Abstract
In this study, we have reported the kinetic and biochemical characterization of ectonucleotide pyrophosphatase/phosphodiesterase (E-NPP) activity in rat cardiac fractions, one soluble and the other enriched in vesicles derived from sarcoplasmic reticulum. Both fractions demonstrated E-NPP activities, which could be observed by extracellular hydrolysis of p-nitrophenyl-5′-thymidine monophosphate (p-Nph-5′-TMP) and other biochemical characteristics. The KM values for the hydrolysis of p-Nph-5′-TMP in soluble and microsomal fractions were 118.53 ± 27.28 and 91.92 ± 12.49 µM, respectively. The Vmax values calculated were 2.56 ± 0.15 and 113.87 ± 21.09 nmol p-nitrophenol/min/mg of protein in soluble and microsomal fractions, respectively. Among the compounds tested to evaluate the possible activity of other enzymes on p-Nph-5′-TMP hydrolysis, only suramin (0.25 mM) produced a significant inhibition of substrate hydrolysis. Thus, our results strongly suggest the presence of E-NPP enzymes in subcellular fractions of rat heart, which could be involved in nucleotide signalling in the cardiac tissue.
Keywords::
Introduction
ATP and its metabolites are considered powerful signalling molecules of the cardiovascular system that stimulates vasoconstriction and vasodilatation, growth of vascular smooth muscle cells and endothelial cells, angiogenesis, are involved in vascular remodelling, platelet aggregation, regulates coagulation, inflammation and several aspects of cardiac functionCitation1. Purinergic signalling may be still considered of similar importance as the sympathetic and renin–angiotensin–aldosterone systems in cardiovascular regulation and pathophysiologyCitation1.
The release of endogenous nucleotides might occur upon different pathophysiological processes and represents a critical component for initiating a signalling cascade. In the heart, ATP can be released as a co-transmitter, together with catecholamines from sympathetic nerves, but it may also be released from other sources, such as endothelium, platelets, red blood cells and ischaemic myocardiumCitation1,Citation2. Once in the extracellular medium, ATP can elicit positive or negative inotropic and chronotropic effectsCitation2,Citation3. Furthermore, this nucleotide has been demonstrated to modulate Ca2+, Na+, K+ and Cl− currents in cardiomyocytes and to induce acidosis, cell depolarization and arrhythmia in the heartCitation2. Other possible roles for ATP concern aspects such as hypertrophy, preconditioning and apoptosisCitation3,Citation4. Subsequent to the signal transduction, extracellular nucleotides are rapidly inactivated to adenosine, which has modulatory properties and is considered a cardioprotective molecule mainly because of its vasodilatory effectsCitation5.
The effects listed above generally occur by interaction with cell surface receptors, called purinoceptors, that are expressed with some selectivity on different types of cells in the cardiovascular systemCitation4,Citation6,Citation7. However, evidences from permeabilized muscle fibres and cardiac sarcoplasmic reticulum vesicles (called microsomes) have suggested that adenine nucleotides and adenosine could modulate the Ca2+ currents of the sarcoplasmic reticulum activating directly the ionic channel of the organelleCitation8–10.
Extracellular nucleotides levels and, consequently, their local effects can be regulated by a variety of enzymes (called ectonucleotidases) that are located on the cell surface or may also be soluble in the interstitial medium or within body fluidsCitation5,Citation11. Members of several enzyme families are capable of hydrolysing extracellular ATP and other nucleotides and are also distributed in the cardiovascular system. These include the family of E-NTPDases (ectonucleoside triphosphate diphosphohydrolases), along with the E-NPP family (ectonucleotide pyrophosphatase/phosphodiesterase), alkaline phosphatases and ecto-5′-nucleotidaseCitation7,Citation11,Citation12. Previous studies of our group have reported that cells and tissues can co-express distinct ectonucleotidases that share common characteristicsCitation13–16. The relative contribution of the distinct ectonucleotidases species to the modulation of purinergic signalling may depend on differential tissue and cell distribution, regulation of expression, targeting to specific membrane domains, and on substrate availability and substrate preferenceCitation17.
The family of E-NPPs consists of seven structurally related enzymes that are located at the cell surface, either expressed as transmembrane proteins or as secreted enzymesCitation18. E-NPP family members are localized in different cellular compartments, suggesting specific physiological functionsCitation19. Three of the seven members of the E-NPP family, namely NPP1–3, are known to hydrolyse nucleotides and have been detected in almost all tissues. The enzymatic action of NPP1–3 (in)directly results in the termination of nucleotide signalling, the salvage of nucleotides and/or the generation of new messengers like ADP, adenosine or pyrophosphateCitation17. Recently, our group described the biochemical characteristics of this enzyme activity in synaptosomes from left ventricle of rat heart and identified the enzyme expression of the E-NPP family members in the cardiac tissueCitation20. In that work, the co-expression of E-NPPs and E-NTPDases in cardiac synaptosomes was suggested as a multiple system for the control of extracellular nucleotide levels.
