Abstract
A series of tetracyclic thienopyrimidines (7–14) was prepared and investigated as inhibitors of acetylcholinesterase from Electrophorus electricus acetylcholinesterase (EeAChE), as well as human acetylcholinesterase (hAChE) and human butyrylcholinesterase (hBChE). A new synthetic procedure was employed for the synthesis of the angularly fused heterocycles 7–10. Among them, the presence of a tetrahydropyrido ring with a benzyl rest at the basic nitrogen was required for EeAChE inhibition. A detailed kinetic analysis of the hyperbolic mixed-type inhibition of EeAChE by 9–14 was performed. These heterocyclic compounds inhibited EeAChE with Ki values of less than 3 µM. Most α values were relatively close to 1, indicating a similar affinity of the inhibitor to the free enzyme and the enzyme-substrate complex. Inhibitor 10 displayed a rather uncompetitive pattern of inhibition (α = 0.47) and a relatively high residual activity of a postulated ternary enzyme-substrate-inhibitor complex (β = 0.24).
Introduction
The serine hydrolase acetylcholinesterase (AChE), a member of the α/β hydrolase family hydrolyzes a broad range of ester and amide substrates, including the neurotransmitter acetylcholine (ACh)Citation1,Citation2. Substrate cleavage proceeds through an acyl transfer mechanism and is mediated by the catalytic triad Ser200-His440-Glu327 (Torpedo californica AChE (TcAChE), numbering)Citation3 located within the active-site at the bottom of a 20 Å deep gorge. Substrate binding is facilitated by the anionic site were aromatic residues, such as Trp84 and Phe330, interact via cation-π interactions and the acidic side chain of Glu199 via electrostatic interaction with the quaternary ammonium group of ACh or acetylthiocholine (ATCh)Citation3,Citation4. A second substrate binding site, the peripheral anionic site (PAS), consists of five residues, Tyr70, Asp72, Tyr121, Trp279, and Tyr334, clustered around the entrance of the active-site gorgeCitation3–5. In addition to the principal physiological function of AChE, that is, hydrolysis of ACh at cholinergic synapses and neuromuscular junctions, several ‘non-classical’ activities of AChE are associated with the PASCitation2,Citation5. For example, AChE has been shown to promote the aggregation and toxicity of β-amyloid, both in vivo and in vitroCitation6.
It was proposed over 25 years ago that degeneration of cholinergic neurons in the basal forebrain and the associated loss of cholinergic neurotransmission in the cerebral cortex contributed significantly to the deterioration in cognitive function seen in patients with Alzheimer’s disease (AD). This cholinergic hypothesis of AD has served as the basis for the majority of treatment strategies and drug development approaches for AD to date. The AChE inhibitors donepezil (), galantamine, and rivastigmine are frequently used drugs to treat the symptoms of ADCitation7–11. Various carbamates have been characterized as pseudo-substrate inhibitors of AChECitation12. During the last decade, several hybrid molecules derived from tacrine () and heterobivalent tacrine derivatives, simultaneously targeting both the active and the PAS, have been developed as inhibitors for both AChE and its relative butyrylcholinesterase (BChE)Citation9–11,Citation13–15. For example, we have designed linker-connected dimers of tacrine with gallamine ()Citation16 or with a coumarin fluorophorCitation17. The latter heterodimer was shown to act not only as an AChE inhibitor in sections from perfused wild-type and triple-transgenic mice, but also as a useful fluorescent probe for β-amyloid in tissues from triple-transgenic mice in vitroCitation17 and in vivoCitation18.
In a previous study, we described 7-benzyl-5,6,7,8-tetrahydro-2-isopropylamino-4H-pyrido[4′,3′:4,5]thieno[2,3-d][1,3]thiazin-4-one I () which inhibits AChE in the submicromolar rangeCitation19. Kinetic analysis as well as structural similarities between donepezilCitation20, AP2238 ()Citation21, and I suggested that these substances act as dual site inhibitors of AChE and bind along the active-site gorge. A detailed kinetic study with compound I and the prototype inhibitors tacrine and gallamine was performed, and I was characterized as a hyperbolic mixed-type inhibitor of AChE from Electrophorus electricusCitation22. A new series of heterocyclic compounds was designed on the basis of structure I as follows. Replacement of the thiazine ring sulfur by nitrogen allowed the fusion of a further ring to the resulting template, i.e. to the 7-benzyl-5,6,7,8-tetrahydro-pyrido[4′,3′:4,5]thieno[2,3-d]pyrimidin-4(1H)-one scaffold. This modification was realized in the tetracyclic representatives 9 and 10 (for structures, see ). The same heterocyclic rings, i.e. imidazole and pyrimidine, were incorporated in the angularly fused structures 13 and 14. Replacement of the benzyl-substituted nitrogen by a methylene unit resulted in the tetrahydrobenzo analogs 7, 8, and 11, 12 respectively. These eight compounds were included in a kinetic study with AChE from Electrophorus electricus AChE (EeAChE). Their inhibitory activities toward human AChE (hAChE) and human BChE (hBChE) are also reported herein.
Table 1 Inhibition of acetylcholinesterase from Electrophorus electricus (EeAChE), human acetylcholinesterase (hAChE) and human butyrylcholinesterase (hBChE) by compounds 7–14.
