix[4]arene. CrystEngComm 2006;8:707–711.
- Ismail MA, Arafa RK, Brun R, Wenzler T, Miao Y, Wilson WD, Generaux C, Bridges A, Hall JE, Boykin DW. Synthesis, DNA affinity, and antiprotozoal activity of linear dications: terphenyl diamidines and analogues. J Med Chem 2006;49:5324–5332.
- Dalai S, Belov VN, Nizamov S, Rauch K, Finsinger D, de Meijere A. Access to Variously Substituted 5,6,7,8-Tetrahydro-3H-quinazolin-4-ones via Diels-Alder Adducts of Phenyl Vinyl Sulfone to Cyclobutene-Annelated Pyrimidinones. Eur J Org Chem 2006;12:2753–2765.
- Ismail MA, Arafa RK, Wenzler T, Brun R, Tanious FA, Wilson WD, Boykin DW. Synthesis and antiprotozoal activity of novel bis-benzamidinoimidazo[1,2-a]pyridines and 5,6,7,8-tetrahydroimidazo[1,2-a]pyridines. Bioorg Med Chem 2008;16:683–691.
- Supplementary X-ray crystallographic data of compounds 1, 2 and 3 (CCDC-748460, CCDC-748458 and CCDC-748459): Cambridge Crystallographic Data Centre, University Chemical Lab, 12 Union Road, Cambridge, CB2 1EZ, UK; E-mail: [email protected].