Moreover, adenine nucleotides and adenosine regulate a variety of intra- and extracellular physiological responses in the cardiovascular system, and investigations about the enzymes responsible based on its concentrations are very important. Data concerning the possible roles of E-NPPs on purinergic signalling of the heart are limited and are focused on the extracellular actions. As ecto-5′-nucleotidase activity was recently described in subcellular fragments of the heartCitation21, the study of other enzymes responsible for the cascade of nucleotide hydrolysis becomes important.
Thus, in the present study, we have demonstrated E-NPP activity in rat cardiac fractions, one soluble and the other enriched in vesicles derived from sarcoplasmic reticulum (called microsomal fraction), to participate in the adenine nucleotide hydrolysis and to contribute to the understanding about the control of nucleotide signalling in the cardiac tissue.
Materials and methods
Animals
Male Wistar rats weighing 200–280 g were used in this study. All the animals were housed in cages with food and water available ad libitum. They were maintained under a 12-h light/dark cycle at a constant temperature of 23 ± 2°C. Procedures for the care and use of animals were approved by Colégio Brasileiro de Experimentação Animal (COBEA), based on the Guide for the Care and Use of Laboratory Animals (National Research Council).
Isolation of cardiac soluble and microsomal fractions
The rats were killed by decapitation, hearts were carefully removed and the ventricles were isolated. Soluble and microsomal fractions were prepared as described by Floreani et al.,Citation22 with minor modifications. In brief, both left and right cardiac ventricles of two animals were minced and homogenized in 1:23 (w/v) of 0.25 M sucrose–10 mM Tris (pH 7.4), using a tissue homogenizer (Sorvall Omni-Mixer, 17105) for 3 min at setting 4. The homogenate was centrifuged for 30 min at 10,000 g. The pellet (P1) was discarded and the supernatant (S1) was centrifuged for 60 min at 105,000 g. The supernatant (S2) obtained represented the soluble fraction, whereas the pellet (P2), resuspended in the homogenization buffer, represented the microsomal fraction. The protein was measured by the Coomassie Blue method using bovine serum albumin as standardCitation23. Both fractions were prepared fresh daily and kept at 4°C throughout the process.
Assay of E-NPP activity
Unless specified, enzyme activity was determined in the following incubation medium: 50 mM of Tris–HCl, pH 8.9, and 6.0 mM of MgCl2 in a final volume of 200 μL. Soluble and microsomal proteins (90 and 30 μg, respectively) were added to the reaction medium and pre-incubated for 10 min at 37°C. The reaction was started by the addition of p-nitrophenyl-5′-thymidine monophosphate (p-Nph-5′-TMP), an artificial substrate used routinely for the in vitro E-NPP assay, to a final concentration of 0.5 mM. After 40 min of incubation for the soluble fraction and 6 min of incubation for the microsomal fraction, the reactions were stopped by the addition of 200 μL of NaOH of 0.2 N. For all enzyme assays, incubation times and protein concentration were chosen to ensure the linearity of the reactions. The amount of p-nitrophenol released from the substrate hydrolysis was measured at 400 nm using a molar extinction coefficient of 18.8 × 10−3/M/cm. Controls to correct for non-enzymatic substrate hydrolysis were prepared by adding the enzyme preparation after the reaction with NaOH ceased, as described earlier. All samples were performed in triplicate. Enzyme activities were generally expressed as nmol p-nitrophenol released per minute per milligram of protein.
Time course and optimal protein concentration
Time curves for soluble fraction were performed using 90 µg of protein and time ranging from 30 and 70 min. To microsomal fraction, time ranged from 2 and 10 min and 30 µg of protein were used. In order to determinate optimal protein concentrations, we developed curves using the concentration of protein varying from 70 to 110 µg and from 20 to 60 µg for soluble and microsomal fractions, respectively. In this case, the incubation times were fixed at 40 min for soluble and 6 min for microsomal fraction. Both curves were done incubating the fractions in a reaction medium containing 50 mM of Tris–HCl, pH 8.9 and 6.0 mM of MgCl2.
Divalent cation dependence and pH dependence
To investigate the cation dependence for E-NPP activities, we tested the hydrolysis of p-Nph-5′-TMP in the presence or absence of divalent cations (Ca2+ or Mg2+ in the range of 2.0, 4.0, 6.0 and 8.0 mM) or ethylenediaminetetraacetic acid (EDTA) (0.025, 0.05 and 0.1 mM). EDTA or cations were added to reaction mixture containing 50 mM of Tris–HCl, pH 8.9. For control groups, not EDTA but divalent cations were added to the medium.