Materials and methods
General methods and materials
Melting points were obtained on a Rapido Boetius apparatus and are uncorrected. 1H nuclear magnetic resonance (NMR) spectra (500 MHz) and 13C NMR spectra (125 MHz) were recorded on a Bruker Avance instrument. Elemental analyses were performed on a Vario EL apparatus. Thin-layer chromatography was carried out using aluminum sheets coated with silica gel 60 F254 (Merck). Enzymatic activity of cholinesterases was measured at a Varian Cary Bio 50 UV/Vis spectrophotometer. AChE from EeAChE was purchased from Fluka (Deisenhofen, Germany). Human acetylcholinesterase (hAChE), ATCh, butyrylthiocholine (BTCh) and 5,5′-dithio-bis-(2-nitrobenzoic acid) (DTNB) were obtained from Sigma (Steinheim, Germany). hBChE was purchased from Lee Biosolutions (St. Louis, MO). Compounds 5 and 6 were prepared according to reported proceduresCitation23–26. Compounds 11–14 were synthesized according to literature reportsCitation27,Citation28.
Synthesis
2,3,6,7,8,9-Hexahydro-benzothieno[2,3-d]-imidazo[1,2-a]pyrimidin-5(11H)-one (7): general procedure for the preparation of linearly fused compounds 7–10. To a solution of compound 5 (0.98 g, 3 mmol) in 2-methoxyethanol (12 mL), ethylenediamine (11.54 g, 12.8 mL, 192 mmol) was added. The mixture was stirred in a sealed flask at 150°C for 24 h. After it was cooled, the solution was poured onto ice-water (150 mL), and the precipitate was filtered off. The crude material was recrystallized from toluene to give 7 (0.69 g, 93%). An analytic sample was obtained from recrystallization from ethanol: mp 261–267°C, conversion 196–201°C; 1H NMR [dimethyl sulfoxide d6 (DMSO-d6)] δ 1.68–1.78 (m, 4H, 7-H, 8-H), 2.57–2.60, 2.74–2.77 (each m, total 4H, 6-H, 9-H), 3.61, 4.01 (each t, J = 8.7 Hz, total 4H, 2-H, 3-H), 7.72 (s, 1H, 11-H); 13C NMR (DMSO-d6) δ 22.06, 22.86 (C-7, C-8), 24.33, 25.38 (C-6, C-9) 40.06, 42.12 (C-2, C-3), 114.00 (C-5a), 125.15, 130.14 (C-5b, C-9a), 155.88, 157.00 (C-11a, C-10a), 166.02 (C-5). Anal. found: C, 58.74; H, 5.46; N, 16.50; S, 12.52. C12H13N3OS requires: C, 58.28; H, 5.30; N, 16.99; S, 12.97.
2,3,4,7,8,9,10,12-Octahydro-6H-benzothieno[2,3-d]pyrimido[1,2-a]pyrimidin-6-one (8). A mixture of compound 5 (0.98 g, 3 mmol) and 1,3-diaminopropane (2.96 g, 3.4 mL, 40 mmol) was refluxed for 40 h. It was kept at 0°C for 48 h, the precipitate was filtered off and washed with H2O. The crude product was dried and recrystallized from toluene to give 8 (0.46 g, 59%): mp 292–293°C; 1H NMR (DMSO-d6) δ 1.67–1.77 (m, 4H, 8-H, 9-H), 1.88–1.91 (m, 2H, 3-H), 2.55–2.57, 2.73–2.76 (each m, total 4H, 7-H, 10-H), 3.27–3.25, 3.84 (m, t, J = 5.8 Hz, total 4H, 2-H, 4-H), 7.71 (s, 1H, 12-H); 13C NMR (DMSO-d6) δ 19.85 (C-3), 22.11, 22.90 (C-8, C-9), 24.33, 25.42 (C-7, C-10) 38.64, 38.82 (C-2, C-4), 112.23 (C-6a), 123.83, 130.21 (C-6b, C-10a), 150.02, 157.67 (C-11a, C-12a), 165.10 (C-6). Anal. found: C, 60.01; H, 6.10; N. 15.64; S, 11.90. C13H15N3OS requires: C, 59.74; H, 5.79; N, 16.08, S, 12.27.
8-Benzyl-2,3,6,7,8,9-hexahydro-imidazo[1,2-a]pyrido[4′,3′:4,5]thieno[2,3-d]pyrimidin-5(11H)-one (9). Compound 6 (1.26 g, 3 mmol) was reacted with ethylenediamine (11.54 g, 12.8 mL, 192 mmol) in 2-methoxyethanol (12 mL). The crude product was recrystallized from 2-methoxyethanol to give 9 (0.38 g, 38%): mp 222–225°C; 1H NMR (DMSO-d6) δ 2.70 (t, J = 5.7 Hz, 2H, 7-H), 2.82 (t, J = 5.7 Hz, 2H, 6-H), 3.45 (s, 2H, 9-H), 3.62, 4.00 (each t, J = 8.8 Hz, total 4H, 2-H, 3-H), 3.65 (s, 2H, C6H5-CH2), 7.23–7.34 (m, 5H, C6H5), 7.80 (s, 1H, 11-H); 13C NMR (DMSO-d6) δ 25.74 (C-6), 40.08, 42.10 (C-2, C-3), 49.35 (C-7), 51.00 (C-9), 61.11 (C6H5-CH2), 113.60 (C-5a), 122.85, 128.54 (C-5b, C-9a), 127.15 (C-4′), 128.37, 128.89 (C-2′, C-3′), 138.38 (C-1′), 156.02, 156.93 (C-10a, C-11a), 166.50 (C-5). Anal. found: C, 64.20; H, 5.89; N., 16.43. C18H18N4OS requires: C, 63.88; H, 5.36; N, 16.56.