The pH curves were performed in a reaction medium containing a mixture of 50 mM of Tris and 50 mM of glycine buffer plus 6.0 mM of MgCl2 (pH varying from 8.0 to 10.0).
Soluble and microsomal protein concentrations and incubation times were the same described in the “Assay of E-NPP activity” section.
Determining effects of inhibitors on p-Nph-5′-TMP hydrolysis
The effects of the following compounds on p-Nph-5′-TMP hydrolysis from rat cardiac soluble and microsomal fractions were analysed: 0.01 mM of lysophosphatidic acid (LPA), 0.4 mg/mL of heparin, 1.0 mM of levamisole, 0.25 mM of suramin, 10 and 20 mM of sodium azide and 0.3 and 0.5 mM of gadolinium chloride. The incubation times as well as protein and substrate concentrations were used as described earlier. Both fractions were pre-incubated in the presence of each inhibitor for 10 min at 37°C, followed by the addition of the substrate. Results were expressed as a percentage of control enzyme activity.
Statistical analysis
The results are expressed as mean ± SD of at least three experiments. Data were analysed by one-way analysis of variance (ANOVA) followed by Tukey-HSD test using the program Graph Pad Prism 5. Values of P < 0.05 were considered significant. The Graph Pad Prism 5 was also used for kinetic analysis of linear regression.
Results
Determining time course and optimal protein concentration
We first investigated the p-Nph-5′-TMP hydrolysis in rat cardiac soluble and microsomal fractions as a function of time and protein concentration to determine the best assay conditions. The fractions were incubated as described in the “Materials and methods” section. The results indicated that the time courses of p-Nph-5′-TMP hydrolysis for the soluble and microsomal fractions were linear up to 70 and 10 min, respectively ( and ). To ensure that the incubation time was within the linearity of the reaction, we chose 40 min as the assay time for the soluble fraction and 6 min for the microsomal fraction in the subsequent experiments.
Figure 1. Time course and protein concentration curves for p-Nph-5′-TMP hydrolysis. Time course in the soluble fraction was performed with 90 µg of protein; 30 µg of protein was used in microsomal fraction. In relation to protein concentration curves were used 40 and 6 min for p-Nph-5′-TMP hydrolysis in soluble and microsomal fractions, respectively. The plots are representative of three independent experiments for each fraction.
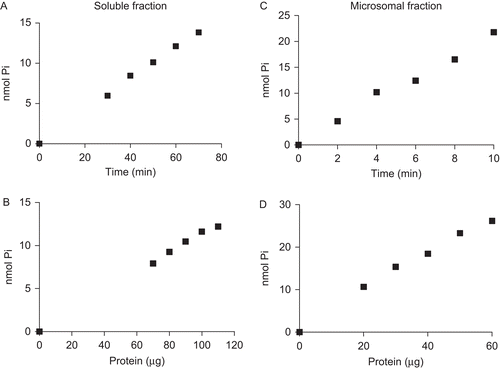
With regard to protein concentration, the results demonstrated that p-Nph-5′-TMP hydrolysis was linear up to 110 and 70 μg for the soluble and microsomal fractions, respectively ( and ). Thus, in the subsequent experiments, we used 90- and 30-μg protein of soluble and microsomal fractions, respectively.
Cation dependence
To investigate the possibility of cation dependence for the rat cardiac soluble and microsomal fractions, we tested the hydrolysis rate of p-Nph-5′-TMP in the presence or absence of divalent cations or EDTA (cation chelator). An activity of approximately 52% and 55% of the total measurable activity in the presence of 6.0 mM of Mg2+ from soluble and microsomal fractions, respectively, was still seen in the absence of added divalent cations. However, these endogenous activities could be removed almost completely by the addition of increasing concentrations of EDTA (0.025, 0.05 and 0.1 mM). This result indicates the presence of endogenous divalent cations in the soluble and microsomal fractions and the cation dependence for both enzyme activities ( and ). Calcium and magnesium concentrations were tested in the range of 2.0–8.0 mM. In the soluble fraction, all concentrations of Mg2+ tested were able to significantly increase the substrate hydrolysis when compared with the respective control group (1.333 ± 0.068 nmol p-nitrophenol/min/mg of protein); on the other hand, none of the Ca2+ concentration tested had significantly effect on the enzyme activity (). In relation to microsomal fraction, our results demonstrated that both divalent cations were able to significantly increase the enzyme activity () when compared with the respective control group (34.853 ± 6.68 nmol p-nitrophenol/min/mg of protein); however, Mg2+ (6.0 and 8.0 mM) was significantly most effective than Ca2+ to stimulate p-Nph-5′-TMP hydrolysis. In both fractions, 6.0 mM of MgCl2 was the final concentration that induced a higher enzyme activity; therefore, this was the concentration chosen in the subsequent experiments.