9-Benzyl-2,3,4,7,8,9,10,12-octahydro-6H-pyrido[4′,3′:4,5]thieno[2,3-d]pyrimido[1,2-a]pyrimidin-6-one (10). Compound 6 (1.26 g, 3 mmol) was reacted with 1,3-diaminopropane (14.23 g, 16 mL, 192 mmol) in 2-methoxyethanol (12 mL). The crude product was recrystallized from 2-methoxyethanol to give 10 (125 mg, 11%): mp 202–207°C, conversion 193–197°C; 1H NMR (DMSO-d6) δ 1.88–1.93 (m, 2H, 3-H), 2.70 (t, J = 5.7 Hz, 2H, 8-H), 2.79–2.81 (m, 2H, 7-H), 3.44 (s, 2H, 10-H), 3.65 (s, 2H, C6H5-CH2), 3.84 (t, J = 5.8 Hz, 2H, 2-H or 4-H), 7.30–7.33 (m, 5H, C6H5), 7.76 (s, 1H, 12-H), one signal (2H, 2-H or 4-H) is not visible due to the water signal (3.20–3.40); 1H NMR (CDCl3) δ 2.02–2.07 (m, 2H, 3-H), 2.83 (t, J = 5.8 Hz, 2H, 8-H), 3.03 (t, J = 5.8 Hz, 2H, 7-H), 3.44–3.48, 4.01 (m, t, J = 5.8 Hz, total 4H, 2-H, 4-H), 3.55 (s, 2H, 10-H), 3.72 (s, 2H, C6H5-CH2), 6.42 (s, 1H, 12-H), 7.27–7.37 (m, 5H, C6H5); 13C NMR (DMSO-d6) δ 19.79 (C-3), 25.80 (C-7), 38.63, 38.84 (C-2, C-4), 49.39, 51.06 (C-8, C-10), 61.13 (C6H5-CH2), 111.76 (C-6a), 121.47, 128.64 (C-6b, C-10a), 127.15 (C-4′), 128.37, 128.90 (C-2′, C-3′), 138.42 (C-1′), 150.91, 157.68 (C-11a, C-12a), 165.77 (C-6). Anal. found: C, 61.99; H, 5.66; N., 15.03. C19H20N4OS × H2O requires: C, 61.60; H, 5.99; N, 15.12.
2,3,8,9,10,11-Hexahydro-benzothieno[3,2-e]imidazo[1,2-c]pyrimidine (11). 1H NMR (DMSO-d6) δ 1.67–1.80 (m, 4H, 9-H, 10-H), 2.65–2.69, 2.74–2.78 (each m, total 4H, 8-H, 11-H), 3.83 (t, J = 9.9 Hz, 2H, 2-H), 4.00 (t, J = 9.9 Hz, 2H, 3-H), 7.90 (s, 1H, 5-H). 13C NMR (DMSO-d6) δ 22.01, 22.70 (C-9, C-10), 24.49, 25.44 (C-8, C-11), 45.27 (C-3), 53.17 (C-2), 117.22 (C-11b), 130.47, 131.36 (C-7a, C-11a), 144.20 (C-5), 150.70, 157.56 (C-6a, C-11c).
3,4,9,10,11,12-Hexahydro-2H-benzothieno[3,2-e]pyrimido[1,2-c]pyrimidine (12). 1H NMR (DMSO-d6) δ 1.67–1.77 (m, 4H, 10-H, 11-H), 1.86–1.92 (m, 2H, 3-H), 2.67–2.72, 2.84–2.88 (each m, total 4H, 9-H, 12-H), 3.43 (t, J = 5.7 Hz, 2H, 2-H), 3.95 (t, J = 5.4 Hz, 2H, 4-H), 7.73 (s, 1H, 6-H); 1H NMR (CDCl3) δ 1.69–1.78 (m, 4H, 10-H, 11-H), 1.89–1.94 (m, 2H, 3-H), 2.64–2.67, 2.87–2.90 (each m, total 4H, 9-H, 12-H), 3.51 (t, J = 5.7 Hz, 2H, 2-H), 3.77 (t, J = 5.8 Hz, 2H, 4-H), 7.16 (s, 1H, 6-H); 13C NMR (DMSO-d6) δ 19.59 (C-3), 22.08, 22.40 (C-10, C-11), 24.87, 26.60 (C-9, C-12), 43.04 (C-2), 46.47 (C-4), 120.05 (C-12b), 130.84, 132.27 (C-8a, C-12a), 144.70, 155.87 (C-7a, C-12c), 146.81 (C-6).
9-Benzyl-2,3,8,9,10,11-hexahydro-imidazo[1,2-c]pyrido[4′,3′:4,5]thieno[3,2-e]pyrimidine (13). 1H NMR (DMSO-d6) δ 2.72 (t, J = 5.8 Hz, 2H, 10-H), 2.82 (t, J = 5.8 Hz, 2H, 11-H), 3.55 (s, 2H, 8-H), 3.67 (s, 2H, C6H5-CH2), 3.85 (t, J = 10.0 Hz, 2H, 2-H), 4.00 (t, J = 10.0 Hz, 2H, 3-H), 7.23–7.35 (m, 5H, C6H5), 7.92 (s, 1H, 5-H); 13C NMR (DMSO-d6) δ 25.67 (C-11), 45.33 (C-3), 49.23 (C-10), 51.06 (C-8), 53.18 (C-2), 60.9 (C6H5-CH2), 116.79 (C-11b), 127.19 (C-4′), 128.39, 128.91 (C-2′, C-3′), 128.85, 129.14 (C-7a, C-11a), 138.29 (C-1′), 144.46 (C-5), 150.57, 158.20 (C-6a, C-11c).