Figure 2. Divalent cations dependence on p-Nph-5′-TMP hydrolysis. Hydrolysis of p-Nph-5′-TMP by rat cardiac soluble (A) and microsomal (B) fractions were analysed in the absence of cations (Control), in the presence of 0.025, 0.05 and 0.1 mM EDTA and in the presence of 2.0–8.0 mM Ca2+ or Mg2+. Bars represent means ± SD of three independent experiments. Results are expressed as nmol p-nitrophenol/min/mg of protein. *Significantly different from respective control group (P < 0.05).
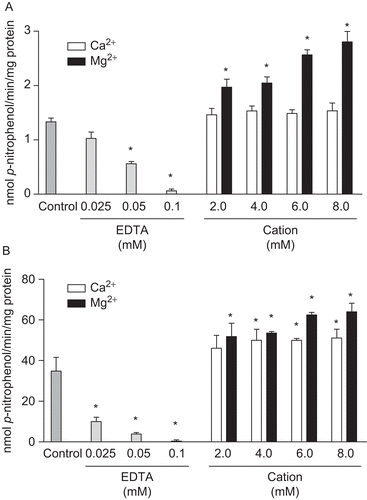
Effect of pH variation
The optimum pH values for p-Nph-5′-TMP hydrolysis were determined in a medium containing a mixture of 50 mM of Tris and 50 mM of glycine buffer (pH varying from 8.0 to 10.0). The pH curves showed the highest activity at a pH of 8.9 and 9.3 for the soluble fraction and microsomal fraction, respectively ( and ). For the subsequent experiments, a pH of 8.9 was chosen for the hydrolysis of p-Nph-5′-TMP in both fractions.
Figure 3. Effect of pH on p-Nph-5′-TMP hydrolysis in rat cardiac soluble (A) and microsomal (B) fractions. Enzyme activity was determined as described in “Materials and methods” using a mixture of the following buffers: Tris and glycine (pH range 8.0 to 10.0). Data represent means ± SD of three different experiments. Results are expressed as nmol p-nitrophenol/min/mg of protein.
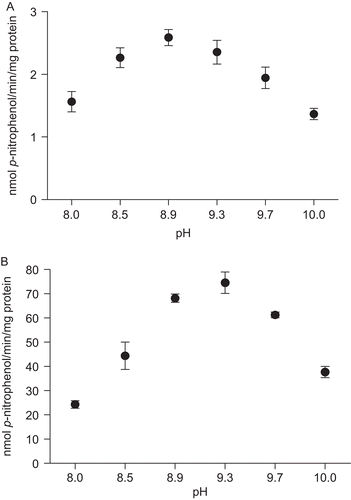
Kinetic parameters
The hydrolysis of p-Nph-5′-TMP was determined at concentrations ranging from 25 to 750 μM. The results ( and , insets) indicated that enzyme activities increased with increasing p-Nph-5′-TMP concentration, with a tendency towards saturation at <750 μM. The Eadie–Hofstee plots for the hydrolysis of p-Nph-5′-TMP on soluble and microsomal fractions are shown in and , respectively. The Michaelis constant (KM) values calculated by linear regression for the hydrolysis of p-Nph-5′-TMP of the soluble and microsomal fractions corresponded to 118.53 ± 27.28 and 91.92 ± 12.49 μM, respectively (mean ± SD, n = 7), and the Vmax values calculated for the soluble and microsomal fractions were 2.56 ± 0.15 and 113.87 ± 21.09 nmol p-nitrophenol/min/mg, respectively (mean ± SD, n = 7).
Figure 4. Eadie–Hofstee plot for p-Nph-5′-TMP hydrolysis. The nucleotide hydrolysis as function of substrate concentration from rat cardiac soluble (A) and microsomal (B) fractions is shown in the insets. The mean KM values calculated for p-Nph-5′-TMP hydrolysis were found to be 118.5 ± 27.2 µM and 91.9 ± 12.4 µM, respectively, and Vmax value calculated in soluble and microsomal fractions were 2.52 ± 0.1 and 113.87 ± 21.1 nmol p-nitrophenol/min/mg, respectively. Data are expressed as mean ± SD, n = 4.