10-Benzyl-3,4,9,10,11,12-hexahydro-2H-pyrido[4′,3′:4,5]thieno[3,2-e]pyrimido[1,2-c]pyrimidin (14). 1H NMR (DMSO-d6) δ 1.80–1.85 (m, 2H, 3-H), 2.68 (t, J = 5.8 Hz, 2H, 11-H), 2.89 (t, J = 5.8 Hz, 2H, 12-H), 3.39 (t, J = 5.5 Hz, 2H, 2-H), 3.52 (s, 2H, 9-H), 3.65 (s, 2H, C6H5-CH2), 3.86 (t, J = 5.7 Hz, 2H, 4-H), 7.13–7.33 (m, 5H, C6H5), 7.55 (s, 1H, 6-H); 13C NMR (DMSO-d6) δ 20.13 (C-3), 27.11 (C-12), 43.79 (C-2), 46.07 (C-4), 49.51 (C-11), 51.30 (C-9), 60.96 (C6H5-CH2), 120.98 (C-12b), 127.14 (C-4′), 128.37, 128.84 (C-2′, C-3′), 128.62, 129.86 (C-8a, C-12a), 138.34 (C-1′), 143.44, 154.77 (C-7a, C-12c), 147.37 (C-6).
Enzyme inhibition experiments
Cholinesterase inhibition was assayed spectrophotometrically at 412 nm at 25°CCitation19,Citation29. Product formation was monitored over 5 min. Assay buffer was 100 mM sodium phosphate, 100 mM NaCl, pH 7.3. Enzyme stock solutions were prepared with assay buffer in the following concentrations ∼ 100 U/mL (EeAChE), ∼ 3 U/mL (hAChE), ∼ 10 U/mL (hBChE), and were kept at 0°C. Appropriate dilutions of the EeAChE (1:30) and hBChE (1:10) solutions were done immediately before starting the measurements. Solutions of ATCh (5, 10, 15, or 20 mM), BTCh (10 mM), and DTNB (7 mM) were prepared in assay buffer and kept at 0°C. Stock solutions of the inhibitors were prepared in acetonitrile. EeAChE was assayed as follows. Into a cuvette containing 830 µL assay buffer, 50 µL of the DTNB solution, 50 µL acetonitrile, 10 µL of an inhibitor solution, and 10 µL of an enzyme solution were added and thoroughly mixed. After incubation for 15 min at 25°C, the reaction was initiated by adding 50 µL of the ATCh solution. The following final concentration were used, ∼ 0.033 U/mL of EeAChE, 250, 500, 750, or 1000 µM of ATCh, 350 µM of DTNB, 6% acetonitrile. Similarly, hAChE (final concentration ∼ 0.03 U/mL) was assayed with 500 µM ATCh, and hBChE (final concentration ∼ 0.01 U/mL) with 500 µM BTCh. The rates of enzyme-catalyzed substrate hydrolysis were corrected by those of the non-enzymatic hydrolysis of ATCh or BTCh, respectively, as determined by using 10 µL of assay buffer, instead of the enzyme solution. A Km value of 550 µMCitation19 for the substrate ATCh used in the EeAChE assay was taken for kinetic analyses.
Results and discussion
Synthesis
The preparation of the linearly fused compounds 7–10 is depicted in Scheme 1. Ethyl 2-aminothiophenecarboxylates 1 and 2 were prepared by Gewald synthesis, for a review, seeCitation30, and reacted with phenylisothiocyanate to afford thiourea derivatives 3 and 4. Cyclocondensation and immediate S-alkylationCitation31 gave tricyclic pyrimidines 5 and 6 bearing a nucleofuge at C-2. Indeed, upon reaction with diaminoalkanes, methylmercaptane was eliminated. The products, however, were formed by a more complex mechanism, which can be envisaged as follows. After the initial nucleophilic attack of the diaminoalkane and repulsion of the leaving group, a Dimroth rearrangement occurred. Then, an intramolecular nucleophilic attack of the terminal amino function, again at C-2, led to the replacement of aniline and formation of the fourth fused ring in the imidazo or pyrimido compounds 7–10. Their structures were confirmed by elemental analyses, 1H and 13C NMR data. The angularly fused compounds 11–14 (structures in ) were prepared according to literature reportsCitation27,Citation28, and NMR data, not published so far, are given in the Material and Methods section. NMR signals were assigned on the basis of and 1H nuclear Overhauser effect and 13C/1H correlation experiments. In order to distinguish the triplets for 2-H and 3-H (in the case of 11 and 13), or 2-H and 4-H (in the case of 12 and 14), respectively, a nuclear overhauser effect (NOE) experiment was exemplary done for compound 12 (). On irradiation of the 6-H resonance, a NOE in 4-H was observed. The corresponding signals in the 13C NMR spectra were assigned with heteronuclear single-quantum coherence spectra.
Inhibition of cholinesterases
The activity of EeAChE was determined in a coupled assay with the substrate ATCh and DTNB. Active inhibitors were measured at five different concentrations. Plots of the rates versus inhibitor concentration, [I], did not become asymptotic to the x-axis. A residual activity at infinite concentration of the inhibitor, v[I]→∞, was considered. The value of v[I]→∞ at a defined substrate concentration was obtained from a plot of the rates versus [I] and non-linear regression according to Equation 1,
where v0 is the velocity in the absence of the inhibitor. The IC50 value calculated by this equation corresponds to the concentration of the inhibitor which reduces the rate of the enzyme-catalyzed reaction to a velocity (v0 – v[I]→∞)/2 + v[I]→∞. Data from EeAChE inhibition experiments at a substrate concentration of 500 µM are given in . A corresponding plot for inhibitor 10 is shown in .
Figure 3. Kinetic model for the interaction of hyperbolic mixed-type inhibitors with Electrophorus electricus acetylcholinesterase. ES, enzyme-substrate complex; ESI, enzyme-substrate-inhibitor complex; EI, enzyme-inhibitor complex.
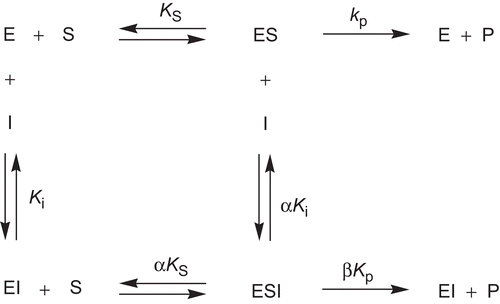
Figure 4. Inhibition of Electrophorus electricus acetylcholinesterase (EeAChE) by compound 10. Plot of the rates versus [I] for the inhibition of EeAChE in 100 mM sodium phosphate, 100 mM NaCl, pH 7.3 with 350 µM 5,5′-dithio-bis-(2-nitrobenzoic acid) (DTNB), 6% acetonitrile and ∼ 0.03 U/mL EeAChE. Rates were obtained by linear regression of the progress curves and are mean values of duplicate experiments. A rate at a substrate concentration of 500 µM in the absence of inhibitor was set 100%. (A) Initial substrate concentration was 250 µM acetylthiocholine (ATCh). The solid line was drawn using the best-fit parameters from a fit according to Equation 1, which gave IC50 = 1.61 ± 0.04 µM and v[I]→∞ = 5.0 ± 0.5%. (B) Initial substrate concentration was 500 µM ATCh. Non-linear regression according to Equation 1 gave IC50 = 1.51 ± 0.07 µM (see ) and v[I]→∞ = 11 ± 1%. (C) Initial substrate concentration was 750 µM ATCh. Non-linear regression according to Equation 1 gave IC50 = 1.39 ± 0.09 µM and v[I]→∞ = 17 ± 2%. (D) Initial substrate concentration was 1000 µM ATCh. Non-linera regression according to Equation 1 gave IC50 = 1.38 ± 0.07 µM and v[I]→∞ = 20 ± 2%.
![Figure 4. Inhibition of Electrophorus electricus acetylcholinesterase (EeAChE) by compound 10. Plot of the rates versus [I] for the inhibition of EeAChE in 100 mM sodium phosphate, 100 mM NaCl, pH 7.3 with 350 µM 5,5′-dithio-bis-(2-nitrobenzoic acid) (DTNB), 6% acetonitrile and ∼ 0.03 U/mL EeAChE. Rates were obtained by linear regression of the progress curves and are mean values of duplicate experiments. A rate at a substrate concentration of 500 µM in the absence of inhibitor was set 100%. (A) Initial substrate concentration was 250 µM acetylthiocholine (ATCh). The solid line was drawn using the best-fit parameters from a fit according to Equation 1, which gave IC50 = 1.61 ± 0.04 µM and v[I]→∞ = 5.0 ± 0.5%. (B) Initial substrate concentration was 500 µM ATCh. Non-linear regression according to Equation 1 gave IC50 = 1.51 ± 0.07 µM (see Table 1) and v[I]→∞ = 11 ± 1%. (C) Initial substrate concentration was 750 µM ATCh. Non-linear regression according to Equation 1 gave IC50 = 1.39 ± 0.09 µM and v[I]→∞ = 17 ± 2%. (D) Initial substrate concentration was 1000 µM ATCh. Non-linera regression according to Equation 1 gave IC50 = 1.38 ± 0.07 µM and v[I]→∞ = 20 ± 2%.](/cms/asset/f7e3d623-891d-4eda-aaee-d8cf80944dce/ienz_a_504674_f0005_b.gif)
In order to discover the type of inhibition of compounds 9–14 towards EeAChE, the assay used to determinate IC50 values was performed at four different substrate concentrations (250 µM, 500 µM, 750 µM, and 1000 µM). A general kinetic model () for the interaction with EeAChE was considered to account for the residual activity observed. This model includes the possible binding of the substrate, S, and the inhibitor, I, to the enzyme, E, at different sites and thus the formation of a ternary enzyme-substrate-inhibitor complex (ESI). The dissociation constants of ESI, are αKS and αKi. The complex ESI is still functional with a decreased rate of product formation governed by the catalytic constant, βkP. This type of inhibition was referred to as hyperbolic mixed-type inhibitionCitation32.
To characterize the EeAChE-inhibitor interaction, the parameters α and β were determined by using the specific velocity plot, introduced by BaiciCitation33. The kinetic analysis is exemplarily shown for inhibitor 10 in and . The specific velocity plot () is described by the specific velocity equation (Equation 2).