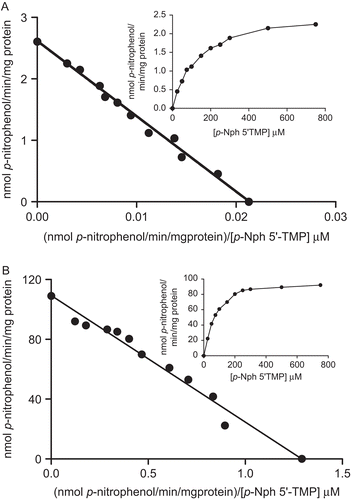
Effect of inhibitors on p-Nph-5′-TMP hydrolysis
To exclude possible enzymatic associations in p-Nph-5′-TMP hydrolysis, we tested some compounds that were reported to affect nucleotide hydrolysis. As shown in , the classical alkaline phosphatase inhibitor, levamisole (1.0 mM)Citation24, the NPP2 inhibitor, LPA (0.01 mM)Citation25 and the NPP1 inhibitor, heparin (0.4 mg/mL)Citation26, were ineffective as inhibitors of p-Nph-5′-TMP hydrolysis in the soluble and microsomal fractions. On the other hand, suramin (0.25 mM), a P2 receptor antagonist and an inhibitor of E-NTPDaseCitation27 and NPP1–2 activitiesCitation28,Citation29, strongly reduced the hydrolysis of p-Nph-5′-TMP in both preparations. However, sodium azide (10 and 20 mM) that is known to inhibit E-NTPDases at high concentration had no effects on substrate hydrolysis. Gadolinium chloride (0.3 and 0.5 mM), a lanthanide that interacts with different pathways of intracellular and extracellular ATP action and that has been considered as the most potent inhibitor for both soluble and membrane-bound E-NTPDasesCitation14,Citation30, had no effects on p-Nph-5′-TMP hydrolysis.
Table 1. Effect of distinct compounds on p-nitrophenyl-5′-TMP hydrolysis from rat cardiac soluble and microsomal fractions.
Discussion
The E-NPP family consists of seven structurally related ectoenzymes (NPP1–NPP7) that possess surprisingly broad substrate specificity, capable of hydrolysing pyrophosphate and phosphodiester bonds in (di)nucleotides, nucleic acids, nucleotide sugars, as well as choline phosphate esters and lysophospholipids. Only the first three members of this family, namely, NPP1 (previously named as plasma cell differentiation antigen-1, PC-1), NPP2 (autotaxin, phosphodiesterase 1α) and NPP3 (gp130 573 RB13-6, B10, phosphodiesterase 1β), are capable of hydrolysing various nucleotides and are therefore relevant in the context of the purinergic signalling cascadeCitation8,Citation19. NPP1–3 has been detected in almost all tissues, although individual isoforms are usually confined to specific substructures and/or cell typesCitation19,Citation31.
In the present study, we showed that rat cardiac soluble and microsomal fractions were able to hydrolyse p-Nph-5′-TMP, with the major biochemical properties already described for E-NPPs. The enzyme activities observed in both fractions demonstrated cation dependence, considering that increasing concentrations of EDTA greatly reduced the catalytic activity. Furthermore, addition of divalent cations, mainly Mg2+, was able to increase p-Nph-5′-TMP hydrolysis. These results are in accordance with the literature that demonstrated NPP1–3 as metalloenzymesCitation31. In addition, with regard to E-NPPs, the pH curves demonstrated a maximal enzymatic activity at alkaline pHCitation20,Citation31. The possible participation of other enzyme activities in the substrate hydrolysis was discarded by the use of several compounds like levamisole, sodium azide and gadolinium chloride, which had no effects. LPA (a potent NPP2 inhibitor) and heparin (NPP1 and NPP2 inhibitor) did not affect significantly the p-Nph-5′-TMP hydrolysis in both fractions; on the other hand, suramin (that also acts as an inhibitor of NPP1 and NPP2 activities) strongly reduced the hydrolysis of p-Nph-5′-TMP in soluble and microsomal fractions. In some cases, suramin have been more potent as an inhibitor of NPP1 and NPP2 than heparinCitation29, which could explain this discrepancy in results. Considering the lack of effect of LPA as an inhibitor of NPP2, the effects of suramin could suggest the presence of NPP1 on both fractions; however, the involvement of NPP3 on p-Nph-5′-TMP hydrolysis may not discarded once it was not investigated. All together our results suggest that under the conditions tested, we worked with a predominant E-NPP activity both in soluble and microsomal fractionsCitation14,Citation20,Citation28. Michaelis constant (KM) and Vmax calculated from the Eadie–Hofstee plot with p-Nph-5′-TMP as substrate demonstrated values that are in accordance with previous studies related to E-NPPsCitation26,Citation29,Citation32.