Figure 5. Specific velocity plot for the inhibition of Electrophorus electricus acetylcholinesterase (EeAChE) by compound 10. Concentrations of 10 were as follows: open circles, [I] = 1 μM; full circles, [I] = 2 μM; open squares, [I] = 3 μM; full squares, [I] = 4 μM; open triangles, [I] = 5 μM. Data were from duplicate measurements at four different substrate concentrations (250 µM, 500 µM, 750 µM, and 1000 µM). Linear regression according to Equation 2 gave values for vertical intercepts at ([S]/Km)/(1 + [S]/Km) = 0 (a values) and for vertival intercepts at ([S]/Km)/(1 + [S]/Km) = 1 (b values).
![Figure 5. Specific velocity plot for the inhibition of Electrophorus electricus acetylcholinesterase (EeAChE) by compound 10. Concentrations of 10 were as follows: open circles, [I] = 1 μM; full circles, [I] = 2 μM; open squares, [I] = 3 μM; full squares, [I] = 4 μM; open triangles, [I] = 5 μM. Data were from duplicate measurements at four different substrate concentrations (250 µM, 500 µM, 750 µM, and 1000 µM). Linear regression according to Equation 2 gave values for vertical intercepts at ([S]/Km)/(1 + [S]/Km) = 0 (a values) and for vertival intercepts at ([S]/Km)/(1 + [S]/Km) = 1 (b values).](/cms/asset/b61b553d-caba-430a-9df3-413a27041331/ienz_a_504674_f0006_b.gif)
Figure 6. Replots of the specific velocity plot shown in . Values a/(a-1) (full circles) and b(b-1) (open circles) were plotted versus the reciprocal concentrations of inhibitor 10. Linear regression according to Equations 3 and 4 gave values Ki = 2.07 ± 0.19 µM, αKi = 0.98 ± 0.13 µM, β = 0.24.
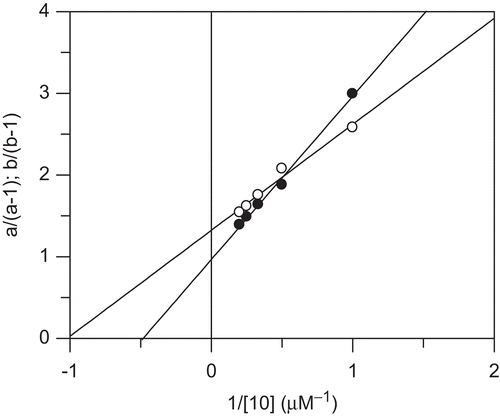
For the replots (), the intersection points for ([S]/Km)/(1 + [S]/Km) = 0 (a values) and for ([S]/Km)/(1 + [S]/Km) = 1 (b values) were used. These replots according to Equations 3 and 4 gave β-values from the vertical intercept (Equation 4), and α-values from β and the vertical intercept (Equation 3). The plot according to Equation 3 gave access to the Ki value by dividing the slope by the intercept. The data for compounds 9–14 are given in .
hAChE was assayed as described above for EeAChE, and IC50 values were obtained using Equation 1 (). The activity of hBChE was measured similarly with BTCh as the substrate. IC50 values were assigned by non-linear regression according to Equation 5.
As noted above, active EeAChE inhibitors of the present series exhibited residual activity at infinite concentration. This behavior is exemplarily shown for 10 in , and the rates v[I]→∞, relative to v0, are listed there. These findings let us conclude that β () takes a value different from zero. To elucidate the interaction between 9 and 14 and EeAChE, it was determined whether the inhibitor binds to the enzyme-substrate complex, ES, with a greater affinity than to the free enzyme, E, or vice versa. The preference of the inhibitor to bind to ES is reflected in values α < 1 () that indicates mixed-type inhibition with a pronounced uncompetitive component. A higher affinity of the inhibitor to E, and thus values α > 1, corresponds to mixed-type inhibition with a more competitive character. Ki values being independent of the substrate concentration also become available that way.
As shown in , the v[I]→∞ values of compound 10 (between 5% and 20%) increased with increasing substrate concentrations (between 250 and 1000 µM). This relationship was also recovered for 9 and 11–14 (data not shown). It reflects that the residual catalytic activity of a postulated ternary ESI complex depended on the concentration of the substrate.
The parameters obtained by the kinetic analyses of the hyperbolic mixed-type inhibition of EeAChE by 9–14 are given in . These heterocyclic compounds inhibited EeAChE with Ki values of less than 3 µM. Most α values were relatively close to 1, indicating a similar affinity of the inhibitor to the free enzyme and the ES complex. Inhibitor 10 displayed a rather uncompetitive pattern of inhibition (α = 0.47). Noteworthy, the highest β value was also obtained for 10, indicating that EeAChE when saturated with 10, would exhibit a relatively high residual activity. From the observed v[I]→∞ values and the derived β values different from zero, the occurrence of a ternary ESI complex could be predicted (). For 9–14, a purely competitive type of inhibition and thus a relevant interaction with the esteratic site of AChE could therefore be excluded.