E-NPP expression in cardiac tissue has already been demonstrated in some worksCitation31,Citation33. However, subcellular distribution and the exact participation of this enzyme activity on nucleotide hydrolysis are poorly understood. In a recent study, Rücker et al.Citation20 investigated the expression of NPP1–3 in rat heart left ventricles, and observed only the NPP2 and NPP3 expression in that tissue; however, NPP1 expression have also been demonstrated in the heart by other researchersCitation31. In addition to demonstrating expression, Rücker et al.Citation20 characterized the biochemical properties of NPP activity in cardiac synaptosomes and suggested the possible involvement of these enzymes in the control of nucleotides and nucleoside levels in the sympathetic nerve ends. In our study, NPP activities identified in soluble and microsomal fractions from heart provide information about a new important way to control the levels of adenine nucleotides, and consequently, its effects on cardiac functions. Soluble NPPs have been described in serum or plasma from humans and other speciesCitation34,Citation35. Nevertheless, the origin of these enzymes is poorly understood, and is generally attributed to their release after different cellular stimulusCitation36–39. NPP activity presents in soluble fraction of the heart may have been originated from a true soluble cytoplasmic form or released from intracellular membranes during homogenization. The physiological role suggested for these enzymes could represent an important auxiliary effector system for local inactivation of acutely elevated nucleotides, especially at sites of injury and inflammationCitation7,Citation13.
Considering that microsomal fraction has origin from intracellular membranes (sarcoplasmic reticulum), NPP activity described in this fraction could represent another way to control nucleotide levels beyond the well-established one. Ectonucleotidases, hydrolysing adenine nucleotides, has also been observed in the microsomes of various tissuesCitation40–42; however, the exact roles of these enzyme activities are not established. The microsomal fraction is described to be enriched by vesicles derived from sarcoplasmic reticulum, and in the heart, this is the major intracellular organelle that sequesters and releases Ca2+, regulating relaxation and tension development by the myocardiumCitation8,Citation9. Evidence has suggested that abnormal release of Ca2+ from this structure has been associated with certain pathological states, including arrhythmias and heart failureCitation43,Citation44. Furthermore, previous studies with isolated Ca2+ channels or microsomes have demonstrated that adenine nucleotides and adenosine are capable of affecting Ca2+ release from sarcoplasmic reticulum through a direct activation of the channelsCitation8,Citation9. Additional evidence about the influence of purinergic signalling on Ca2+ release from sarcoplasmic reticulum can be found in a recent report in which adenosine was described to modulate Ca2+ release through binding at A2A receptors in the sarcoplasmic reticulumCitation45. Taking into consideration the findings discussed earlier and considering that ecto-5′-nucleotidase activity was also recently described in cardiac microsomesCitation21, we suggested that NPP activity observed in the microsomal fraction could modulate Ca2+ release from sarcoplasmic reticulum by controlling the nucleotide levels, and consequently affecting the cardiac excitation–contraction coupling process.
Conclusions
In conclusion, our data demonstrate that enzyme activities obtained from rat cardiac soluble and microsomal fractions share major biochemical properties already described for E-NPPs. These activities were able to hydrolyse p-Nph-5′-TMP, demonstrating cation dependence and a maximal enzymatic activity at alkaline pH. Besides, suramin, an inhibitor of E-NPP activities, strongly reduced the hydrolysis of p-Nph-5′-TMP in both preparations, and the KM and Vmax with p-Nph-5′-TMP as substrate demonstrated values that are in accordance with previous studies related to E-NPPs. The role of these enzymes in the cardiovascular system remains to be clarified, but co-existence with ecto-5′-nucleotidase in these fractionsCitation21 could be important for the control of the nucleotide/nucleoside ratio that are described to be involved in several physiological and pathological processes in heart.
Acknowledgements
This work was supported by grants and fellowship from Conselho Nacional de Desenvolvimento Científico e Tecnológico (CNPq). The authors are very grateful to Dr. JJ Sarkis for his supervision during this work and for dedicating his professional life to study the purinergic system.
Declaration of interest
The authors report no conflicts of interest. The authors alone are responsible for the content and writing of the article.
References
- Erlinge D, Burnstock G. P2 receptors in cardiovascular regulation and disease. Purinergic Signal 2008;4:1–20.
- Vassort G. Adenosine 5′-triphosphate: a P2-purinergic agonist in the myocardium. Physiol Rev 2001;81:767–806.
- Ralevic V, Burnstock G. Involvement of purinergic signaling in cardiovascular diseases. Drug News Perspect 2003;16:133–140.
- Kunapuli SP, Daniel JL. P2 receptor subtypes in the cardiovascular system. Biochem J 1998;336 (Pt 3):513–523.
- Robson SC, Sévigny J, Zimmermann H. The E-NTPDase family of ectonucleotidases: structure function relationships and pathophysiological significance. Purinergic Signal 2006;2:409–430.
- Ralevic V, Burnstock G. Roles of P2-purinoceptors in the cardiovascular system. Circulation 1991;84:1–14.
- Yegutkin GG. Nucleotide- and nucleoside-converting ectoenzymes: important modulators of purinergic signalling cascade. Biochim Biophys Acta 2008;1783:673–694.
- Meissner G. Adenine nucleotide stimulation of Ca2+-induced Ca2+ release in sarcoplasmic reticulum. J Biol Chem 1984;259:2365–2374.
- Meissner G, Henderson JS. Rapid calcium release from cardiac sarcoplasmic reticulum vesicles is dependent on Ca2+ and is modulated by Mg2+, adenine nucleotide, and calmodulin. J Biol Chem 1987;262:3065–3073.
- Duke AM, Steele DS. Effects of caffeine and adenine nucleotides on Ca2+ release by the sarcoplasmic reticulum in saponin-permeabilized frog skeletal muscle fibres. J Physiol (Lond) 1998;513 (Pt 1):43–53.
- Zimmermann H. Ectonucleotidases: some developments and a note on nomenclature. Drug Dev Res 2001;52:44–56.
- Burnstock G. Pathophysiology and therapeutic potential of purinergic signaling. Pharmacol Rev 2006;58:58–86.
- Oses JP, Cardoso CM, Germano RA, Kirst IB, Rücker B, Fürstenau CR et al. Soluble NTPDase: an additional system of nucleotide hydrolysis in rat blood serum. Life Sci 2004;74:3275–3284.
- Fürstenau CR, Trentin Dda S, Barreto-Chaves ML, Sarkis JJ. Ecto-nucleotide pyrophosphatase/phosphodiesterase as part of a multiple system for nucleotide hydrolysis by platelets from rats: kinetic characterization and biochemical properties. Platelets 2006;17:84–91.
- Henz SL, Fürstenau CR, Chiarelli RA, Sarkis JJ. Kinetic and biochemical characterization of an ecto-nucleotide pyrophosphatase/phosphodiesterase (EC 3.1.4.1) in cells cultured from submandibular salivary glands of rats. Arch Oral Biol 2007;52:916–923.
- Buffon A, Casali EA, Cardoso VV, Zerbini LF, Robson SC, Sarkis JJ et al. Differential expression of nucleotide pyrophosphatase/phosphodiesterases by Walker 256 mammary cancer cells in solid tumors and malignant ascites. Life Sci 2010;86:435–440.
- Stefan C, Jansen S, Bollen M. Modulation of purinergic signaling by NPP-type ectophosphodiesterases. Purinergic Signal 2006;2:361–370.
- Stefan C, Jansen S, Bollen M. NPP-type ectophosphodiesterases: unity in diversity. Trends Biochem Sci 2005;30:542–550.
- Goding JW, Grobben B, Slegers H. Physiological and pathophysiological functions of the ecto-nucleotide pyrophosphatase/phosphodiesterase family. Biochim Biophys Acta 2003;1638:1–19.
- Rücker B, Almeida ME, Libermann TA, Zerbini LF, Wink MR, Sarkis JJ. Biochemical characterization of ecto-nucleotide pyrophosphatase/phosphodiesterase (E-NPP, E.C. 3.1.4.1) from rat heart left ventricle. Mol Cell Biochem 2007;306:247–254.
- Pochmann D, Innocente AM, Cotomacci G, Barreto-Chaves ML, Sarkis JJ. AMP hydrolysis in soluble and microsomal rat cardiac cell fractions: kinetic characterization and molecular identification of 5′-nucleotidase. Biosci Rep 2008;28:267–273.
- Floreani M, Napoli E, Quintieri L, Palatini P. Oral administration of trans-resveratrol to guinea pigs increases cardiac DT-diaphorase and catalase activities, and protects isolated atria from menadione toxicity. Life Sci 2003;72:2741–2750.
- Bradford MM. A rapid and sensitive method for the quantitation of microgram quantities of protein utilizing the principle of protein–dye binding. Anal Biochem 1976;72:248–254.
- Constantopoulos A, Kafasi V, Doulas N, Liakakos D, Matsaniotis N. The effect of levamisole on phosphodiesterase activity. Experientia 1977;33:395–396.
- van Meeteren LA, Ruurs P, Christodoulou E, Goding JW, Takakusa H, Kikuchi K et al. Inhibition of autotaxin by lysophosphatidic acid and sphingosine 1-phosphate. J Biol Chem 2005;280:21155–21161.
- Hosoda N, Hoshino SI, Kanda Y, Katada T. Inhibition of phosphodiesterase/pyrophosphatase activity of PC-1 by its association with glycosaminoglycans. Eur J Biochem 1999;265:763–770.