Structure-activity relationships for the cholinesterase-inhibiting activity of 7–14 could be drawn as follows. Among the linearly fused derivatives 7–10, the presence of a basic N-benzyl moiety was a prerequisite for potency against EeAChE. Inhibitors 9 and 10 resembled donepezil, AP2238, and I () with respect to the benzyl substituent, its attachment to a basic nitrogen and the carbonyl group in a similar distance. The crystal structure of TcAChE complexed with donepezilCitation20 showed the inhibitor oriented along the axis of the gorge, extending from the anionic site to the PAS. Main interaction occurred through aromatic π-π stacking between Trp84 and the benzyl moiety of donepezil, orientated to the bottom of the gorge, and between Trp279 and the indanone ring of donepezil. The charged piperidine nitrogen made a cation-π interaction with Phe330 at the midpoint of the gorge. The carbonyl interacted with the aromatic rings of Phe331 and Phe290, and indirectly via a water molecule with the amide bond of Phe288. Donepezil did not directly interact with residues of the catalytic triad. Similar contacts stabilized the complex of AP2238 with human AChE as concluded from docking studies. The coumarin ring interacted with the PAS and the carbonyl group established an H-bond interaction with a phenylalanine backbone amide groupCitation21. Donepezil, AP2238 and I have been described as mixed-type inhibitors of EeAChE. groupCitation21,Citation22,Citation34. Moreover, 4H-pyrido[4′,3′:4,5]thieno[2,3-d][1,3]oxazin-4-ones, relatives of I, showed a reduced inhibitory potency towards EeAChE when the benzyl rest was shorted, whereas an exchange of N-benzyl by oxygen or a methylene unit led to a loss in activityCitation19. The kinetic analyses, as discussed above, and the structural resemblance to the known inhibitors led us to suspect a similar orientation of our inhibitors 9 and 10 in the active-site gorge of AChE.
Compounds 7 and 8 were inactive against the human enzymes hAChE and hBChE, too, while the AChE-inhibiting activity of 9 and 10 was also observed at hAChE. Compound 9 was completely inactive against hBChE. Compound 10 was the most active representative of this series at hAChE with an IC50 of around 6 µM. It exhibited a 10-fold higher IC50 value at hBChE.
Unexpectedly, the angularly fused heterocycles 11–14 were all found to inhibit EeAChE in the low micromolar range, regardless whether they possess a basic, benzyl-substituted nitrogen or not. Their α values were somewhat higher than 1, indicating a mixed-type inhibition with a slightly pronounced competitive character. These inhibitors, lacking the carbonyl group of the angular derivatives, might be accommodated in a different way along the active-site gorge. However, as they exhibited only weak potency against human AChE and no selectivity for hAChE over hBChE, a more detailed investigation was not attempted.
Conclusions
A series of eight tetracyclic compounds (7–14) was prepared by routes starting from 2-aminothiophene derivatives, accessible by Gewald reaction. The kinetic analysis of the hyperbolic mixed-type inhibition of EeAChE by 9–14 performed in this study was based on the determination of the inhibitory parameters Ki, αKi, and β. A residual enzymatic activity at an infinite inhibitor concentration and thus a catalytically active ternary ESI complex was concluded for the active compounds 9–14. These compounds do not reach the potency and selectivity of other cholinesterase inhibitors, in particular those of some tacrine-derived dimers. However, the linearly fused derivative 10, exhibiting an IC50 value of around 6 µM for human AChE, will serve as a starting point for further structural modifications. For example, an extrusion of the thiophene sulfur would result in a structure of higher flexibility and more closely related to that of donepezil, with an N-benzyl piperidine moiety as substituent at a bicyclic system.
Declaration of interest
This work was supported by the DFG, Graduate College 677.
References
- Soreq H, Seidman S. Acetylcholinesterase–new roles for an old actor. Nat Rev Neurosci 2001; 2:294–302.
- Silman I, Sussman JL. Acetylcholinesterase: ‘classical’ and ‘non-classical’ functions and pharmacology. Curr Opin Pharmacol 2005; 5:293–302.
- Sussman JL, Harel M, Frolow F, Oefner C, Goldman A, Toker L, Silman I. Atomic structure of acetylcholinesterase from Torpedo californica: a prototypic acetylcholine-binding protein. Science 1991; 253:872–879.
- Colletier JP, Fournier D, Greenblatt HM, Stojan J, Sussman JL, Zaccai G, Silman I, Weik M. Structural insights into substrate traffic and inhibition in acetylcholinesterase. EMBO J 2006; 25:2746–2756.
- Johnson G, Moore SW. The peripheral anionic site of acetylcholinesterase: structure, functions and potential role in rational drug design. Curr Pharm Des 2006; 12:217–225.
- Inestrosa NC, Dinamarca MC, Alvarez A. Amyloid-cholinesterase interactions. Implications for Alzheimer’s disease. FEBS J 2008; 275:625–632.
- Giacobini E, Becker RE. One hundred years after the discovery of Alzheimer’s disease. A turning point for therapy? J Alzheimers Dis 2007; 12:37–52.
- Melnikova I. Therapies for Alzheimer’s disease. Nat Rev Drug Discov 2007;6:341–342.
- Muñoz-Torrero D, Camps P. Dimeric and hybrid anti-Alzheimer drug candidates. Curr Med Chem 2006; 13:399–422.
- Musial A, Bajda M, Malawska B. Recent developments in cholinesterases inhibitors for Alzheimer’s disease treatment. Curr Med Chem 2007; 14:2654–2679.
- Muñoz-Torrero D. Acetylcholinesterase inhibitors as disease-modifying therapies for Alzheimer’s disease. Curr Med Chem 2008; 15:2433–2455.
- Lin G, Liu YC, Lin YF, Wu YG. Ortho effects in quantitative structure-activity relationships for acetylcholinesterase inhibition by aryl carbamates. J Enzyme Inhib Med Chem 2004; 19:395–401.