- Leal DB, Streher CA, Neu TN, Bittencourt FP, Leal CA, da Silva JE et al. Characterization of NTPDase (NTPDase1; ecto-apyrase; ecto-diphosphohydrolase; CD39; EC 3.6.1.5) activity in human lymphocytes. Biochim Biophys Acta 2005;1721:9–15.
- Grobben B, Claes P, Roymans D, Esmans EL, Van Onckelen H, Slegers H. Ecto-nucleotide pyrophosphatase modulates the purinoceptor-mediated signal transduction and is inhibited by purinoceptor antagonists. Br J Pharmacol 2000;130:139–145.
- Vollmayer P, Clair T, Goding JW, Sano K, Servos J, Zimmermann H. Hydrolysis of diadenosine polyphosphates by nucleotide pyrophosphatases/phosphodiesterases. Eur J Biochem 2003;270:2971–2978.
- Escalada A, Navarro P, Ros E, Aleu J, Solsona C, Martín-Satué M. Gadolinium inhibition of ecto-nucleoside triphosphate diphosphohydrolase activity in Torpedo electric organ. Neurochem Res 2004;29:1711–1714.
- Bollen M, Gijsbers R, Ceulemans H, Stalmans W, Stefan C. Nucleotide pyrophosphatases/phosphodiesterases on the move. Crit Rev Biochem Mol Biol 2000;35:393–432.
- Kelly SJ, Dardinger DE, Butler LG. Hydrolysis of phosphonate esters catalyzed by 5′-nucleotide phosphodiesterase. Biochemistry 1975;14:4983–4988.
- Stefan C, Gijsbers R, Stalmans W, Bollen M. Differential regulation of the expression of nucleotide pyrophosphatases/phosphodiesterases in rat liver. Biochim Biophys Acta 1999;1450:45–52.
- Birk AV, Broekman MJ, Gladek EM, Robertson HD, Drosopoulos JH, Marcus AJ et al. Role of extracellular ATP metabolism in regulation of platelet reactivity. J Lab Clin Med 2002;140:166–175.
- Yegutkin GG, Samburski SS, Jalkanen S. Soluble purine-converting enzymes circulate in human blood and regulate extracellular ATP level via counteracting pyrophosphatase and phosphotransfer reactions. Faseb J 2003;17:1328–1330.
- Sorensen RG, Mahler HR. Localization of endogenous ATPases at the nerve terminal. J Bioenerg Biomembr 1982;14:527–547.
- Todorov LD, Mihaylova-Todorova S, Westfall TD, Sneddon P, Kennedy C, Bjur RA et al. Neuronal release of soluble nucleotidases and their role in neurotransmitter inactivation. Nature 1997;387:76–79.
- Yegutkin G, Bodin P, Burnstock G. Effect of shear stress on the release of soluble ecto-enzymes ATPase and 5′-nucleotidase along with endogenous ATP from vascular endothelial cells. Br J Pharmacol 2000;129:921–926.
- Yegutkin GG, Samburski SS, Jalkanen S, Novak I. ATP-consuming and ATP-generating enzymes secreted by pancreas. J Biol Chem 2006;281:29441–29447.
- Miura Y, Hirota K, Arai Y, Yagi K. Purification and partial characterization of adenosine diphosphatase activity in bovine aorta microsomes. Thromb Res 1987;46:685–695.
- Valenzuela MA, López J, Depix M, Mancilla M, Kettlun AM, Catalán L et al. Comparative subcellular distribution of apyrase from animal and plant sources. Characterization of microsomal apyrase. Comp Biochem Physiol, B 1989;93:911–919.
- Alleva KE, Espelt MV, Krumschnabel G, Schwarzbaum PJ. Identification of two distinct E-NTPDases in liver of goldfish (Carassius auratus L.). Comp Biochem Physiol B, Biochem Mol Biol 2002;131:725–731.
- Györke S, Györke I, Lukyanenko V, Terentyev D, Viatchenko-Karpinski S, Wiesner TF. Regulation of sarcoplasmic reticulum calcium release by luminal calcium in cardiac muscle. Front Biosci 2002;7:d1454–d1463.
- Hove-Madsen L, Prat-Vidal C, Llach A, Ciruela F, Casadó V, Lluis C et al. Adenosine A2A receptors are expressed in human atrial myocytes and modulate spontaneous sarcoplasmic reticulum calcium release. Cardiovasc Res 2006;72:292–302.
- Hleihel W, Lafoux A, Ouaini N, Divet A, Huchet-Cadiou C. Adenosine affects the release of Ca2+ from the sarcoplasmic reticulum via A2A receptors in ferret skinned cardiac fibres. Exp Physiol 2006;91:681–691.