- Marco-Contelles J, León R, de Los Ríos C, Guglietta A, Terencio J, López MG, García AG, Villarroya M. Novel multipotent tacrine-dihydropyridine hybrids with improved acetylcholinesterase inhibitory and neuroprotective activities as potential drugs for the treatment of Alzheimer’s disease. J Med Chem 2006; 49:7607–7610.
- Elsinghorst PW, Tanarro CM, Gütschow M. Novel heterobivalent tacrine derivatives as cholinesterase inhibitors with notable selectivity toward butyrylcholinesterase. J Med Chem 2006; 49:7540–7544.
- Tumiatti V, Minarini A, Bolognesi ML, Milelli A, Rosini M, Melchiorre C. Tacrine derivatives and Alzheimer’s disease. Curr Med Chem 2010;17:1825–1838.
- Elsinghorst PW, Cieslik JS, Mohr K, Tränkle C, Gütschow M. First gallamine-tacrine hybrid: design and characterization at cholinesterases and the M2 muscarinic receptor. J Med Chem 2007;50:5685–5695.
- Elsinghorst PW, Härtig W, Goldhammer S, Grosche J, Gütschow M. A gorge-spanning, high-affinity cholinesterase inhibitor to explore beta-amyloid plaques. Org Biomol Chem 2009;7:3940–3946.
- Härtig W, Kacza J, Paulke BR, Grosche J, Bauer U, Hoffmann A, Elsinghorst PW, Gütschow M. In vivo labelling of hippocampal beta-amyloid in triple-transgenic mice with a fluorescent acetylcholinesterase inhibitor released from nanoparticles. Eur J Neurosci 2010; 31:99–109.
- Pietsch M, Gütschow M. Synthesis of tricyclic 1,3-oxazin-4-ones and kinetic analysis of cholesterol esterase and acetylcholinesterase inhibition. J Med Chem 2005; 48:8270–8288.
- Kryger G, Silman I, Sussman JL. Structure of acetylcholinesterase complexed with E2020 (Aricept): implications for the design of new anti-Alzheimer drugs. Struct Fold Des 1999;7:297–307.
- Piazzi L, Rampa A, Bisi A, Gobbi S, Belluti F, Cavalli A, Bartolini M, Andrisano V, Valenti P, Recanatini M. 3-(4-[[Benzyl(methyl)amino]methyl]phenyl)-6,7-dimethoxy-2H-2-chromenone (AP2238) inhibits both acetylcholinesterase and acetylcholinesterase-induced beta-amyloid aggregation: a dual function lead for Alzheimer’s disease therapy. J Med Chem 2003; 46:2279–2282.
- Pietsch M, Christian L, Inhester T, Petzold S, Gütschow M. Kinetics of inhibition of acetylcholinesterase in the presence of acetonitrile. FEBS J 2009; 276:2292–2307.
- Ram VJ, Pandey HK, Vlietinck AJ. Thieno[2,3-d]pyrimidines as potential chemotherapeutic agents. II. J. J Heterocyclic Chem 1981;18:1277–1280.
- Smolanka IV, Khripak SM, Frolova NP. Dobosh AA. Alkylation of 2-thioureido-3-carboxythiophenes. Ukr Khim Zh 1979;45:871–872.
- Leistner S, Gütschow M, Wagner G. The facile synthesis of 2-aminothieno[2,3-d][1,3]thiazin-4-ones, in some cases 5,6-anellated. Synthesis 1987;466–470.
- Leistner S, Wagner G, Gütschow M, Glusa E. Synthesis of 7-benzyl-5,6,7,8-tetrahydro-pyrido[4′,3′:4,5]thieno [2,3-d]pyrimidine-4(3H)-ones with alkylthioester groups in position 2 and examination of their platelet aggregation-inhibiting activity. Pharmazie 1986; 41:54–55.
- Leistner S, Gütschow M, Vieweg H, Wagner G, Strohscheidt T, Grupe R. Various synthetic routes to imidazo[1,2-c]thieno[3,2-e]pyrimidines and pyrimido[1,2-c]thieno[3,2-e]pyrimidines in some cases anellated. Pharmazie 1988; 43:756–760.
- Ghorab MM. New biologically active N-(tetrahydrobenzothieno-pyrimidin-4-yl)-amino acids, thiourethane, sulfonamides and related compounds. Phosphorus Sulfur Silicon Relat Elem 2000;165:221–35.
- Ellman GL, Courtney KD, Andres V, Feather-Stone RM. A new and rapid colorimetric determination of acetylcholinesterase activity. Biochem Pharmacol 1961;7:88–95.
- Huang Y, Dömling A. The Gewald multicomponent reaction. Mol Divers 2010 (DOI: 10.1007/s11030-010-9229-6).
- Leistner S, Gütschow M, Wagner G. A facile synthesis of 2-alkylthio-4-amino-thieno[2,3-d]pyrimidines. Arch Pharm (Weinheim) 1989;322:227–30.
- Segel IH. Enzyme kinetics. Behavior and analysis of rapid equilibrium and steady-state enzyme systems. New York: John Wiley & Sons Inc., 1993:161–226.
- Baici A. The specific velocity plot. A graphical method for determining inhibition parameters for both linear and hyperbolic enzyme inhibitors. Eur J Biochem 1981; 119:9–14.
- Nochi S, Asakawa N, Sato T. Kinetic study on the inhibition of acetylcholinesterase by 1-benzyl-4-[(5,6-dimethoxy-1-indanon)-2-yl]methylpiperidine hydrochloride (E2020). Biol Pharm Bull 1995; 18:1145–1147.